Abstract
Ribosomes account for a majority of the cell's RNA and much of its protein and represent a significant investment of cellular resources. The turnover and degradation of ribosomes has been proposed to play a role in homeostasis and during stress conditions. Mechanisms for the turnover of rRNA and ribosomal proteins have not been fully elucidated. We show here that the RNS2 ribonuclease and autophagy participate in RNA turnover in Arabidopsis thaliana under normal growth conditions. An increase in autophagosome formation was seen in an rns2–2 mutant, and this increase was dependent on the core autophagy genes ATG9 and ATG5. Autophagosomes and autophagic bodies in rns2–2 mutants contain RNA and ribosomes, suggesting that autophagy is activated as an attempt to compensate for loss of rRNA degradation. Total RNA accumulates in rns2–2, atg9–4, atg5–1, rns2–2 atg9–4, and rns2–2 atg5–1 mutants, suggesting a parallel role for autophagy and RNS2 in RNA turnover. rRNA accumulates in the vacuole in rns2–2 mutants. Vacuolar accumulation of rRNA was blocked by disrupting autophagy via an rns2–2 atg5–1 double mutant but not by an rns2–2 atg9–4 double mutant, indicating that ATG5 and ATG9 function differently in this process. Our results suggest that autophagy and RNS2 are both involved in homeostatic degradation of rRNA in the vacuole.
Abbreviations
AP | = | autophagosome |
ATG | = | autophagy related |
ConA | = | concanamycin A |
GFP | = | green fluorescent protein |
LD | = | long-day |
MDC | = | monodansylcadaverine |
RNase | = | ribonuclease |
WT | = | wild type. |
Introduction
Ribosomes are essential components of all cells and represent a major sink for cellular resources.Citation1 The turnover of ribosomes is important to maintain cell viability and homeostasis during periods of nutritional stress. It was recognized early that macromolecular turnover and rRNA turnover in particular are activated when eukaryotic cells are subjected to deficiencies in carbon, nitrogen, or phosphate; available evidence pointed to a role for autophagic processes in the delivery of macromolecules to vacuoles in plants or lysosomes in animals and protozoa for degradation and recycling.Citation2–5
Autophagy is a macromolecular degradation pathway that functions in the vacuolar degradation and recycling of cellular components. Macroautophagy is the best-characterized form of autophagy and is well studied in plants.Citation6 Macroautophagy, referred to here as autophagy, involves de novo formation of double-membrane vesicles in the cytoplasm through the action of autophagy-related (ATG) proteins. The initial autophagic compartment, called a phagophore, encapsulates cargo targeted for degradation; the phagophore expands and seals to form an autophagosome, which is trafficked to and fuses with the vacuole, resulting in single-membrane autophagic bodies within the lumen. The autophagic bodies are then degraded and the breakdown products recycled. Autophagy functions at a basal level to maintain homeostasis and can be upregulated during stress to aid plant survival.Citation7,8 Both nonselective and selective autophagy pathways exist in many eukaryotes.Citation8–14
Recently, a selective type of autophagy termed ribophagy was described in yeast cells undergoing nitrogen starvation. Genetic analyses showed that ribophagy specifically targets ribosomes for degradation during nutritional stress in a process that depends on components of the basal, nonselective autophagy machinery, such as the scaffold protein Atg17 and E1-like protein Atg7.Citation15 Mutations affecting activity of Ubp3, a ubiquitin protease, caused interruption of ribophagy but not basal autophagy and resulted in cell death when cells were maintained in prolonged nutrient starvation. This indicates that ribosome turnover through selective ribophagy is essential for survival in stressed yeast cells,Citation15 a conclusion also supported indirectly by transcriptome analyses.Citation16 While ribophagy has not yet been specifically studied in other eukaryotes, mounting evidence suggests that the process could be conserved in plants and animals and that starvation is not required for ribophagy to occur.Citation17–19
In addition to an important role in response to nutrient deficiency, rRNA turnover also seems to be necessary for maintenance of cellular homeostasis during periods of normal growth. In Arabidopsis thaliana mutants lacking RNS2 (At2g39780), a nonspecific endoribonuclease of the RNase T2 family that resides in the endoplasmic reticulum and the vacuole, rRNA has a longer half-life than in wild-type (WT) plants, indicating that this enzyme is necessary for normal rRNA decay.Citation19 Moreover, the rns2–2 mutants accumulated RNA intracellularly and mutant cells accumulated acidic vesicles revealed by monodansylcadaverine (MDC) staining, hypothesized to be autophagosomes, even under nonstarvation conditions. These results led to the hypothesis that RNS2 participates in a ribophagy-like mechanism that targets ribosomes for degradation as a housekeeping function that maintains cellular homeostasis. Disruption of this mechanism would lead to accumulation of RNA and the induction of nonselective autophagy as a compensatory mechanism.Citation19 This mechanism seems to be conserved in eukaryotes. Mutations affecting Danio rerio (zebrafish) RNASET2 also resulted in animals that accumulate rRNA in lysosomes, particularly in neurons, and this accumulation caused white-matter lesions in the fish brain, again indicating that rRNA degradation is necessary for cellular homeostasis in nonstarvation conditions.Citation18
The involvement of Arabidopsis RNS2 and zebrafish RNASET2 in housekeeping rRNA turnover is consistent with the hypothesis that the conservation of the RNase T2 family in all eukaryotes is due to a conserved housekeeping role performed by these enzymes.Citation20 In fact, RNase T2 enzymes have been shown to participate in rRNA degradation in conditions of nutritional stress in addition to the housekeeping role in plants and animals described above. For example, RNase T2 enzymes accumulate in the vacuoles of phosphate-deprived tomato cells in parallel with significant rRNA degradation;Citation2 Rny1, the only RNase T2 enzyme in yeast, is responsible for rRNA and bulk RNA degradation in response to starvation and oxidative stress which results in metabolic changes;Citation5,21,Citation22 and mutations affecting Tetrahymena thermophila RNase T2 enzymes cause changes in the patterns of rRNA degradation observed under starvation, suggesting that these enzymes also participate in a ribophagy-like mechanism as a nutrient stress response.Citation23
While there is some agreement that rRNA degradation can occur in the vacuole or lysosome in plants and animals, the mechanisms of transport of rRNA or ribosomes to these organelles is not well characterized, with only the ribophagy mechanism in yeast being somewhat understood. The analysis of rns2–2 phenotypes led us to hypothesize that autophagy may be part of this process. In this work we establish that the rns2–2 mutant has increased basal autophagy. Higher levels of RNA accumulated in rns2–2 and atg mutants. rRNA accumulated in vacuoles from rns2–2 and rns2–2 atg9 plants, but not rns2–2 atg5 mutants. Both RNS2 and autophagy therefore participate in an RNA turnover mechanism, and this autophagic turnover of rRNA is dependent on ATG5 but not ATG9, suggesting a differential role for these proteins.
Results
ATG8-labeled autophagic bodies accumulate in an rns2-2 mutant
The Arabidopsis thaliana RNS2 null mutant (rns2–2) was previously shown to accumulate MDC-stained structures even under normal nutrient-rich conditions,Citation19 which were hypothesized to be autophagosomes. Since this assumption is central to the hypothesis that ribosomes are recycled through a ribophagy-like mechanism in Arabidopsis, and since MDC can stain other acidic organelles in addition to autophagosomes,Citation24 our first objective was to determine whether the MDC-stained structures observed in rns2–2 are autophagosomes. For this purpose, we used transgenic WT and rns2–2 plants containing green fluorescent protein (GFP)-ATG8e (At2g45170). ATG8 isoforms decorate both the inner and outer surface of a completed autophagosome.Citation6,8,Citation25 Fusion of this protein with GFP allows visualization of autophagosomes present in the cytoplasm and autophagic bodies present in the vacuole; thus GFP-ATG8 has been used extensively as an autophagosome and autophagic body marker.Citation26–28
It was unknown if the potential accumulation of autophagosomes observed in rns2–2 was a result of increased autophagosome formation or decreased autophagic body degradation in the vacuole. To differentiate between these 2 possibilities, vacuolar degradation was inhibited with concanamycin A (ConA). ConA inhibits V-type ATPases and subsequently raises vacuolar pH, which reduces hydrolytic enzyme activity and blocks hydrolase delivery to the vacuole.Citation29,30 Seven-d-old seedlings were incubated in liquid Murashige and Skoog (MS) medium containing either 1 μM ConA or dimethylsulfoxide (DMSO) as a solvent control and incubated for 5 h in the dark on an orbital shaker. Seedling roots were then imaged using confocal microscopy (). WT GFP-ATG8e roots had few GFP-labeled autophagosomes without treatment and little autophagic body accumulation upon treatment with ConA. rns2–2,GFP-ATG8e also had a low number of autophagosomes visible, likely because GFP-ATG8e-labeled autophagosomes are difficult to detect in the cytoplasm due to the presence of free cytosolic GFP-ATG8e. However, upon treatment of rns2–2,GFP-ATG8e plants with ConA, numerous GFP-labeled autophagic bodies accumulated inside the vacuole, indicating that autophagosomes are produced constitutively in this mutant. A similar phenotype was also seen in WT and rns2–2 Arabidopsis leaf protoplasts transiently expressing GFP-ATG8e (Fig. S1). This demonstrates that the MDC-stained structures previously reported to accumulate in rns2 mutants are ATG8-tagged autophagosomes, and that their accumulation results from increased autophagosome formation and not decreased autophagic body degradation.
Figure 1. GFP-ATG8e-containing autophagic bodies accumulate in rns2–2 seedling root cells. Seven-d-old transgenic Arabidopsis WT and rns2–2 seedlings expressing the GFP-ATG8e autophagosome marker were treated for 5 h with either DMSO as a solvent control or 1 μM ConA to block vacuolar degradation. Roots were visualized by confocal microscopy. Images are representative of 3 biological replicates. Scale bar: 10 μm.
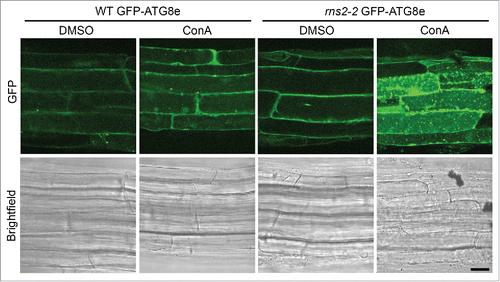
Constitutive autophagy in rns2–2 is complemented by expression of RNS2
To confirm that the constitutive autophagy present in rns2–2 results from loss of RNS2 activity, we introduced the RNS2 cDNA driven by a cauliflower mosaic virus 35S constitutive promoter into the rns2–2 mutant and assessed autophagy by staining with MDC. We first confirmed that GFP-ATG8e colocalizes with MDC-stained autophagosomes in roots as it does in leaf protoplasts.Citation26 It has recently been shown that GFP-ATG8e labeled autophagosomes do not colocalize with MDC-stained structures in Arabidopsis root tips.Citation31 We typically avoid imaging the extreme root tip due to higher background fluorescence. In the region of the root in which we observe autophagosomes, between the elongation zone and ~1 cm from the root tip, the majority of MDC- and GFP-ATG8e-labeled puncta colocalized in the cytoplasm (Fig. S2).
Seven-d-old transgenic seedling roots from 2 independent transformants were stained with MDC to detect autophagosomes and visualized by fluorescence microscopy. Expression of RNS2 in rns2–2 plants led to a decrease in autophagy for both transgenic events tested (). RNS2 activity was verified in the transgenic lines by an in-gel ribonuclease activity assay using high-molecular weight torula yeast RNA as substrate.Citation19,32 Even though RNS2 event #1 had much lower RNS2 activity than WT, both lines showed complementation of the constitutive autophagy phenotype, indicating that relatively low RNS2 activity is sufficient for complementation ().
Figure 2. Genetic complementation of rns2–2 mutant with wild-type RNS2 eliminates the increased autophagy phenotype. (A) Seven-d-old WT, rns2–2, 35S-RNS2 rns2–2 event 1 (RNS2 E1), and 35S-RNS2 rns2–2 event 2 (RNS2 E2) Arabidopsis seedling roots were stained with 50 μM monodansylcadaverine (MDC) and imaged using fluorescence microscopy. Scale bar: 20 μm. (B) Quantification of autophagy. MDC-stained structures as shown in part (A) were quantified from fluorescence microscopy images. Autophagosomes (APs) were counted per frame from 30 root images taken in the late elongation zone per genotype across 3 biological replicates. Error bars represent standard error. Similar letters indicate no significant difference according to the pairwise Student 2-sided equal variance t test (P > 0.05). (C) Activity gel of RNS2 activity. Total protein extracts from 7-d-old seedlings were analyzed using an RNase activity in-gel assay. The position of RNS2 is indicated.
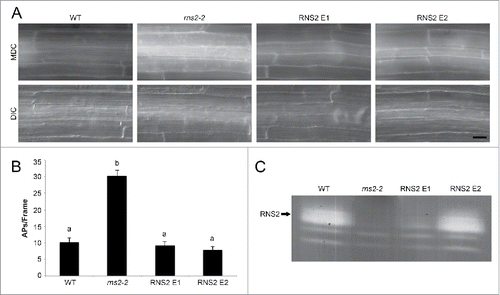
Blocking autophagy in rns2–2 results in rosette growth defects but not stress tolerance defects
We hypothesized that autophagy increases to compensate for lost RNS2 “housekeeping” activity in the rns2–2 mutant. Thus, blocking autophagy in rns2–2 could lead to more severe phenotypes. To test this hypothesis, we crossed rns2–2 with the autophagy mutant atg9 to generate an rns2-2atg9 double mutant. ATG9 (At2g31260) is probably involved in trafficking membrane lipid to newly forming autophagosomes and is required for autophagosome formation.Citation33–35 A previously described atg9 null mutant had early bolting and senescence, as well as reduced seed production and accelerated chlorosis during nitrogen starvation.Citation36 We identified an additional atg9 allele from the SALK T-DNA insertion mutant collection (SALK_145980, CS859902), atg9–4. Characterization of individuals homozygous for the atg9–4 mutation using MDC staining (Fig. S3) showed that, consistent with the previously described atg9 mutant, they are deficient in autophagy activation during salt, nitrogen starvation, and carbon starvation stresses.
rns2–2 was also crossed with an atg5–1 mutant (corresponding to the At5g17290 locus) to generate a rns2–2 atg5–1 double mutant.Citation28 Mutations in ATG5 have been shown to block macroautophagy, selective macroautophagy of protein aggregates, and microautophagy mechanisms in plants and other eukaryotes.Citation28,37–41
rns2–2 has a T-DNA insertion in the fifth intron and is null for RNS2 RNase activity.Citation19 To verify that rns2–2 atg9–4 and rns2–2 atg5–1 plants lack RNS2 activity, total protein extracts from seedlings were subjected to the in-gel ribonuclease activity assay (Fig S4A and S5A). In this zymogram analysis, multiple bands for RNS2 are sometimes detected in WT extracts due to glycosylation of RNS2.Citation19 In contrast, rns2–2, rns2–2 atg9–4, and rns2–2 atg5–1 lacked activity bands in the RNS2 range. RT-PCR demonstrated that expression of ATG9 or ATG5 is absent in atg9–4, atg5–1, rns2–2 atg9–4, and rns2–2 atg5–1 mutants (Fig. S4B and S5B).
Many Arabidopsis autophagy mutants have smaller rosettes,Citation42 while no detailed analysis of changes in growth has been performed for the rns2–2 mutant. To determine whether growth differences exist between the different genotypes analyzed in our work, 26-d-old rosettes were analyzed by Rosette Tracker software.Citation43 Rosette area was determined for rosettes of each genotype and normalized to WT (). It is evident from this analysis that the rns2–2, atg9–4, and atg5–1 mutations have an effect on plant growth. The rns2–2 mutant showed a small but significant increase in total rosette area with respect to WT plants. atg9–4 and atg5–1 were both smaller than WT as expected from observations of other atg mutants. rns2–2 atg9–4 and rns2–2 atg5–1 had smaller rosette areas than atg9–4 and atg5–1 single mutants, indicating that loss of RNS2 enhances the atg mutant growth phenotype. The rns2–2 atg5–1 double mutant showed the most severe phenotype, potentially because of a more complete autophagy block than in atg9–4 mutants.Citation44
Figure 3. Effect of the rns2–2 and atg mutations on rosette size and carbon-starvation tolerance. (A) Twenty-six-d-old Arabidopsis rosettes from WT, rns2–2, atg9–4, atg5–1, rns2–2 atg9–4, and rns2–2 atg5–1 plants grown under LD conditions. Scale bar: 2 cm. (B) WT, rns2–2, atg9–4, and rns2–2 atg9–4 seedlings were grown on MS medium lacking sucrose for 14 d in LD, placed in darkness for 10 d, and recovered in LD for 12 d. (C) Boxplot of normalized rosette area (A) using Rosette Tracker software as a FIJI plugin. 54 to 66 plants were measured for each genotype, with 4 biological replicates for atg9–4 mutants and 2 biological replicates for atg5–1 mutants. The shaded box represents the interquartile range, horizontal line represents the median, and open circles represent outliers >1 .5 standard deviations away from the mean. (D) Percent survival of seedlings grown under sucrose starvation conditions (B) was calculated by counting green seedlings compared to the number of germinated seedlings. 60 to 122 seedlings were used for each genotype, with 7 biological replicates. Error bars represent standard error. Similar letters indicate no significant difference according to the pairwise Student 2-sided equal variance t test (P > 0.05).
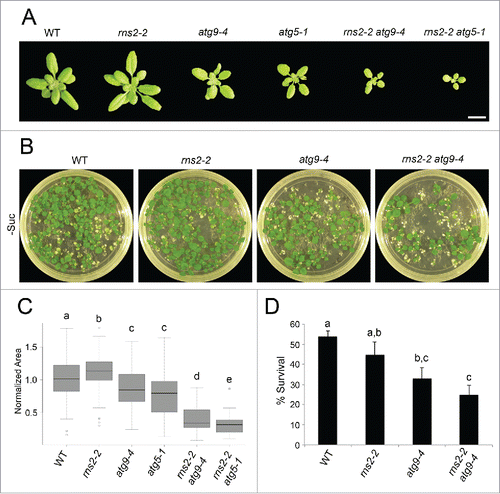
Autophagy mutants, including atg5–1, have increased sensitivity to nutrient stress.Citation28,36,Citation45,46 To test if rns2–2, atg9–4, atg5–1, rns2–2 atg9–4, or rns2–2 atg5–1 also showed increased sensitivity to nutrient deficiency, we subjected seedlings to fixed-carbon starvation stress.Citation47 Seedlings were grown under a long-day (LD) photoperiod on solid MS medium lacking sucrose for 2 wk. Seedlings were then placed in darkness for 10 d and allowed to recover for 12 d under LD. The number of surviving seedlings was compared to the number of germinated seedlings that existed prior to dark exposure (). No significant difference existed between WT and rns2–2. However, atg9–4 and rns2–2 atg9–4 showed increased sensitivity to fixed-carbon starvation. No significant differences existed between atg9–4 and rns2–2 atg9–4, suggesting that autophagy is not essential for rns2–2 plant's survival under fixed-carbon starvation and that rns2–2 adopts atg9-like phenotypes in the rns2–2 atg9–4 double mutant. atg5–1 containing mutants were more sensitive to fixed-carbon starvation than atg9–4 mutants (Fig. S6). No seedlings survived in either atg5–1 or rns2–2 atg5–1, suggesting ATG5 is more important than ATG9 for surviving fixed-carbon starvation stress. This is consistent with previous studies using the atg5–1 mutant.Citation28 The difference in percent survival of WT and rns2–2 seedlings between atg9–4- and atg5–1-containing studies is likely due to differences in the seed stocks used in each analysis.
Autophagy is blocked in rns2–2 atg9–4 and rns2–2 atg5–1
Some autophagy-like processes have been described that do not rely on the classical autophagy machinery.Citation48–50 To characterize the dependence of the rns2–2 constitutive autophagy phenotype on known core autophagy proteins, we analyzed autophagy in rns2–2 atg9–4 and rns2–2 atg5–1 double mutants. Seven-d-old seedling roots were stained with MDC to detect autophagosomes and were visualized by confocal microscopy (). Autophagy is present at a low basal level in WT seedlings,Citation51,52 as indicated by a few fluorescent puncta present in root cells. rns2–2 has increased autophagy, with multiple puncta.Citation19 As determined above, atg9–4 and atg5–1 are deficient in autophagy, and no puncta were observed. Introduction of the atg9–4 or atg5–1 mutant alleles eliminated the increased autophagy phenotype in rns2–2. Quantification of the puncta observed with MDC staining and fluorescence microscopy showed that autophagosome number is significantly and substantially increased in rns2–2 and significantly reduced in atg9–4, atg5–1, rns2–2 atg9–4, and rns2–2 atg5–1 (). More important, mutations in ATG9 and ATG5 completely eliminate the constitutive autophagy phenotype in the rns2–2 mutant. Differences in autophagosome number are seen in the rns2–2 mutant in different experiments, possibly due to variability in growth conditions, compared to WT seedlings. These results further confirm that the MDC-stained puncta that accumulate in rns2–2 are autophagosomes, and that this accumulation relies on the core ATG machinery.
Figure 4. rns2–2 has constitutive autophagy that is eliminated in the atg9–4 and atg5–1 backgrounds. (A and B) Seven-d-old WT, rns2–2, atg9–4, atg5–1, rns2–2 atg9–4, and rns2–2 atg5–1 Arabidopsis seedling roots were stained with 50 μM MDC and imaged using confocal microscopy. Scale bar: 25 μm. (C and D) MDC-stained structures as shown in part (A and B) were quantified from fluorescence microscopy images. Autophagosomes (APs) were counted per frame from 30 root images taken in the late elongation zone per genotype with 3 or 4 biological replicates. Error bars represent standard error. Similar letters indicate no significant difference according to the pairwise Student 2-sided equal variance t test (P > 0.05).
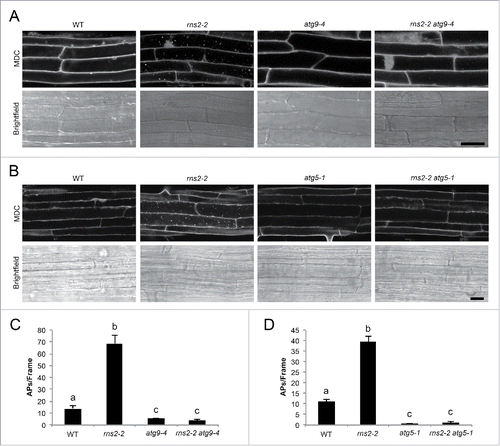
As an alternative approach to test for accumulation of autophagic bodies (and other vesicles) in the vacuole, seedlings were treated with either 1 μM ConA or DMSO as a solvent control for 8 h in liquid MS medium in darkness. Differential interference contrast microscopy was used to visualize vesicle accumulation in the vacuole in seedling root cells. WT, rns2–2, atg9–4, and rns2–2 atg9–4 lines all lacked vesicle accumulation upon treatment with DMSO solvent control (Fig. S7) suggesting vesicle turnover is not affected. However, treatment with ConA resulted in some vesicles accumulating in WT, likely a result of basal autophagy, and a large number of vesicles accumulating in rns2–2. Very few vesicles accumulated in atg9–4 and rns2–2 atg9–4, further indicating that autophagosome trafficking to the vacuole is blocked in the atg9–4 background.
Autophagosomes in rns2–2 contain RNA and ribosomes
We hypothesized that the increased autophagy in rns2–2 may be an attempt to compensate for the loss of RNS2 activity by trafficking ribosomes or rRNA to the vacuole for degradation. To investigate the role of autophagy in trafficking RNA to the vacuole, 7-d-old WT and rns2–2 seedlings were costained with MDC to visualize autophagosomes and SYTO RNASelect to label RNA (). Some cell autofluorescence is detected in unstained controls in the MDC channel, but no puncta are observed in either WT or rns2–2. Significantly more MDC- and SYTO- stained bodies were observed in rns2–2 than in WT seedlings. Colocalization of MDC- and SYTO RNASelect-labeling was also significantly increased in rns2–2, with ~55% of the MDC-labeled structures colocalizing with the SYTO stain (). This suggests that many of the MDC-stained bodies, most likely autophagosomes, contain RNA.
Figure 5. MDC-stained bodies in rns2–2 colocalize with SYTO RNASelect-stained RNA. (A) Seven-d-old WT and rns2–2 Arabidopsis seedlings were costained with 50 μM MDC and 5 μM SYTO RNASelect and analyzed by confocal microscopy. Arrowheads indicate colocalized puncta. Scale bar: 25 μm. (B) Colocalization analysis by line fluorescence tracing of pixel intensity using FIJI software of colocalized puncta in rns2–2. (C) Quantification of MDC, SYTO RNASelect, and colocalized puncta. Percentage of colocalized puncta was calculated by determining the number of colocalized puncta/number of MDC-stained puncta. 5 to 15 images per genotype were analyzed with 3 biological replicates. Error bars represent standard error. Similar letters indicate no significant difference according to the pairwise Student 2-sided equal variance t test (P > 0.05).
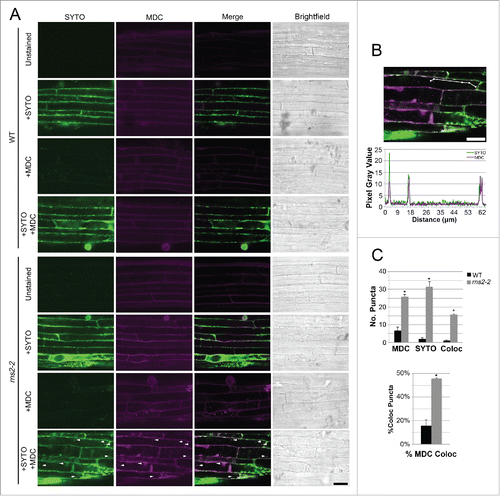
WT and rns2–2 seedling roots were also analyzed by transmission electron microscopy. Autophagosomes contain cytoplasmic components and have a characteristic double-membrane morphology when in the cytoplasm. Upon fusion with the tonoplast, single-membrane autophagic bodies are released into the vacuole lumen. DMSO-treated control seedlings had vacuoles lacking vesicles (). Treatment with ConA resulted in some accumulation of vesicles in the vacuoles of both WT and rns2–2 seedlings. Vesicles in WT were only found occasionally and contained little cytoplasmic cargo. rns2–2 cells accumulated numerous single membrane vesicles within the vacuole that contained components such as mitochondria, endoplasmic reticulum, and ribosomes, indicating that they were autophagic bodies. No double-membrane autophagosomes were observed in the cytoplasm, likely because of their transient nature and rapid trafficking to the vacuole.
Figure 6. rns2–2 accumulates autophagic bodies, containing organelles, inside the vacuole. WT and rns2–2 Arabidopsis seedlings were treated for 8 h in the dark with dimethyl sulfoxide (DMSO) as a solvent control or 1 μM concanamycinA (ConA) to block vacuolar degradation and imaged by transmission electron microscopy. Insets show autophagic bodies within the vacuole (V). rns2–2 inset labels: (R) ribosomes, (M) mitochondron, (ER) endoplasmic reticulum. All scale bars: 2 μm.
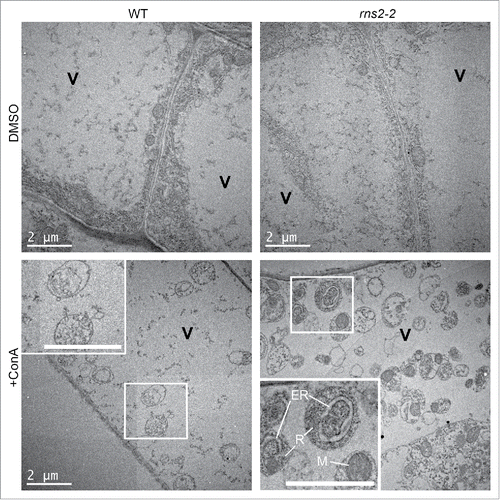
RNA differentially accumulates in mutant lines
Previous results indicated higher levels of SYTO RNASelect staining in Arabidopsis rns2–2 roots and protoplasts.Citation19 Mutations affecting a recently described selective autophagy process (RNautophagy) in mice resulted in increased total cellular RNA levels.Citation53 To examine whether the total level of RNA present in rns2–2, atg9–4, atg5–1, rns2–2 atg9–4, and rns2–2 atg5–1 lines differs from WT, tissue was lyophilized and RNA extracted from each mutant, quantified and normalized to dry weight. rns2–2, atg9–4, atg5–1, rns2–2 atg9–4 and rns2–2 atg5–1 mutants were all found to have significantly higher levels of RNA than WT (). Interestingly, rns2–2 atg5–1 had significantly higher total RNA than the single mutants and the rns2–2 atg9–4 double mutant. This indicates that both RNS2 and the autophagy pathway are required for maintenance of normal cellular RNA levels, with ATG5 having a greater involvement than ATG9 in an rns2–2 background.
Figure 7. Total RNA content is elevated in the rns2–2, atg9–4, and atg5–1 mutants. Total RNA was extracted from lyophilized adult rosette leaves. After quantification, RNA content was normalized to the mass of lyophilized tissue used for the extraction. For each genotype, 5 samples with leaves pooled from 4 plants each were collected in 3 sets of independently grown plants. Error bars represent standard error. Similar letters indicate no significant difference according to the pairwise Student 2-sided equal variance t test (P > 0.05).
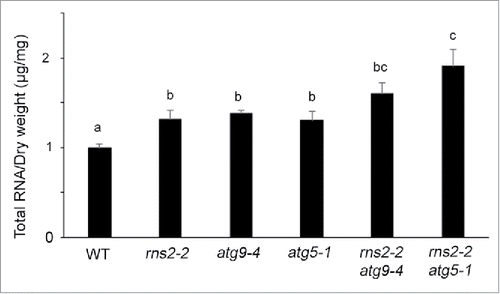
rRNA accumulates in vacuoles of rns2–2 and rns2–2 atg9–4 mutants
As rRNA is the most abundant form of RNA in cells and the half-life of rRNA is increased in rns2–2,19 we hypothesized that rRNA can be transported to the vacuole by autophagy for degradation by RNS2. To test this hypothesis we developed a method for the quantification of RNA within the plant cell vacuole. Vacuoles were purified from WT, rns2–2, atg9–4, atg5–1, rns2–2 atg9–4, and rns2–2 atg5–1 plants and purity assessed by immunoblotting (Fig. S8). Vacuole preps showed high enrichment of the vacuolar protein aleurain, whereas the endoplasmic reticulum protein BiP was undetectable. RNA was extracted from each vacuole sample, cDNA synthesized, and quantitative real-time PCR (qRT-PCR) was used to measure the amount of rRNA in each vacuole preparation. However, no reference gene RNA for qRT-PCR normalization is available within vacuoles. Therefore, to control for vacuole yield and normalize the qRT-PCR results, acid phosphatase activity was measured in aliquots of purified vacuoles (). Acid phosphatase is a vacuolar resident enzyme in plant cellsCitation54,55 and has similar activity in WT, rns2–2, atg9–4, atg5–1, rns2–2 atg9–4, and rns2–2 atg5–1 vacuoles (). Arabidopsis 18S and 25S rRNA-specific primers were used to quantify the amount of rRNA in vacuoles per unit of acid phosphatase activity. rns2–2 vacuoles were found to contain significantly more 18S and 25S rRNA than WT, atg9–4, atg5–1, or rns2–2 atg5–1 (). Interestingly, disrupting autophagy via the rns2–2 atg9–4 mutant did not reduce the amount of rRNA in the vacuole compared with the rns2–2 single mutant, while the rns2–2 atg5–1 double mutant had reduced rRNA accumulation. Furthermore, atg5–1 vacuoles had significantly lower amounts of rRNA than WT or atg9–4. This indicates differing functions of ATG9 and ATG5 in the trafficking and turnover of rRNA in the vacuole by autophagy.
Figure 8. rns2–2 and rns2–2 atg9–4 accumulate rRNA within vacuoles while atg5–1 mutants do not. (A) Vacuolar rRNA quantification protocol. Protoplasts were prepared from adult rosette leaves. Vacuoles were purified from these protoplasts, and checked for quality under the microscope. Each vacuole sample was then split for RNA quantification (including RNA extraction, DNase treatment, cDNA synthesis and qPCR) or acid phosphatase analysis. (B) Acid phosphatase activity was similar across all tested genotypes. Protein was extracted from leaf tissue from the same stage as those used in vacuole purification and assayed for total protein using Bradford analysis or for acid phosphatase activity using 4-methylumbelliferyl phosphate. Total acid phosphatase activity was normalized to total protein revealing no significant differences between genotypes. (C) Quantification of vacuolar rRNA. rRNA from WT and mutant vacuoles was measured using qPCR, and the results were normalized to acid phosphatase activity to account for differences in the amount of vacuoles in each sample. These results are expressed relative to the WT average. WT, rns2–2, atg9–4, and rns2–2 atg9–4 were analyzed in triplicate for 5 biological replicates. WT, rns2–2, atg5–1, and rns2–2 atg5–1 were analyzed in triplicate for 3 biological replicates. Error bars represent standard error. Similar letters indicate no significant difference according to the pairwise Student 2-sided equal variance t test (P > 0.05).
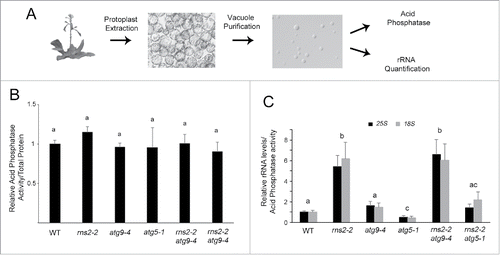
Discussion
The cell closely monitors the synthesis and assembly of ribosomes through a number of processes due to the sizeable anabolic requirements and importance of making stable ribosomes.Citation1,56 Several pathways have been described for the decay of mRNA, misassembled ribosomal subunits, and nonfunctional ribosomes.Citation56,57 However, decay mechanisms by which turnover of normal ribosomes occur are not fully understood. The purpose of this work is to better understand the relationship between autophagy and the turnover of ribosomes in plants. We show that the core autophagy machinery is activated to compensate for lost RNS2 activity in the rns2–2 mutant, and that the autophagosomes contain RNA and ribosomes. The lack of RNS2 activity in this mutant leads to the accumulation of undigested rRNA within the vacuole, and this accumulation is blocked in an rns2–2 atg5–1 double mutant. Moreover, rns2–2, atg9–4, atg5–1, rns2–2 atg9–4, and rns2–2 atg5–1 mutants show elevated amounts of total RNA, suggesting that autophagy is a mechanism of ribosome turnover.
RNase T2 enzymes represent the most widely distributed of all the endoribonuclease families and are conserved in eukaryotes, some prokaryotes, and some viruses.Citation20,58–61 RNS2 is one of 5 RNase T2 enzymes found in Arabidopsis and is the only class II RNase T2 constitutively expressed throughout the plant and throughout development.Citation60,62 RNase T2 proteins are targeted to organelles of the secretory pathway, including the endoplasmic reticulum, Golgi apparatus, vacuole, lysosome, and associated vesicles, or secreted from the cell.Citation20,59,Citation63 RNS2 is localized to the endoplasmic reticulum and vacuole,Citation19 where it functions in degradation of rRNA (and possibly other types of RNA) after uptake into the vacuole either as free RNA or as intact ribosomes. This pathway is thus complementary to cytoplasmic RNA degradation pathways, in which different classes of ribonucleases and degradation machineries function.Citation64,65 Studies in multiple systems have found RNS2 orthologs to be important for the degradation of rRNA. Mutant alleles of RNASET2 are associated with accumulation of undigested rRNA within lysosomes in zebrafish and human neurons,Citation18,66 and rRNA is a cellular substrate for the RNase T2 enzymes of unicellular eukaryotes such as yeast and Tetrahymena during nutrient starvation.Citation22,23
We previously showed that under normal growth conditions rns2–2 and antisense RNS2 plants had rRNA with extended half-life, indicating that rRNA is a substrate for RNS2 in plants. Labeling with the RNA-specific stain SYTO RNASelect suggested that RNA accumulated in the vacuoles of rns2–2 mutants.Citation19 To determine whether this RNA inside the vacuole included rRNA and to measure this accumulation, we developed a method to quantify rRNA within plant cell vacuoles. qRT-PCR is typically normalized to a reference gene such as 18S, ACT2/actin, or Tip41-like;Citation67 however, these transcripts are not present in the vacuole and therefore cannot be used as normalization controls in our system. We also tested for the presence of UBQ10/Ubiquitin and RNS2 but could not detect these transcripts in the vacuole fractions. Instead, acid phosphatase enzyme activity was used to determine the relative amount of purified vacuoles for standardization of qRT-PCR. With this method, we found that 18S and 25S rRNAs accumulate to ~6-fold higher levels in rns2–2 than WT vacuoles. Thus, our results demonstrate that RNS2 participates in the degradation of rRNA that has been delivered to the vacuole either by mechanisms that rely on the core autophagy machinery or by alternative mechanisms (see below).
It was previously shown that rns2–2 plants have increased accumulation of MDC-stained acidic vesicles under normal growth conditions,Citation19 and we show here that these structures are in fact autophagosomes, based on the presence of ATG8 in their membranes and the requirement for ATG9 and ATG5 for their formation. Furthermore, we have shown that autophagosomes and autophagic bodies accumulating in rns2–2 contain RNA and/or ribosomes. These results support the hypothesis that autophagy is being activated to deliver rRNA to the vacuole through a ribophagy-like mechanism to compensate for a reduction in rRNA turnover due to lost RNS2 activity. Quantification of RNA in rosette leaves also indicated that autophagy may function in normal bulk RNA turnover, as rns2–2, atg9–4, atg5–1, rns2–2 atg9–4, and rns2–2 atg5–1 all showed increased levels of total cellular RNA.
However, total RNA accumulation in rns2–2 atg5–1 double mutants was significantly higher than in single mutants, with rns2–2 atg9–4 also showing a marginal increase in total RNA accumulation, suggesting that RNS2 and autophagy do not function entirely in the same pathway for RNA degradation. Reduced accumulation in rns2–2 relative to rns2–2 atg5–1 and rns2–2 atg9–4 may be an effect of the constitutive autophagy phenotype in rns2–2. It is currently unknown if ribosomes can be selectively targeted by autophagy in plants. However, the autophagosomes that form in rns2–2 may also encapsulate stress granules and P-body-like structures in the cytoplasm, which contain RNA degradation machineries including ribonucleases.Citation68,69 Studies in mammals have shown that stress granules are cleared by autophagy.Citation70 If this is the case, the constitutive autophagy may traffic cytoplasmic ribonucleases to the vacuole where they can degrade some of the RNA present there, albeit at a reduced rate since the vacuolar environment is likely below their pH optimum, and the RNases themselves may be targets for degradation by vacuolar proteases.
Interestingly, genetically blocking autophagy using the atg9–4 mutant did not eliminate the accumulation of rRNA in vacuoles, as the rns2–2 atg9–4 mutant contained approximately the same amount of rRNA in its vacuoles as rns2–2. It was recently reported that the atg9 mutant has reduced but not completely blocked autophagic flux.Citation44 Although our microscopy data suggests that atg9–4 blocks the formation of autophagosomes, vacuolar rRNA quantification indicates that rRNA is still delivered to the vacuole in this mutant. atg5–1 is thought to completely lack autophagy, and vacuolar rRNA accumulation is completely lost in rns2–2 atg5–1. These data indicate that either atg9–4 has a low level of autophagy activity remaining that is sufficient to deliver rRNA to the vacuole but is not detectable with our microscopy assays, or that ATG9 and ATG5 function differently in the autophagic trafficking of rRNA to the vacuole.
During macroautophagy, ATG9 is involved in trafficking membrane to the phagophore during autophagosome biosynthesis while ATG5 functions in the conjugation of ATG8 to phosphatidylethanolamine.Citation71 However, other forms of autophagy have been described in eukaryotes. One form, microautophagy, occurs through invagination of the vacuolar membrane to form single membrane vesicles that enter the vacuole for degradation.Citation72 Microautophagy has been reported during plant senescence and development, but little is known about its role in plant cell homeostasis.Citation48,50,Citation73–75 It is possible that ATG5 is involved in a microautophagy-like or other novel mechanism that does not require ATG9 (). Thus, the atg5–1 mutant would block both macro- and microautophagy while atg9–4 would block only macroautophagy. This would also explain the lower vacuolar rRNA accumulation of atg5–1 compared with WT and atg9–4.
Figure 9. Model for the role of RNS2 and autophagy in rRNA turnover. (A) Under normal conditions RNS2 is present in the vacuole and ribosomes are in the cytoplasm with basal autophagy functioning to maintain homeostasis. (B) Mutated rns2–2 results in increased activation of autophagy. This results in accumulation of rRNA within the vacuole. This may occur either by transfer of intact ribosomes as shown, or via transport of rRNA after disassembly of ribosomes (not shown). Some of the autophagosomes may nonspecifically engulf stress granules and P-body-like structures that contain RNases and contribute to some RNA degradation in the vacuole (shown in gray). (C) Disruption of autophagy in atg mutants causes accumulation of RNA in the cytoplasm, likely from ribosomes. Some ribosomes may enter the vacuole through a microautophagy-like mechanism (shown in gray). (D) rns2–2 atg9–4 mutants have increased accumulation of RNA, and much of the rRNA accumulates in the vacuole, possibly by a microautophagy-like mechanism in which ATG9 is not involved. This results in some autophagic flux to the vacuole (shown in gray). (E) rns2–2 atg5–1 blocks both macro- and microautophagy mechanisms resulting in total RNA and rRNA accumulation in the cytoplasm.
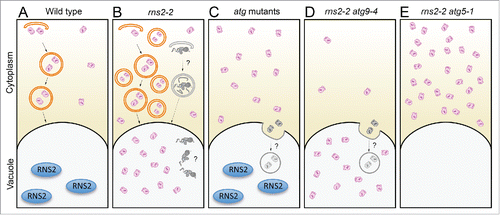
While it is logical to propose that the upregulation of autophagy in rns2–2 is a compensation mechanism for lost RNS2 activity that helps to maintain cellular homeostasis, the actual cellular changes caused by lack of rRNA recycling that lead to increased autophagic activity are unclear. Basal autophagy is always present in plants, turning over cytoplasmic components and functioning as a quality control mechanism.Citation52,76 Autophagy is upregulated during the developmental processes of senescence and cell death as well as during biotic and abiotic stresses such as oxidative stress, drought, nutrient starvation, and pathogen infection.Citation6,36,Citation77–81 Upregulation of autophagy in rns2–2 could be caused by a lack of ribosome turnover that results in the accumulation of toxic products and oxidative stress, or it could be the result of a starvation response, since rRNA represents a large proportion of cellular resources. The turnover of ribosomes through autophagy or other mechanisms may be important for homeostasis and the resources recycled through this process, such as bases, nucleosides, nitrogen, or energy, may be needed to maintain housekeeping functions in normal cells.
In summary, we have shown that autophagy is upregulated in response to a mutation in the RNS2 ribonuclease in Arabidopsis and that the autophagosomes produced contain RNA and/or ribosomes. Disrupting either RNS2 activity or autophagy results in RNA accumulation within the cell, suggesting a role for ATG5-mediated autophagy in RNA turnover. Autophagy, however, may not be the sole process for rRNA turnover in plants. Recent reports suggest RNA and DNA can be transported into lysosomes by a transmembrane transporter in mammalian cells.Citation53,82 LAMP2C, a splice variant of LAMP2, is a lysosomal membrane protein that binds to both DNA and RNA and facilitates transport of DNA and RNA into the lysosomal lumen. Interestingly, LAMP2-deficient mice mutants have constitutive autophagy, suggesting that autophagy may compensate for loss of the LAMP2C transporter.Citation83 Thus, LAMP2C may serve a role in homeostasis and act in a mechanism of normal RNA turnover. Although no ortholog of LAMP2C is present in Arabidopsis, possible alternative transport pathways for RNA are under investigation.
Materials and Methods
Plant growth and Arabidopsis thaliana genotypes
Arabidopsis thaliana Columbia-0 accession was used as wild-type control. For microscopy analyses, seeds were surface sterilized in 33% (v/v) bleach, 1% Triton X-100 (Sigma-Aldrich, T8787) for 20 min followed by cold treatment for ≥2 d. Plants were grown for 7 d under LD conditions (16 h light/8 h dark) at 22°C on nutrient solid Murashige-Skoog medium with vitamins (MS; Caisson Labs, MSP09), 1% sucrose (IBI Scientific, IB37160), 2.4 mM MES, pH 5.7, 0.8% (w/v) phytoagar (Caisson Labs, PTP01) as described.Citation84 Plants used for protoplast, vacuole, and dry weight analyses were grown in soil under LD conditions at 22°C. Sensitivity to fixed carbon starvation was performed as described.Citation45,47 Seedlings were grown in LD conditions on solid MS medium as above without sucrose for 14 d, transferred to darkness for 10 d, and allowed to recover in LD conditions for 12 days, and the percent surviving seedlings counted. Rosette size was analyzed using 26-d-old rosettes grown in LD and Rosette Tracker software as described.Citation43 For salt stress, 7-day-old seedlings were transferred to liquid MS medium +160 mM NaCl for 6 h. For nitrogen and carbon starvation, 7-d-old seedlings were transferred to medium lacking either nitrogen or sucrose for 4 d. Carbon-starvation plates were kept in the dark as described.Citation85,86
T-DNA insertion mutants used in this study were rns2–2 (SALK_069588), atg9–4 (SALK_145980, ABRC stock CS859902), and atg5–1 as described by Thompson et al.Citation28 Crossing of rns2–2 with atg9–4 and atg5–1 was done according to Weigel and Glazebrook.Citation87 Genomic DNA from F1 and F2 progeny was analyzed by PCR using the primers listed in Table S1 to identify rns2–2 atg9–4 and rns2–2 atg5–1 homozygous double-mutant lines. GFP-ATG8e transgenic plants have been previously described.Citation88 Wild-type RNS2 was amplified from cDNA prepared from wild-type leaves. The PCR product was inserted into the MCS11 binary vector containing a 35S promoter using the Clontech InFusion HD recombination based cloning kit (Clontech Laboratories, Inc., 638909). Both rns2–2 GFP-ATG8e and rns2–2 RNS2-expressing transgenic plants were generated by Agrobacterium tumefaciens-mediated transformation using floral dip.Citation89
RT-PCR and ribonuclease activity analysis
RNA was extracted from 7-d-old seedlings using Trizol reagent (Invitrogen, 15596018) and treated with DNaseI (Invitrogen, 18068015). cDNA was synthesized using SuperScript III reverse transcriptase (Invitrogen, 18080093) and oligo dT primer (Promega, C1101). RT-PCR was completed for 28 cycles using the newly synthesized template and primers listed in Table S1. Ribonuclease activity assays were performed as previously described using purified high-molecular weight torula yeast RNA (Sigma, R6625) as substrate.Citation19,32
Transient transformation of rosette leaf protoplasts
Arabidopsis rosette leaf protoplasts were prepared and transformed according to Sheen.Citation90 Thirty micrograms of GFP-ATG8e plasmid DNA was used for each transformation. Transformed protoplasts were treated with 1 μM concanamycin A (Sigma, C9705) or dimethyl sulfoxide (DMSO) as a solvent control for 16 h in darkness rotating on an orbital shaker at 50 rpm and visualized using confocal microscopy.
Staining and microscopy
For differential interference contrast microscopy (DIC), 7-d-old seedlings were treated with 1 μM concanamycin A or DMSO as a solvent control in liquid MS medium for 5 h in the dark at 50 rpm on an orbital shaker. Samples were visualized using a Zeiss Axioplan II light microscope equipped with an Axio Cam HRC digital imaging system and 40 × objective (Carl Zeiss Inc., Jena, Germany). For MDC staining, 7-d-old Arabidopsis seedlings were stained with 50 μM MDC (Sigma, 30432) in phosphate-buffered saline, pH 7.4, according to Contento et al.Citation26 For all seedling microscopy, roots were imaged in the late elongation zone and neighboring cells in the differentiation zone. Root tips and older differentiation region cells were excluded. MDC fluorescence was visualized using a UV lamp and 4’,6-diamidino-2-phenylindole-specific filter (DAPI) with excitation of 360±20 nm and emission of 460±25 nm (Chroma Technology Corp.). The number of motile MDC-stained autophagosomes in all cells visible in the focal plane was quantified and expressed per frame.
Confocal microscopy was performed using a Leica (Leica Microsystems, Wetzlar, Germany) SP5 X MP confocal multiphoton microscope and HPX PL APO CS 63.0x1.40 oil objective. Confocal microscopy of MDC staining used an excitation/emission of 405 nm/430 to 550 nm. For confocal microscopy of seedlings expressing GFP-ATG8e, 7-d-old seedlings were treated with 1 μM concanamycin A or DMSO as a solvent control in liquid MS medium for 5 h in the dark at 50 rpm on an orbital shaker, and visualized using an excitation of 484 nm and emission of 500 to 600 nm.
For costaining using SYTO RNASelect (Invitrogen, S32703) and MDC, 7-day-old seedlings were stained with 5 μM SYTO RNASelect in liquid MS medium for 3 h in darkness on an orbital shaker followed by 3 5-min washes with liquid MS medium. SYTO-stained seedlings were then subjected to MDC staining as above. Fluorescence was visualized using confocal microscopy with an excitation/emission of 405 nm/430 to 480 nm and 490 nm/515 to 540 nm for MDC and SYTO RNASelect respectively with sequential excitation and acquisition to reduce cross-excitation. Fluorescence analysis and quantification was done using FIJI software.Citation91
Transmission electron microscopy
Seven-d-old Arabidopsis seedlings were collected and fixed with 2% glutaraldehyde (w/v) and 1% paraformaldehyde (w/v) in 0.1 M cacodylate buffer, pH 7.2 for 48 h at 4°C. Samples were rinsed 3 times in 0.1 M cacodylate buffer and then post-fixed in 1% osmium tetroxide in 0.1 M cacodylate buffer for 1 h at room temperature. The samples were rinsed in deionized distilled water and enbloc stained with 2% aqueous uranyl acetate for 30 min., dehydrated in a graded ethanol series, cleared with ultrapure acetone, infiltrated and embedded using Spurr epoxy resin (Electron Microscopy Sciences, 14300). Resin blocks were polymerized for 48 h at 65°C. Thick and ultrathin sections were made using a UC6 ultramicrotome (Leica, Wetzlar, Germany) Thick (1 µm) sections were collected onto slides, stained with 1% toluidine blue and imaged using a Zeiss Axioplan II light microscope (Carl Zeiss Inc., Jena, Germany). Ultrathin (60 to 70 nm) sections were collected onto copper grids and counter-stained with 2% uranyl acetate in deionized distilled water for 30 min. Images were captured using a JEOL 2100 scanning and transmission electron microscope (Japan Electron Optic Laboratories, Tokyo, Japan) at the Iowa State University Microscopy and Nanoimaging Facility.
Dry weight total RNA quantification
Arabidopsis rosette leaves were lyophilized for 2 or 3 d, and RNA was extracted from 4–8 mg of dry tissue using TRI Reagent (Invitrogen, AM9738) and resuspended in 100 μL of RNase-free water.Citation53 RNA was quantified using a NanoDrop 1000 Spectrophotometer (Thermo Scientific, Waltham, MA, USA ) and normalized to dry weight.
Vacuolar rRNA quantification
Vacuoles were purified from Arabidopsis rosette leaves grown under LD conditions during early boltingCitation92 and frozen in liquid nitrogen. Vacuole purity was assessed by immunoblotting using antibodies against the endoplasmic reticulum resident protein BiP (Enzo Life Sciences, AD1-SPA-818, 1:1000 dilution) and the vacuolar protein aleurain (1:500 dilution).Citation93 Upon thawing, vacuoles were homogenized by vortexing and 100 μl used for acid phosphatase analysis. RNA was extracted from the remaining vacuoles using an RNeasy Plant Mini Kit (Qiagen, 74904). For this, 900 µl of vacuoles were combined with 600 µl of lysis buffer RLT and RNA extracted according to the manufacturer's protocol. Samples were DNase-treated on the Qiagen RNA column using an on-column DNase (Qiagen, 79254) and RNA eluted with 30 μl of supplied nuclease-free water.
A 13 μl aliquot of vacuole RNA was used for cDNA synthesis using an iScript Select cDNA synthesis kit (Bio-Rad, 170–8896) using random primers with a final cDNA dilution volume of 80 μl. cDNA was tested by semiquantitative PCR using the primers in Table S1. qRT-PCR was carried out using SYBR-Green (Thermo, AB-1158/A) and a Stratagene Mx4000 multiplex quantitative PCR system (Agilent Technologies, Santa Clara, CA, USA) with primers listed in Table S1, with TIP41-like used as an internal control in protoplasts.Citation68 The efficiency for each primer pair and the relative amount of RNA in each sample were calculated using a standard curve as described.Citation94 qPCR results were normalized to the vacuole marker enzyme acid phosphatase activity, which was measured as previously described.Citation54,95 Three to 5 biological replicates per genotype were carried out.
Disclosure of Potential Conflicts of Interest
No potential conflicts of interest were disclosed.
Supplemental_Material.zip
Download Zip (32.9 MB)Acknowledgements
We thank Dr. Richard Vierstra for the atg5–1 mutant, Dr. Natasha Raikhel for the antibodies against aleurain, Tracey Pepper and Randy den Adel for preparing electron microscopy samples, Melanie Torrie and Hayden Stuppnig for help with phenotyping mutants, and Siau Ting Lau for plant maintenance.
Supplemental Material
Supplemental data for this article can be accessed on the publisher's website.
Funding
This work was supported by grant no. MCB-1051818 from the United States National Science Foundation to G.C.M. and D.C.B.
References
- Warner JR. The economics of ribosome biosynthesis in yeast. Trends Biochem Sci 1999; 24:437-40; http://dx.doi.org/10.1016/S0968-0004(99)01460-7
- Loffler A, Abel S, Jost W, Beintema JJ, Glund K. Phosphate-regulated induction of intracellular ribonucleases in cultured tomato (Lycopersicon esculentum) cells. Plant Physiol 1992; 98:1472-8; PMID:16668816; http://dx.doi.org/10.1104/pp.98.4.1472
- Klemperer HG, Pilley DJ. The breakdown of tetrahymena ribosomes by lysosomal-enzymes - inhibition by cytosol. Int J Biochem 1985; 17:399-404; http://dx.doi.org/10.1016/0020-711X(85)90217-4
- Lardeux BR, Mortimore GE. Amino acid and hormonal control of macromolecular turnover in perfused rat liver. Evidence for selective autophagy. J Biol Chem 1987; 262:14514-9
- Huang H, Kawamata T, Horie T, Tsugawa H, Nakayama Y, Ohsumi Y, Fukusaki E. Bulk RNA degradation by nitrogen starvation-induced autophagy in yeast. EMBO J 2015; 34:154-68; PMID:25468960; http://dx.doi.org/10.15252/embj.201489083
- Liu Y, Bassham DC. Autophagy: pathways for self-eating in plant cells. Annu Rev Plant Biol 2012; 63:215-37; PMID:22242963; http://dx.doi.org/10.1146/annurev-arplant-042811-105441
- Thompson AR, Vierstra RD. Autophagic recycling: lessons from yeast help define the process in plants. Curr Opin Plant Biol 2005; 8:165-73; PMID:15752997; http://dx.doi.org/10.1016/j.pbi.2005.01.013
- Li F, Vierstra RD. Autophagy: a multifaceted intracellular system for bulk and selective recycling. Trends Plant Sci 2012; 17:526-37; http://dx.doi.org/10.1016/j.tplants.2012.05.006
- Floyd BE, Morriss SC, Macintosh GC, Bassham DC. What to eat: Evidence for selective autophagy in plants. J Integr Plant Biol 2012; 54:907-20; PMID:23046163
- Grasso D, Ropolo A, Lo Re A, Boggio V, Molejon MI, Iovanna JL, Gonzalez CD, Urrutia R, Vaccaro MI. Zymophagy, a novel selective autophagy pathway mediated by VMP1-USP9x-p62, prevents pancreatic cell death. J Biol Chem 2011; 286:8308-24; http://dx.doi.org/10.1074/jbc.M110.197301
- Johansen T, Lamark T. Selective autophagy mediated by autophagic adapter proteins. Autophagy 2011; 7:279-96; PMID:21189453; http://dx.doi.org/10.4161/auto.7.3.14487
- Kageyama T, Suzuki K, Ohsumi Y. Lap3 is a selective target of autophagy in yeast, Saccharomyces cerevisiae. Biochem Biophys Res Commun 2009; 378:551-7; PMID:19061865; http://dx.doi.org/10.1016/j.bbrc.2008.11.084
- Kim I, Rodriguez-Enriquez S, Lemasters JJ. Selective degradation of mitochondria by mitophagy. Arch Biochem Biophys 2007; 462:245-53; PMID:17475204; http://dx.doi.org/10.1016/j.abb.2007.03.034
- Kraft C, Peter M, Hofmann K. Selective autophagy: ubiquitin-mediated recognition and beyond. Nat Cell Biol 2010; 12:836-41; http://dx.doi.org/10.1038/ncb0910-836
- Kraft C, Deplazes A, Sohrmann M, Peter M. Mature ribosomes are selectively degraded upon starvation by an autophagy pathway requiring the Ubp3p/Bre5p ubiquitin protease. Nat Cell Biol 2008; 10:602-10; http://dx.doi.org/10.1038/ncb1723
- Davey HM, Cross EJ, Davey CL, Gkargkas K, Delneri D, Hoyle DC, Oliver SG, Kell DB, Griffith GW. Genome-wide analysis of longevity in nutrient-deprived Saccharomyces cerevisiae reveals importance of recycling in maintaining cell viability. Environ Microbiol 2012; 14:1249-60; http://dx.doi.org/10.1111/j.1462-2920.2012.02705.x
- Cebollero E, Reggiori F, Kraft C. Reticulophagy and ribophagy: Regulated degradation of protein production factories. Int J Cell Biol 2012; 2012:182834; PMID:22481944; http://dx.doi.org/10.1155/2012/182834
- Haud N, Kara F, Diekmann S, Henneke M, Willer JR, Hillwig MS, Gregg RG, MacIntosh GC, Gärtner J, Alia A, et al. rnaset2 mutant zebrafish model familial cystic leukoencephalopathy and reveal a role for RNase T2 in degrading ribosomal RNA. Proc Natl Acad Sci USA 2011; 108:1099-103; http://dx.doi.org/10.1073/pnas.1009811107
- Hillwig MS, Contento AL, Meyer A, Ebany D, Bassham DC, Macintosh GC. RNS2, a conserved member of the RNase T2 family, is necessary for ribosomal RNA decay in plants. Proc Natl Acad Sci USA 2011; 108:1093-8; http://dx.doi.org/10.1073/pnas.1009809108
- MacIntosh GC. RNase T2 family: Enzymatic properties, functional diversity, and evolution of ancient ribonucleases. In: AW Nicholson, ed. Berlin Heidelberg: Ribonucleases, Nucleic Acids and Molecular Biology Springer-Verlag; 2011, 2011.
- MacIntosh GC, Bariola PA, Newbigin E, Green PJ. Characterization of Rny1, the Saccharomyces cerevisiae member of the T2 RNase family of RNases: unexpected functions for ancient enzymes Proc Natl Acad Sci USA 2001; 98:1018-23; PMID:11158587; http://dx.doi.org/10.1073/pnas.98.3.1018
- Thompson DM, Parker R. The RNase Rny1p cleaves tRNAs and promotes cell death during oxidative stress in Saccharomyces cerevisiae. J Cell Biol 2009; 185:43-50; http://dx.doi.org/10.1083/jcb.200811119
- Andersen KL, Collins K. Several RNase T2 enzymes function in induced tRNA and rRNA turnover in the ciliate Tetrahymena. Mol Biol Cell 2012; 23:36-44; PMID:22049026; http://dx.doi.org/10.1091/mbc.E11-08-0689
- Mizushima N. Methods for monitoring autophagy. Int J Biochem Cell Biol 2004; 36:2491-502; PMID:15325587; http://dx.doi.org/10.1016/j.biocel.2004.02.005
- Xie Z, Nair U, Klionsky DJ. Atg8 controls phagophore expansion during autophagosome formation. Mol Biol Cell 2008; 19:3290-8; PMID:18508918; http://dx.doi.org/10.1091/mbc.E07-12-1292
- Contento AL, Xiong Y, Bassham DC. Visualization of autophagy in Arabidopsis using the fluorescent dye monodansylcadaverine and a GFP-AtATG8e fusion protein. Plant J 2005; 42:598-608; PMID:15860017; http://dx.doi.org/10.1111/j.1365-313X.2005.02396.x
- Yoshimoto K, Hanaoka H, Sato S, Kato T, Tabata S, Noda T, Ohsumi Y. Processing of ATG8s, ubiquitin-like proteins, and their deconjugation by ATG4s are essential for plant autophagy. Plant Cell 2004; 16:2967-83; PMID:15494556; http://dx.doi.org/10.1105/tpc.104.025395
- Thompson AR, Doelling JH, Suttangkakul A, Vierstra RD. Autophagic nutrient recycling in Arabidopsis directed by the ATG8 and ATG12 conjugation pathways. Plant Physiol 2005; 138:2097-110; PMID:16040659; http://dx.doi.org/10.1104/pp.105.060673
- Drose S, Bindseil KU, Bowman EJ, Siebers A, Zeeck A, Altendorf K. Inhibitory effect of modified bafilomycins and concanamycins on P- and V-type adenosinetriphosphatases. Biochemistry 1993; 32:3902-6; PMID:8385991; http://dx.doi.org/10.1021/bi00066a008
- Dettmer J, Hong-Hermesdorf A, Stierhof YD, Schumacher K. Vacuolar H+-ATPase activity is required for Endocytic and secretory trafficking in Arabidopsis. Plant Cell 2006; 18:715-30; PMID:16461582; http://dx.doi.org/10.1105/tpc.105.037978
- Merkulova EA, Guiboileau A, Naya L, Masclaux-Daubresse C, Yoshimoto K. Assessment and optimization of autophagy monitoring methods in arabidopsis roots indicate direct fusion of autophagosomes with vacuoles. Plant Cell Physiol 2014; 55:715-26; PMID:24566535; http://dx.doi.org/10.1093/pcp/pcu041
- Yen Y, Green PJ. Identification and properties of the Major Ribonucleases of Arabidopsis thaliana. Plant Physiol 1991; 97:1487-93; PMID:16668575; http://dx.doi.org/10.1104/pp.97.4.1487
- Noda T, Kim J, Huang WP, Baba M, Tokunaga C, Ohsumi Y, Klionsky DJ. Apg9p/Cvt7p is an integral membrane protein required for transport vesicle formation in the Cvt and autophagy pathways. J Cell Biol 2000; 148:465-80; PMID:10662773; http://dx.doi.org/10.1083/jcb.148.3.465
- Yamamoto H, Kakuta S, Watanabe TM, Kitamura A, Sekito T, Kondo-Kakuta C, Ichikawa R, Kinjo M, Ohsumi Y. Atg9 vesicles are an important membrane source during early steps of autophagosome formation. J Cell Biol 2012; 198:219-33; PMID:22826123; http://dx.doi.org/10.1083/jcb.201202061
- Webber JL, Tooze SA. New insights into the function of Atg9. FEBS Letts 2010; 584:1319-26; http://dx.doi.org/10.1016/j.febslet.2010.01.020
- Hanaoka H, Noda T, Shirano Y, Kato T, Hayashi H, Shibata D, Tabata S, Ohsumi Y. Leaf senescence and starvation-induced chlorosis are accelerated by the disruption of an Arabidopsis autophagy gene. Plant Physiol 2002; 129:1181-93; PMID:12114572; http://dx.doi.org/10.1104/pp.011024
- Le Bars R, Marion J, Le Borgne R, Satiat-Jeunemaitre B, Bianchi MW. ATG5 defines a phagophore domain connected to the endoplasmic reticulum during autophagosome formation in plants. Nat Commun 2014; 5:4121; PMID:24947672
- Wang T, Lao U, Edgar BA. TOR-mediated autophagy regulates cell death in Drosophila neurodegenerative disease. J Cell Biol 2009; 186:703-11; PMID:19720874; http://dx.doi.org/10.1083/jcb.200904090
- Vazquez P, Arroba AI, Cecconi F, de la Rosa EJ, Boya P, de Pablo F. Atg5 and Ambra1 differentially modulate neurogenesis in neural stem cells. Autophagy 2012; 8:187-99; PMID:22240590; http://dx.doi.org/10.4161/auto.8.2.18535
- Filimonenko M, Isakson P, Finley KD, Anderson M, Jeong H, Melia TJ, Bartlett BJ, Myers KM, Birkeland HC, Lamark T, et al. The selective macroautophagic degradation of aggregated proteins requires the PI3P-binding protein Alfy. Mol Cell 2010; 38:265-79; PMID:20417604; http://dx.doi.org/10.1016/j.molcel.2010.04.007
- Guiboileau A, Yoshimoto K, Soulay F, Bataille MP, Avice JC, Masclaux-Daubresse C. Autophagy machinery controls nitrogen remobilization at the whole-plant level under both limiting and ample nitrate conditions in Arabidopsis. New Phytol 2012; 194:732-40; PMID:22404536; http://dx.doi.org/10.1111/j.1469-8137.2012.04084.x
- Patel S, Dinesh-Kumar SP. Arabidopsis ATG6 is required to limit the pathogen-associated cell death response. Autophagy 2008; 4:20-7; PMID:17932459; http://dx.doi.org/10.4161/auto.5056
- De Vylder J, Vandenbussche F, Hu Y, Philips W, Van Der Straeten D. Rosette tracker: an open source image analysis tool for automatic quantification of genotype effects. Plant Physiol 2012; 160:1149-59; PMID:22942389; http://dx.doi.org/10.1104/pp.112.202762
- Shin KD, Lee HN, Chung T. A revised assay for monitoring autophagic flux in Arabidopsis thaliana reveals involvement of AUTOPHAGY-RELATED9 in autophagy. Mol Cells 2014; 37:399-405; PMID:24805779; http://dx.doi.org/10.14348/molcells.2014.0042
- Suttangkakul A, Li FQ, Chung T, Vierstra RD. The ATG1/ATG13 Protein Kinase Complex Is Both a Regulator and a Target of Autophagic Recycling in Arabidopsis. Plant Cell 2011; 23:3761-79; PMID:21984698; http://dx.doi.org/10.1105/tpc.111.090993
- Phillips AR, Suttangkakul A, Vierstra RD. The ATG12-conjugating enzyme ATG10 is essential for autophagic vesicle formation in Arabidopsis thaliana. Genetics 2008; 178:1339-53; http://dx.doi.org/10.1534/genetics.107.086199
- Chung T, Phillips AR, Vierstra RD. ATG8 lipidation and ATG8-mediated autophagy in Arabidopsis require ATG12 expressed from the differentially controlled ATG12A AND ATG12B loci. Plant J 2010; 62:483-93; PMID:20136727; http://dx.doi.org/10.1111/j.1365-313X.2010.04166.x
- Toyooka K, Okamoto T, Minamikawa T. Cotyledon cells of Vigna mungo seedlings use at least two distinct autophagic machineries for degradation of starch granules and cellular components. J Cell Biol 2001; 154:973-82; PMID:11524437; http://dx.doi.org/10.1083/jcb.200105096
- Yano K, Hattori M, Moriyasu Y. A novel type of autophagy occurs together with vacuole genesis in miniprotoplasts prepared from tobacco culture cells. Autophagy 2007; 3:215-21; PMID:17224627; http://dx.doi.org/10.4161/auto.3739
- Levanony H, Rubin R, Altschuler Y, Galili G. Evidence for a Novel Route of Wheat Storage Proteins to Vacuoles. J Cell Biol 1992; 119:1117-28; PMID:1447291; http://dx.doi.org/10.1083/jcb.119.5.1117
- Slavikova S, Shy G, Yao YL, Giozman R, Levanony H, Pietrokovski S, Elazar Z, Galili G. The autophagy-associated Atg8 gene family operates both under favourable growth conditions and under starvation stresses in Arabidopsis plants. J Exp Bot 2005; 56:2839-49; PMID:16157655; http://dx.doi.org/10.1093/jxb/eri276
- Inoue Y, Suzuki T, Hattori M, Yoshimoto K, Ohsumi Y, Moriyasu Y. AtATG genes, homologs of yeast autophagy genes, are involved in constitutive autophagy in Arabidopsis root tip cells. Plant Cell Physiol 2006; 47:1641-52; PMID:17085765; http://dx.doi.org/10.1093/pcp/pcl031
- Fujiwara Y, Furuta A, Kikuchi H, Aizawa S, Hatanaka Y, Konya C, Uchida K, Yoshimura A, Tamai Y, Wada K, et al. Discovery of a novel type of autophagy targeting RNA. Autophagy 2013; 9:403-9; PMID:23291500; http://dx.doi.org/10.4161/auto.23002
- Ahmed SU, Rojo E, Kovaleva V, Venkataraman S, Dombrowski JE, Matsuoka K, Raikhel NV. The plant vacuolar sorting receptor AtELP is involved in transport of NH2-terminal propeptide-containing vacuolar proteins in Arabidopsis thaliana. J Cell Biol 2000; 149:1335-44; PMID:10871276; http://dx.doi.org/10.1083/jcb.149.7.1335
- Leigh RA, Walker RR. Atpase and acid-phosphatase activities associated with vacuoles isolated from storage roots of red beet (Beta-Vulgaris L). Planta 1980; 150:222-9; PMID:24306686; http://dx.doi.org/10.1007/BF00390830
- Lafontaine DLJ. A 'garbage can' for ribosomes: how eukaryotes degrade their ribosomes. Trends Biochem Sci 2010; 35:267-77; PMID:20097077; http://dx.doi.org/10.1016/j.tibs.2009.12.006
- Houseley J, Tollervey D. The Many Pathways of RNA Degradation. Cell 2009; 136:763-76; PMID:19239894; http://dx.doi.org/10.1016/j.cell.2009.01.019
- Hillwig MS, Rizhsky L, Wang Y, Umanskaya A, Essner JJ, MacIntosh GC. Zebrafish RNase T2 genes and the evolution of secretory ribonucleases in animals. BMC Evol Biol 2009; 9:170; PMID:19619322; http://dx.doi.org/10.1186/1471-2148-9-170
- Irie M. Structure-function relationships of acid ribonucleases: Lysosomal, vacuolar, and periplasmic enzymes. Pharmacol Therapeut 1999; 81:77-89; http://dx.doi.org/10.1016/S0163-7258(98)00035-7
- MacIntosh GC, Hillwig MS, Meyer A, Flagel L. RNase T2 genes from rice and the evolution of secretory ribonucleases in plants; PMID:20182746; http://dx.doi.org/10.1007/s00438-010-0524-9
- Ambrosio L, Morriss S, Riaz A, Bailey R, Ding J, MacIntosh GC. Phylogenetic analyses and characterization of RNase X25 from Drosophila melanogaster suggest a conserved housekeeping role and additional functions for RNase T2 enzymes in protostomes. PLoS One 2014; 9:e105444; PMID:25133712; http://dx.doi.org/10.1371/journal.pone.0105444
- Igic B, Kohn JR. Evolutionary relationships among self-incompatibility RNases. Proc Natl Acad Sci USA 2001; 98:13167-71; PMID:11698683; http://dx.doi.org/10.1073/pnas.231386798
- Luhtala N, Parker R. T2 Family ribonucleases: ancient enzymes with diverse roles. Trends Biochem Sci 2010; 35:253-9; PMID:20189811; http://dx.doi.org/10.1016/j.tibs.2010.02.002
- Decker CJ, Parker R. P-bodies and stress granules: possible roles in the control of translation and mRNA degradation. Cold Spring Harbor Perspectives in Biology 2012; 4:a012286; PMID:22763747; http://dx.doi.org/10.1101/cshperspect.a012286
- Schoenberg DR, Maquat LE. Regulation of cytoplasmic mRNA decay. Nat Rev Genet 2012; 13:246-59; PMID:22392217; http://dx.doi.org/10.1038/nrg3254
- Henneke M, Diekmann S, Ohlenbusch A, Kaiser J, Engelbrecht V, Kohlschutter A, Kratzner R, Madruga-Garrido M, Mayer M, Opitz L, et al. RNASET2-deficient cystic leukoencephalopathy resembles congenital cytomegalovirus brain infection. Nat Genet 2009; 41:773-5; PMID:19525954; http://dx.doi.org/10.1038/ng.398
- Czechowski T, Stitt M, Altmann T, Udvardi MK, Scheible WR. Genome-wide identification and testing of superior reference genes for transcript normalization in Arabidopsis. Plant Physiol 2005; 139:5-17; PMID:16166256; http://dx.doi.org/10.1104/pp.105.063743
- Xu J, Chua NH. Processing bodies and plant development. Curr Opin Plant Biol 2011; 14:88-93; PMID:21075046; http://dx.doi.org/10.1016/j.pbi.2010.10.003
- Eulalio A, Behm-Ansmant I, Izaurralde E. P bodies: at the crossroads of post-transcriptional pathways. Nat Rev Mol Cell Biol 2007; 8:9-22; PMID:17183357; http://dx.doi.org/10.1038/nrm2080
- Buchan JR, Kolaitis RM, Taylor JP, Parker R. Eukaryotic stress granules are cleared by autophagy and Cdc48/VCP function. Cell 2013; 153:1461-74; PMID:23791177; http://dx.doi.org/10.1016/j.cell.2013.05.037
- Noda NN, Inagaki F. Mechanisms of autophagy. Annu Rev Biophys 2015; 44:101-22; PMID:25747593
- Mijaljica D, Prescott M, Devenish RJ. Microautophagy in mammalian cells: revisiting a 40-year-old conundrum. Autophagy 2011; 7:673-82; PMID:21646866; http://dx.doi.org/10.4161/auto.7.7.14733
- Inada N, Sakai A, Kuroiwa H, Kuroiwa T. Three-dimensional analysis of the senescence program in rice (Oryza sativa L.) coleoptiles. Investigations of tissues and cells by fluorescence microscopy. Planta 1998; 205:153-64; PMID:9637068; http://dx.doi.org/10.1007/s004250050307
- Smith MT, Saks Y, Vanstaden J. Ultrastructural-Changes in the Petals of Senescing Flowers of Dianthus-Caryophyllus L. Ann Bot 1992; 69:277-85
- Thompson JE, Froese CD, Hong Y, Hudak KA, Smith MD. Membrane deterioration during senescence. Can J Bot 1997; 75:867-79; http://dx.doi.org/10.1139/b97-096
- Gupta HS. Vacuolar autophagy - apparent dilution and elimination of cytoplasmic organelles in acridine orange-treated plant-cells. Caryologia 1988; 41:347-59; http://dx.doi.org/10.1080/00087114.1988.10797875
- Chen MH, Liu LF, Chen YR, Wu HK, Yu SM. Expression of alpha-amylases, carbohydrate-metabolism, and autophagy in cultured rice cells is coordinately regulated by sugar nutrient. Plant J 1994; 6:625-36; PMID:8000424; http://dx.doi.org/10.1046/j.1365-313X.1994.6050625.x
- Beers EP. Programmed cell death during plant growth and development. Cell Death Differ 1997; 4:649-61; PMID:16465277; http://dx.doi.org/10.1038/sj.cdd.4400297
- Kwon SI, Cho HJ, Jung JH, Yoshimoto K, Shirasu K, Park OK. The Rab GTPase RabG3b functions in autophagy and contributes to tracheary element differentiation in Arabidopsis. Plant J 2010; 64:151-64; PMID:20659276
- Liu Y, Schiff M, Czymmek K, Talloczy Z, Levine B, Dinesh-Kumar SP. Autophagy regulates programmed cell death during the plant innate immune response. Cell 2005; 121:567-77; PMID:15907470; http://dx.doi.org/10.1016/j.cell.2005.03.007
- Moriyasu Y, Ohsumi Y. Autophagy in tobacco suspension-cultured cells in response to sucrose starvation. Plant Physiol 1996; 111:1233-41; PMID:12226358
- Fujiwara Y, Kikuchi H, Aizawa S, Furuta A, Hatanaka Y, Konya C, Uchida K, Wada K, Kabuta T. Direct uptake and degradation of DNA by lysosomes. Autophagy 2013; 9:1167-71; PMID:23839276; http://dx.doi.org/10.4161/auto.24880
- Tanaka Y, Guhde G, Suter A, Eskelinen EL, Hartmann D, Lullmann-Rauch R, Janssen PML, Blanz J, von Figura K, Saftig P. Accumulation of autophagic vacuoles and cardiomyopathy in LAMP-2-deficient mice. Nature 2000; 406:902-6; PMID:10972293; http://dx.doi.org/10.1038/35022595
- Liu YM, Burgos JS, Deng Y, Srivastava R, Howell SH, Bassham DC. Degradation of the endoplasmic reticulum by autophagy during endoplasmic reticulum stress in arabidopsis. Plant Cell 2012; 24:4635-51; PMID:23175745; http://dx.doi.org/10.1105/tpc.112.101535
- Liu YM, Xiong Y, Bassham DC. Autophagy is required for tolerance of drought and salt stress in plants. Autophagy 2009; 5:954-63; PMID:19587533; http://dx.doi.org/10.4161/auto.5.7.9290
- Doelling JH, Walker JM, Friedman EM, Thompson AR, Vierstra RD. The APG8/12-activating enzyme APG7 is required for proper nutrient recycling and senescence in Arabidopsis thaliana. J Biol Chem 2002; 277:33105-14; PMID:12070171; http://dx.doi.org/10.1074/jbc.M204630200
- Weigel D, Glazebrook J. Arabidopsis: A laboratory manual. Cold spring harbor. New York: Cold Spring Harbor Laboratory Press; 2002.
- Xiong Y, Contento AL, Nguyen PQ, Bassham DC. Degradation of oxidized proteins by autophagy during oxidative stress in Arabidopsis. Plant Physiol 2007; 143:291-9; PMID:17098847; http://dx.doi.org/10.1104/pp.106.092106
- Clough SJ, Bent AF. Floral dip: a simplified method for Agrobacterium-mediated transformation of Arabidopsis thaliana. Plant J 1998; 16:735-43; PMID:10069079; http://dx.doi.org/10.1046/j.1365-313x.1998.00343.x
- Sheen J. A transient expression assay using Arabidopsis meshophyll protoplasts. http://geneticsmghharvardedu/sheenweb/2002.
- Schindelin J, Arganda-Carreras I, Frise E, Kaynig V, Longair M, Pietzsch T, Preibisch S, Rueden C, Saalfeld S, Schmid B, et al. Fiji: an open-source platform for biological-image analysis. Nat Methods 2012; 9:676-82; PMID:22743772; http://dx.doi.org/10.1038/nmeth.2019
- Robert S, Zouhar J, Carter C, Raikhel N. Isolation of intact vacuoles from Arabidopsis rosette leaf-derived protoplasts. Nat Protoc 2007; 2:259-62; PMID:17406583; http://dx.doi.org/10.1038/nprot.2007.26
- Ahmed SU, Rojo E, Kovaleva V, Venkataraman S, Dombrowski JE, Matsuoka K, Raikhel NV. The plant vacuolar sorting receptor AtELP is involved in transport of NH(2)-terminal propeptide-containing vacuolar proteins in Arabidopsis thaliana. J Cell Biol 2000; 149:1335-44; PMID:10871276; http://dx.doi.org/10.1083/jcb.149.7.1335
- Pfaffl MW. Quantification strategies in real-time PCR. A-Z of quantitative PCR. Ed. SA Bustin. La Jolla, CA, USA: International University Line; 2004: 87-112.
- Reilly TJ, Baron GS, Nano FE, Kuhlenschmidt MS. Characterization and sequencing of a respiratory burst-inhibiting acid phosphatase from Francisella tularensis. J Biol Chem 1996; 271:10973-83; PMID:8631917; http://dx.doi.org/10.1074/jbc.271.18.10973