ABSTRACT
The requirement of macroautophagic/autophagic machinery for filamentous fungal development and pathogenicity has been recognized, but the underlying effects and mechanisms remain elusive. The insect pathogenic fungus Metarhizium robertsii infects hosts by cuticular penetration through the formation of the infection structure appressoria. Here, we show that autophagic fluxes were highly activated during the appressorial formation of M. robertsii. Genome-wide deletion of the autophagy-related genes and insect bioassays identified 10 of 23 encoded MrATG genes with requirements for topical fungal infection of insect hosts. Besides the defect in forming appressoria on insects (two null mutants), these virulence-reduced mutants were largely impaired in penetrating cellophane membrane and insect cuticles, suggesting their failures in generating proper appressorium turgor. We found that the conidial storage of lipid droplets (LDs) had no obvious difference between strains, but autophagic LD degradation was impaired in different mutants. After induction of cell autophagy by nitrogen starvation, we found that LD entry into vacuoles was unaffected in the selected mutant cells with potential failures in forming autophagosomes. The finding therefore reveals a microlipophagy machinery employed in this fungus and that the direct engulfment of LDs occurs without inhibition by the downstream defective lipolysis. Our data first unveil the activation and contribution of microlipophagy to fungal infection biology. The obtained technique may benefit future detection of microlipophagy in different organisms by examining vacuolar or lysosomal engulfment of LDs in core autophagic gene deletion mutants.
Abbreviations: AIM: Atg8-family interacting motif; ATG: autophagy-related; CM: complete medium; CMAC: 7-amino-4-chloromethylcoumarin; DTT: dithiothreitol; ER: endoplasmic reticulum; GFP: green fluorescent protein; LD, lipid droplet; MM: minimum medium; MM-N: minimum medium without nitrogen source; PDA: potato dextrose agar; PMSF: phenylmethylsulfonyl fluoride; RFP: red fluorescent protein; SDB: Sabouraud dextrose broth; SDS-PAGE: sodium dodecyl sulfate-polyacrylamide gel electrophoresis; TAG: triacylglycerol; TEM: transmission electron microscopy; WT, wild type.
Introduction
Cell autophagy plays an essential role in maintaining cellular homeostasis and physiology by degrading damaged proteins or organelles in responses to nutrient starvation and or adverse environments [Citation1,Citation2]. Based on the study of the budding yeast and mammalian cells, autophagy processes have been classified into three forms: macroautophagy, microautophagy, and chaperone-mediated autophagy (CMA) in association with the pattern of cargo delivery [Citation3,Citation4]. Of which, macroautophagy (usually referred to as autophagy) has been mostly characterized through the formation of autophagic vesicles (i.e., autophagosomes) to selectively mediate the cargo transfer and degradation in lysosomes or vacuoles [Citation5]. In contrast, microautophagy mediates the direct engulfment of the cytoplasmic cargoes into lysosomes or vacuoles for degradation [Citation6,Citation7], which is still understudied. For example, a few studies have revealed a process resembling microautophagy for lipid-droplet (LD) degradation (i.e., microlipophagy) in yeast cells [Citation8–12]. It has just been found by live-cell imaging that stable contacts could occur between LDs and lysosomes to mediate microlipophagic degradation of LDs in hepatocytes [Citation13]. Otherwise, the microlipophagic effect and machinery of LD degradation have not been reported in other organisms including filamentous fungi.
Yeast-like autophagy-related genes (ATGs) are omnipresent in different eukaryotes that can be grouped into different modules to mediate the autophagic processes such as autophagy initiation, autophagosome formation, and cargo degradation [Citation2,Citation14,Citation15]. Studies have shown that different ATG-like genes are required for the virulence of plant, mammalian and invertebrate pathogenic fungi [Citation2,Citation16–18]. For example, the yeast ATG8-like gene MoATG8 of Magnaporthe oryzae is required for mediating conidium cell death to initiate the rice blast disease [Citation19]. Further individual deletions of 22 ATG-like genes in M. oryzae identified 16 of them that are required for full fungal virulence [Citation20]. The generation of 28 ATG gene-deletion mutants in Fusarium graminearum found that, except for Fgatg17∆, all other FgATG-gene mutants lost their abilities to cause wheat head blight disease [Citation21]. Deletion of multiple ATG genes in the human pathogen Cryptococcus neoformans revealed that 14 genes were required for the development of cryptococcosis in mice or the infection of the wax moth (Galleria mellonella) model [Citation22,Citation23]. Filamentous insect pathogenic fungus such as Metarhizium robertsii and Beauveria bassiana have been developed as alternative insect biocontrol agents and exploited as the models for investigating the mechanisms of fungus-animal interactions [Citation24,Citation25]. Deletion of a few ATG genes in either M. robertsii or B. bassiana also led to the varying levels of virulence reduction in these fungi against insect hosts [Citation18,Citation26]. Other than the findings of the ATG-gene involvements in fungal sporulation, appressorium differentiation and or stress responses [Citation27], the contribution of autophagy components to fungal development and virulence largely remains elusive.
In this study, we found that cellular autophagic activities were highly activated to mediate the degradation of LDs during the appressorium formation and maturation in M. robertsii. Genome-wide deletion of the yeast-like ATG genes followed by insect bioassays identified 10 out of 23 obtained mutants with reduced virulence during topical infection of insect hosts. Null mutants were largely impaired in penetrating insect cuticles associated with the defect of autophagic LD degradation instead of LD engulfment. We provide evidence to support a microlipophagy machinery employed in this fungus for mediating LD lipolysis to ensure fungal success in infecting insect hosts.
Results
Activation of cell autophagic fluxes during appressorium formation
We first transformed the wild type (WT) strain of M. robertsii with a fused RFP-MrATG8 cassette under the control of the MrATG8 gene promoter. It was found that, relative to the growth in a nutrient-rich medium, nitrogen starvation could trigger the accumulation of the RFP-MrAtg8 signals in vacuoles, i.e., the characteristic induction of autophagic activities in M. robertsii (). Similar to the effect of nitrogen deprivation, we found that the elevated RFP-MrAtg8 signals were detected in appressoria induced on the hydrophobic surfaces when the fused protein was controlled by the promoter of either the MrATG8 gene or a constitutive HSP70 gene (). The vacuolar distribution of the RFP-MrAtg8 signals was in contrast to the cytosolic expression of RFP (). Appressoria were also induced on the front wings of the mealworm beetles. The transmission electron microscopy (TEM) analysis confirmed the formation of autophagic bodies within the vacuoles of appressorial cells (). Thus, activation of the autophagic fluxes occurred during the appressorium formation of M. robertsii.
Figure 1. Autophagic activation during nitrogen starvation and appressorial formation. (A) Accumulation of the RFP-MrAtg8 signal in vacuoles upon nitrogen starvation. The fused gene was controlled by the MrATG8 gene promoter. Relative to the growth in the nutrient-rich SDB medium for 16 h (upper panels), vacuole formation (stained with the CMAC dye) and localization of RFP-MrAtg8 signals were induced in an MM-N medium for 4 h. Bar: 5 μm. (B) TEM analysis showing the formation of autophagic bodies (arrowed) upon nitrogen starvation. Relative to the growth in the nutrient-rich SDB medium (upper panel), autophagic bodies were formed in vacuole when the fungus was grown in an MM-N medium. LD, lipid droplet. Bar: 0.5 μm. (C) Accumulation of the RFP-MrAtg8 signal in appressorium vacuoles. After induction on a hydrophobic surface for 20 h, expression of RFP-MrAtg8 was controlled by either the MrATG8 promoter (Self-Pro) or a constitutive HSP70 promoter (pHSP70) of M. robertsii. Vacuoles were stained with the specific dye CMAC. Bar: 5 μm. (D) RFP expression in appressorium controlled by the HSP70 prpmoter. Inset shows the bright field image. Bar: 5 μm. (E) TEM analysis showing the formation of autophagic bodies (arrowed) in appressorial vacuoles. Appressoria were induced on the mealworm front wings for 18 h. AP, appressorium; PP, penetration peg. Bar: 0.5 μm.
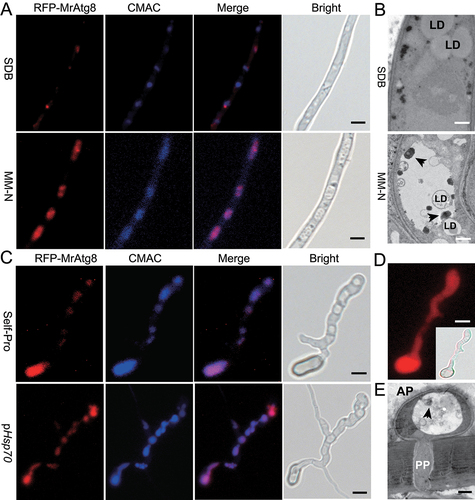
Identification of the virulence-related autophagy genes
Genome survey indicated that different from the budding yeast (38 ATG genes), 24 yeast-like ATG genes are present in the genome of M. robertsii (Table S1) [Citation26,Citation28]. To further interrogate the functional contribution of each gene to the activation of autophagic activities in appressoria, we performed individual gene deletions by homologous replacements and PCR verifications (Fig. S1A). As a result, except for the failure of deleting MrATG6 after multiple trials, 23 null mutants were successfully obtained, including four were acquired before [Citation26]. Relative to the WT strain, substantial phenotypic variations were evident between individual mutants (Fig. S2A). For example, relative to the WT, 16 of 23 mutants had considerable defects of conidiation on potato dextrose agar (PDA), but only one mutant (Mratg20∆) had a significant reduction of sporulation when fungi were grown on a defined complete medium (CM) (Fig. S2B and S2C). Conidial spores harvested from the CM medium were then used for further experiments. No obvious differences were observed between WT and mutants when the fungi were challenged with the osmotic, oxidative and endoplasmic reticulum (ER) stress factors (Fig. S3). Topical infection of the wax moth larvae indicated that the virulence of ten gene-deletion mutants was significantly (P < 0.05) reduced when compared with the WT strain (). Further injection assays were conducted to bypass insect cuticles, and the results indicated that only two of these ten mutants, i.e., Mratg1∆ and Mratg8∆, also had substantial virulence reductions when compared with the WT (Table S2). Taken together, the results indicated that these virulence-related MrATG genes largely contributed to the penetration of insect cuticles.
Table 1. Statistic estimation and comparison of the median lethal time (LT50) of the WT and mutant strains during topic infection of the wax moth larvae
Autophagy genes required for appressorium formation and penetration
To further corroborate the findings mentioned above, we conducted the induction of the infection structure appressoria on different substrates for these virulence-related gene mutants. As a result, the appressorium formation rates of Mratg5∆ (P < 0.05), Mratg7∆ (P < 0.01), Mratg8∆ (P < 0.001), Mratg12∆ (P < 0.05), and Mratg16∆ (P < 0.05) were significantly reduced when compared with that of the WT strain induced on the hydrophobic surfaces (). Appressoria were also induced on cicada wings. In contrast to the hydrophobic surface, only Mratg7∆ (P < 0.01) and Mratg8∆ (P < 0.01) were considerably impaired in appressorium formation in comparison with the WT (). The complementation of Mratg8∆ with either the authentic MrATG8 gene or the RFP-MrATG8 fusion cassette could successfully restore the null mutant ability to produce appressoria (Fig. S1B). Both cellophane and cicada-wing penetration assays were then performed, and the results demonstrated that, except for Mratg13∆, the impairment in penetration could be evident for all other mutants (). Thus, in addition to the defects in appressorium formation of a few mutants, the damage of cuticular penetration might be largely responsible for the virulence reduction of these MrATG gene-deletion mutants.
Figure 2. Appressorial induction and fungal penetration assays. (A) Induction of appressorium formation on hydrophobic surfaces. The WT and virulence-related MrATG gene mutants were induced on Petri dishes for 20 h. (B) Induction of appressorium formation on cicada wings. The WT and virulence-related MrATG gene mutants were induced on cicada wings for 18 h. The significance of difference between WT and individual mutant is at: *, P < 0.05; **, P < 0.01; ***, P < 0.001. (C) Penetration of cellophane membrane. Spore suspensions (2 μl of 1 × 106 conidia/ml) were inoculated on cellophane for four days, removed and the plates were kept for incubation for another six days (upper panels) or on membranes for seven days, removed and the plates kept for incubation for another three days (lower panels). (D) Penetration of cicada wings. Spore suspensions were inoculated on cicada wings for two days, removed and the plates were kept for incubation for another six days (upper panels) or on wings for four days, removed and the plates kept for incubation for another four days (lower panels).
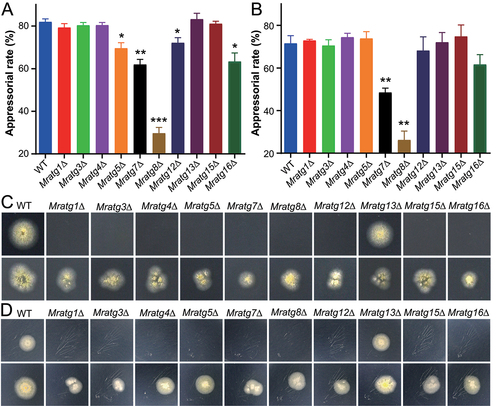
Glycogen and triacylglycerol lipases may not contribute to appressorium maturation
The buildup of a proper turgor pressure is a prerequisite for appressorium maturation and fungal penetration of host cuticles [Citation24,Citation29]. It has been found in M. oryzae that glycogen transfer from mother conidia to appressoria contributes to the generation of turgor pressure [Citation30]. We performed time-course staining of glycogen during Metarhizium appressorium formation, and did not found obvious difference in glycogen distribution between conidial and appressorial cells of both the WT and mutant strains (Fig. S4). Thus, in contrast to M. oryzae [Citation30], cellular glycogen might not contribute to the buildup of the appressorial turgor in M. robertsii. Our previous findings revealed that the LD contents affect fungal penetration and virulence [Citation31–33]; we then hypothesized that LD degradation might primarily impact the appressorial turgor formation in M. robertsii. In yeast cells, the triacylglycerol (TAG) lipases Tgl3, Tgl4 and Tgl5 are localized on LDs for mediating the lipolysis of LD contents [Citation34,Citation35]. The homologs of these lipases are also present in M. robertsii, termed MrTgl3 (EXV02893; 29% identity to yeast Tgl3 at amino acid level), MrTgl4 (EXV05173, 42%) and MrTgl5 (EXV03914, 26%). These three genes were individually deleted (Fig. S1C), and we found that all three mutants had no obvious defects in appressorium formation, cellophane penetration, and topical infection of the caterpillar larvae compared with the WT (Fig. S5). Thus, TAG lipases might not be responsible for LD degradation and turgor generation during appressorial maturation of M. robertsii.
Effects of the virulence-related autophagy genes on Mpl1 accumulation and MrAtg8 lipidation
Similar to the mammalian perilipin, the LD-specific perilipin-like protein Mpl1 localizes on the LD surface and its turnover reflects the level of cellular LD storage in M. robertsii [Citation33]. After co-transforming the WT strain with both the RFP-MrATG8 and GFP-MPL1 fusion cassettes, a time-course induction of appressorium differentiation indicated that LD degradation occurred along with the vacuolar accumulation of RFP-MrAtg8 signals (). Likewise, nitrogen starvation could reduce LD storage compared with the cells grown in a nutrient-rich medium Sabouraud dextrose broth (SDB) (). We found that LD storage had no obvious difference between the WT and mutant conidia harvested from the CM medium as indicated by fluorescent staining of LDs (). Consistently, a similar accumulation level of Mpl1 was detected in the conidial cells of different strains by western blot analysis with an anti-Mpl1 antibody ().
Figure 3. Lipid droplet degradation and accumulation. (A) Time-scale monitoring of LD degradation and formation of the RFP-MrAtg8 signal in appressorial cells. The co-transformant spores were induced on a hydrophobic surface for different times. Bar: 5 µm. (B) Monitoring of the LD distribution and RFP-MrAtg8 signal in mycelia harvested from different conditions. The co-transformed strain was grown in SDB for two days, harvested and washed prior to the inoculation in MM-N for 16 h. Bar: 5 μm. (C) LD accumulation within the WT and mutant conidia. Conidia of the WT and virulence-related mutants were harvested from the two-week-old CM plates and stained with the specific dye Bodipy. Bar: 5 μm.
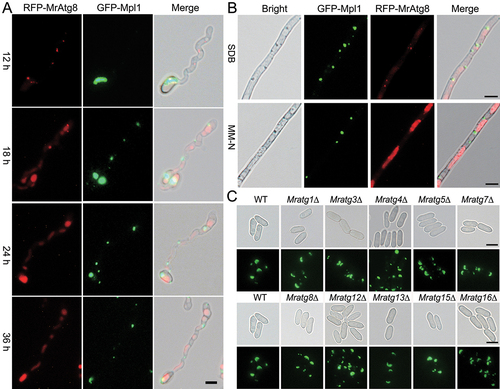
Figure 4. Features of protein expression and degradation in different strains. (A) Comparison of the LD-specific protein Mpl1 accumulation in conidia. Conidia of the WT and mutants were harvested from two-week-old CM plates and homogenized for western blot analysis. (B) Analysis of Mpl1 protein between strains after nitrogen starvation. The anti-Mpl1 antibody was used for blotting. (C) Analysis of MrAtg8 protein between WT and mutants after nitrogen starvation. The anti-Atg8 antibody was used for blotting. (D) Analysis of GFP-MrAtg8 degradation in the WT and mutant mycelial cells after nitrogen starvation. The anti-GFP antibody was used for blotting. For each analysis, the parallel-running protein gels were stained as references.
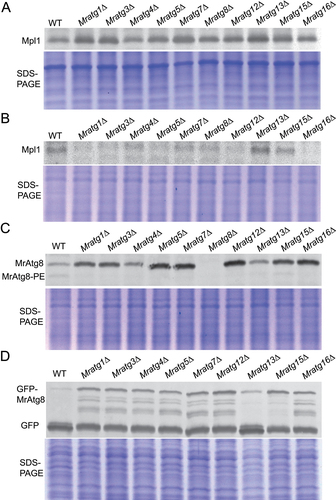
To further determine the Mpl1 degradation pattern in different mutants, we transformed the GFP-MPL1 fusion cassette into both the WT and virulence-related MrATG mutants. When grown in SDB, cellular Mpl1 level was similar between WT and mutants (Fig. S6A), and the degradation pattern of the GFP-Mpl1 fused protein was also similar between WT and mutants (Fig. S6B). In contrast, except for Mratg15∆, the fused protein GFP-Mpl1 was completely degraded in the WT and other mutant cells after the growth in a nitrogen-depleted medium (Fig. S6C). Upon nitrogen starvation, cellular Mpl1 levels in the mutants (except for Mratg13∆ and Mratg15∆) were more highly reduced than that observed in the WT ( and S6D). We also found that, except for Mratg13∆ and Mratg15∆, MrAtg8 lipidation was impaired in other virulence-related gene mutants when compared with the WT (), which was consistent with the irregular degradation pattern of the GFP-MrAtg8 protein in the mutant cells after nitrogen starvation (). This pattern was in contrast to fungal growth in the SDB medium (Fig. S6E). Thus, the virulence-related MrAtg proteins might involve in direct or indirect control of the Mpl1 turnover and MrAtg8 lipidation.
Effects of the virulence-related autophagy genes on LD engulfment and degradation
To further determine the virulence-related MrATG genes’ effect on LD degradation, we performed TEM analysis of the WT and five selected mutants after nitrogen starvation. The results revealed that LDs could be engulfed in mutant vacuoles by the formation of either larger (Mratg1∆, Mratg4∆, Mratg7∆ and Mratg8∆) or multiple (Mratg15∆) droplets compared with the WT (). Before LD entry into vacuoles, no obvious difference in LD sizes was found between the WT and mutants (). Subsequent quantification analysis revealed that relative to those of the WT, TAG content became significantly (P < 0.05) elevated, especially in Mratg7∆ (P = 0.004) (). In consequence, total glycerol content turned to be substantially (P < 0.05) reduced in these mutant cells after nitrogen starvation (). It was evidenced, therefore, that LD entry into vacuoles became unaffected, but LD degradation was impaired in mutant cells.
Figure 5. Variation in LD accumulation and degradation between strains. (A) TEM analysis of the vacuolar localization features of LDs in the WT and mutant mycelial cells after nitrogen starvation. The autophagic bodies are arrowed in the WT cell. Bar: 0.5 μm. (B) TEM featuring of the LDs outside the vacuoles of the WT and mutant mycelial cells after nitrogen starvation. Bar: 2 μm. (C) Quantification and comparison of the cellular TAG content between WT and mutants after nitrogen starvation. (D) Quantification and comparison of the cellular glycerol level between WT and mutants after nitrogen starvation. Fungal spores were inoculated in SDB for two days and transferred to the MM-N medium for 16 h. The significance of difference between WT and individual mutant is at: *, P < 0.05; **, P < 0.01; ***, P < 0.001.
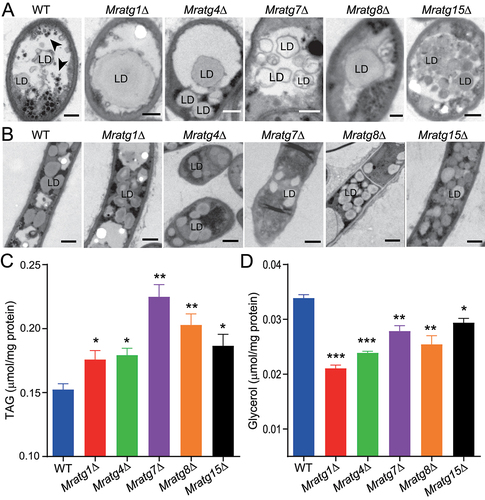
The requirement of the virulence-related autophagy genes on the formation of autophagic bodies
Autophagic bodies formed in vacuoles mediate the degradation of autophagic substrates in fungi [Citation36]. To interrogate the potential mechanism(s) resulting in the failure of LD degradation in different mutants, we examined the cellular formation of vacuoles and autophagic bodies. As a result, we found that vacuole formation was not affected in the virulence-related mutant appressorial cells or mycelia after nitrogen starvation by staining with a specific fluorescent dye (Fig. S7). However, except for Mratg13∆ and Mratg15∆, the vacuolar accumulation of the RFP-MrAtg8 signal was impaired in other mutant appressoria compared with those of the WT (). Consistently, relative to the WT, impairment of the autophagic body formation was evident in mutant vacuoles (). The similarly impaired or unimpaired accumulation of the RFP-MrAtg8 signal was also observed in mutant mycelia after nitrogen starvation (Fig. S8A). In contrast, a weak signal of RFP-MrAtg8 was similarly observed in each strain after the growth in the nutrient-rich SDB medium (Fig. S8B).
Selective interactions between MrAtg8 and other proteins
Different Atg protein complexes will be formed during autophagic processes [Citation15]. Considering that, except for Mratg13∆ and Mratg15∆, the vacuolar translocation of MrAtg8 was damaged in other virulence-reduced MrATG mutants; we examined the potential interactions between MrAtg8 and other virulence-related MrAtg proteins by yeast two-hybrid analysis. It was found that MrAtg8 could only interact with MrAtg7 and MrAtg15 (). We also examined the potential interaction between Mpl1 and MrAtg8, and between Mpl1 and MrAtg15. As a result, positive interactions were not evident between these two protein pairs (). To further determine whether MrAtg8 and MrAtg15 had a colocalization effect, we transformed the RFP-MrATG8 strain with an MrATG15-GFP fusion cassette. As a result, we found that both fluorescent signals could be well overlapped in the vacuoles of the appressorial and mycelial cells after nitrogen starvation (). In addition, it was found that deletion of MrATG8 did not impair the vacuolar location of MrAtg15-GFP ().
Figure 7. Protein interaction and colocalization assays. (A) Positive and negative interactions between MrAtg8 and other virulence-related proteins by yeast two-hybrid analysis. (B) Non-interaction between the perilipin Mpl1 and MrAtg8 or MrAtg15. (C) Colocalization of RFP-MrAtg8 and MrAtg15-GFP in vacuoles. Appressoria were induced on a hydrophobic surface. Nitrogen starvation was treated in MM-N medium for 4 h after spore germination in SDB for 16 h. Bright/CMAC means the overlap of the bright-field image with the CMAC-stained image for vacuoles. CO, conidium; AP, appressorium. Bar: 5 μm. (D) Location of MrAtg15-GFP in the vacuoles of the Mratg8∆ cell. The mutant spores were induced on a hydrophobic surface for 20 h. CO, conidium; Bar: 5 μm.
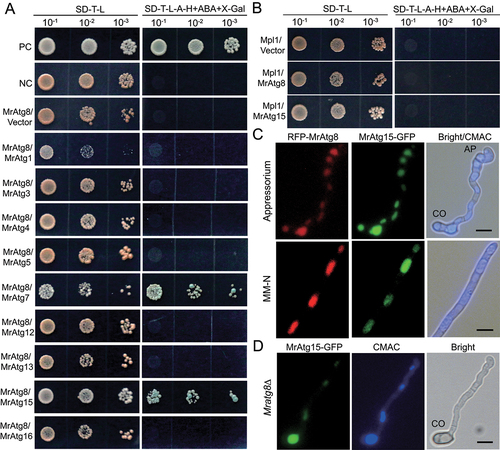
Discussion
In this study, we found that autophagic fluxes were activated along with the appressorium formation in the insect pathogenic fungus M. robertsii. After genome-wide deletion of the 23 MrAtg genes encoded in M. robertsii, 10 genes were found with substantial contributions to topical fungal infection of insect hosts. The null mutants were largely impaired in penetrating insect cuticles. We found that LD degradation mediated by a microlipophagy machinery is potentially implicated in building up the appressorium turgor pressure. Thus, direct engulfment of LD into vacuoles was evident, and the process could not be blocked after deletion of the autophagic genes involved in mediating autophagosome formation. However, the followed-up LD lipolysis was impaired in mutant vacuoles with defects in autophagic body formation. We, therefore, unveiled that the activation and contribution of microlipophagy to fungal infection biology ().
Figure 8. Schematic representation of the activation of microlipophagy in the appressorial cells of M. robertsii. The virulence-related autophagy components identified in this study are listed that may alternatively contribute to the activation of cell autophagy, lipidation of MrAtg8 and LD degradation. The autophagosome-mediated transfer of LDs into vacuoles may not occur based on the observations in this study, thus the employment of microlipophagic machinery to facilitate fungal early infection. PAS, phagophore assembly site; PE, phosphatidylethanolamine; AB, autophagic body.
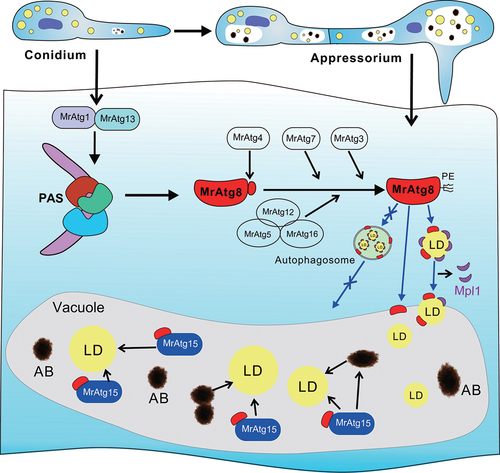
The formation of the infection structure is the start for fungi to establish a nutritional relationship with hosts [Citation24,Citation37]. Except for waxes, both plant and insect cuticular surfaces are nutrient-poor [Citation38,Citation39]. It is, therefore, not surprising to detect the activation of autophagic activities within the appressorial cells of M. robertsii. Based on the functional studies of the ATG genes in yeast (Table S1) [Citation1,Citation15], it is postulated that these virulence-related MrATG genes may involve in autophagy induction/initiation, Atg8 lipidation, and autophagic body formation (). Thus, besides the core MrATG8 gene, the yeast-like ATG1, ATG3, ATG4, ATG5, ATG7, ATG12, ATG13, ATG15 and ATG16 are required for the full virulence of both insect and plant pathogenic fungi [Citation40]. Functional divergence of the homologous Atg proteins has also been found in different organisms. For example, we found that appressorial formation was substantially impaired after deletion of MrATG8 in M. robertsii. In contrast, Moatg8∆ of M. oryzae could produce the WT-like appressoria [Citation19]. Even being putatively required to form the MrAtg1-MrAtg13 complex, divergent effects were found between these two proteins on mediating fungal penetration () and the vacuolar accumulation of MrAtg8 (). Likewise, the functional divergence has been found between Atg1 and Atg13 in yeast [Citation41] and between MoAtg1 and MoAtg13 in M. oryzae [Citation27]. Otherwise, we found that MrATG9 was not required for the virulence of M. robertsii, which is in contrast to the findings of the infection requirement of the ATG9-like gene in other pathogenic fungi [Citation20,Citation23,Citation42]. The exact mechanism of functional divergence between Atg proteins requires further investigations in different organisms.
Following the process of differentiation, appressorium maturation requires the generation of turgor pressure to facilitate fungal penetration of host cuticles [Citation24,Citation37,Citation43]. In contrast to the findings of the glycogen transfer and TAG lipase activity required for appressorium maturation in M. oryzae [Citation30], we found that glycogen was similarly distributed in mother conidia and appressoria of the WT and virulence-related MrATG mutants. Deletion of MrTGL genes did not impair the penetration and virulence of M. robertsii. Thus, autophagy-mediated LD degradation mainly contributes to the turgor generation in M. robertsii. We found that disruption of the MrATG1 and MrATG8 genes also reduced fungal virulence during injection assays (Table S2), indicating that autophagic genes contribute to fungal pathogenicity beyond the involvement in cuticle penetration.
It has been indicated in yeast cells that the Atg1 complex mediates the initiation of autophagy to form the phagophore assembly site while the Atg8-conjugation system sequentially coordinates the expansion of phagophore to form autophagosomes [Citation15,Citation44]. The null mutants of these related genes experience defects in forming autophagosomes [Citation44–46]. With this in mind, we found that vacuolar engulfment of LDs was not blocked in the null mutants of these core genes. The data, therefore, provide genetic evidence to support the microlipophagic machinery of LD degradation in M. robertsii, i.e., without the assistance of autophagosomes for vacuolar internalization of LDs (). It has been similarly found in mammalian cells that knockdown of ATG5 or the gene responsible for CMA did not inhibit the direct ingestion of LDs by lysosomes [Citation47]. In addition, it has been found in M. oryzae that fungal early infection could trigger ER stress responses and the ER stress factor dithiothreitol (DTT) induced autophagy [Citation48]. In yeast cells, ER stress factors such as DTT and tunicamycin could induce microlipophagy [Citation10]. Taken together, it is supportive to conclude that microlipophagy is activated during appressorium formation of M. robertsii, and it is technically feasible to detect the activation of microlipophagy by monitoring vacuolar/lysosomal engulfment of LDs in core autophagic gene-deletion mutants.
We found that the engulfed LDs were accumulated in vacuoles without degradation in the virulence-related mutants, which was supported by the TAG content increase while glycerol content decrease in mutant cells when compared with the WT. The defects of MrAtg8 lipidation/translocation and or autophagic body formation would be the cause for the LD-degradation failure within the mutant cells [Citation49]. Different from other mutants, the WT-like MrAtg8 signal could be observed in Mratg15∆ cell vacuoles. Considering that yeast Atg15 is responsible for LD and autophagic body breakdown in vacuoles [Citation8,Citation50], it is not surprising to find that deletion of MrATG15 also resulted in vacuolar accumulation of LDs. Our findings, therefore, indicate that the microlipophagic processes of LD engulfment and degradation occur separately, and the former process can take place without inhibition by the downstream lipolytic defects. Considering the possibility of co-occurrence of the macro- and microlipophagy in yeast and mammalian cells [Citation6,Citation13], we cannot rule out at this stage that both types of machinery can occur in M. robertsii in association with fungal developments and nutrient availabilities.
Providing that LD degradation being blocked in the virulence-related gene mutants, it is intriguing to find that LD sizes varied in the vacuoles of different mutants (). Cellular LD size reflects a balance of lipogenesis and lipolysis, and both the LD surface proteins (e.g., PLIN/perilipin and BSCL2/seipin) and phospholipid moieties jointly control the content and size of LDs [Citation31,Citation33,Citation51]. A reduced level of PLIN echoes either the reduction of LD contents or the increase of LD size. Taken together with the quantification of TAG content (), the reduction (Mratg1∆, Mratg4∆, Mratg7∆ and Mratg8∆) or non-reduction (Mratg15∆) of Mpl1 level was generally consistent with the LD sizing patterns observed in mutants after nitrogen deprivation. On the other hand, our finding of a rather complete degradation of GFP-Mpl1 in the WT and mutant cells after nitrogen starvation would suggest that Mpl1 might be released before LD entry into vacuoles, which led to the formation of the large-sized LDs in vacuoles (). The studies of the LD proteome have revealed dynamic protein features associated with growth nutrients [Citation52–54]. It has been found in M. robertsii that the LD-surface proteins containing a DUF3129 domain were highly upregulated in appressoria in association with Mpl1 turnover and fungal virulence [Citation55]. The involvement of both the Atg and non-Atg proteins in microlipophagy warrants further investigations.
We found that, except for MrAtg7 and MrAtg15, positive interactions were not detected between MrAtg8 and other virulence-related Atg components in yeast two-hybrid analysis. This observation is different from the findings in yeast [Citation56]. For example, regarding the Atg8–PE conjugation system, studies have revealed that Atg3, Atg4 and Atg7 interact with Atg8 through the Atg8-family interacting motif (AIM) W/YxxL in yeast cells [Citation57,Citation58]. Atg1 can also interact with Atg8 via the AIM motif [Citation59]. Consistent with the results of our two-hybrid analysis, the AIM-like motif WGCL (502–505 amino acid [aa] residues) is present in MrAtg15 while MrAtg7 contains three YxxL motifs [i.e., YSAL (19–22 aa), YDKL (29–32 aa), YADL (106–109 aa)]. We found the positive interaction between MrAtg8 and MrAtg15 and their colocalization in vacuoles after nitrogen starvation of the fungus, which suggests that both proteins might form a complex for the formation and degradation of LDs and autophagic bodies (). It is noteworthy that an AIM motif is also present in the yeast Atg15 lipase (YFAL, 32–35 aa). To our knowledge, the interaction between Atg8 and Atg15 has not been examined and reported before. Within the co-transformed strain, partial fluorescence overlap could be observed between RFP-MrAtg8 and GFP-Mpl1 signals in appressorial and mycelial cells (), and both proteins could be detected in the LD proteome data of M. robertsii [Citation54]. However, direct interaction was not evident between MrAtg8 and Mpl1, nor between Mpl1 and MrAtg15 (). Future efforts are still required to determine the dynamic interaction and formation of protein complexes during microlipophagy in fungi and other organisms.
In conclusion, we report the activation and contribution of the characteristic microlipophagy to mediate LD degradation in the insect pathogenic fungus M. robertsii during early infection of insects. The results of this study advance the understanding of the functional insights of microlipophagy in controlling fungal virulence, and may benefit future investigation of the microlipophagic effect and mechanism in different organisms. Taken together with the findings in yeast and mammalian cells, our results also suggest that microlipophagic degradation of LDs might commonly occur but remain largely neglected in different organisms.
Material and methods
Fungal strains and growth conditions
The WT and mutant strains of M. robertsii ARSEF 2575 (USDA ARS Collection of Entomopathogenic fungal Cultures) were routinely grown on PDA (BD Difco, BD 213300) or a complete medium [CM; 6 g/L NaNO3; 0.52 g/L KCl; 1 g/L yeast extract (Oxoid, LP0021); 0.52 g/L MgSO4 · 7H2O; 0.25 g/L KH2PO4; 1 g/L casamino acid (Qisong Bio, QS81015505); 1 g/L glucose (Sinopharm, 10021463); 1 ml/L trace elements; 1 ml/L vitamin supplement] for sporulation induction at 25°C for 14–20 d [Citation32]. The trace element solution (100 ml) was prepared with: ZnSO4•7H2O, 2.2 g; H3BO3, 1.1 g; MnCl2•4H2O, 0.5 g; FeSO4•7H2O, 0.5 g; CoCl2•6H2O, 0.17 g; CuSO4•5H2O, 0.16 g; Na2MoO4•5H2O, 0.15 g and Na4EDTA, 5 g. The vitamin supplement (100 ml) was prepared with: Biotin (Aladdin, B105434), 0.01 g; Pyridoxine (TargetMol, T0973), 0.01 g; Thiamine (Serva, 3,602,002) 0.01 g, Riboflavin (Adamas, 85,641 C), 0.01 g; P-aminobenzonic acid (Adamas, 25,459 C), 0.01 g and Nicotinic acid (TargetMol, T0879), 0.01 g. For nutrient starvation, fungi were cultured on a minimum medium (MM; 6 g/L NaNO3, 0.52 g/L KCl, 0.52 g/L MgSO4 · 7H2O, 0.25 g/L KH2PO4) [Citation60]. For comparing the stress responses between WT and mutants, the cultures were grown on PDA amended with 0.5 M NaCl, 0.5 M KCl, 0.5 M sorbitol (Ebaiao, 04-GS172), 1 mM DTT (Yeasen, 20311ES10), or 2 mM H2O2 (Aladdin, H112520) at 25°C. The cultures were also incubated at 37°C for the temperature stress test. For characterizing the autophagic effects after fungal growth in different nutrients, conidia collected from the 14-day-old CM agar were inoculated in SDB (BD Difco, BD 238230) for 16 h. Germlings were collected, washed three times with sterile water, and then re-grown for 4 h in the MM without nitrogen (MM-N) source, MM without carbon source, or MM without both carbon and nitrogen sources plus 2 mM phenylmethylsulfonyl fluoride (PMSF; Sigma-Aldrich, P7626) [Citation54].
Gene deletions and fungal transformation
To determine the potential contribution of each MrATG gene to the full function of appressorial formation and fungal virulence, we deleted 24 putative yeast-like ATG genes encoded in M. robertsii (Table S1) in this study. Gene deletions were performed by homologous recombination using the Agrobacterium tumefaciens (Mingzhou Bio, BMZ115299)-mediated transformation method as described in our previous study [Citation61]. Briefly, the 1–1.5 kb of the 5ʹ- and 3ʹ-flanking regions of each MrATG gene were amplified by PCR using genomic DNA as the template with different primer pairs (Table S3). The products were purified and subsequently inserted into the binary vector pDHt-bar (modified from the plasmid pDHt-SK [Addgene, 63615; deposited by Seogchan Kang] with a Bar gene for conferring glufosinate resistance) to produce the pBar-ATG knockout vectors to transform the WT strain of M. robertsii. The homologous triacylglycerol-lipase genes, i.e., MrTGL3 (EXV02893), MrTGL4 (EXV05173) and MrTGL5 (EXV03914) [Citation28], were also deleted in M. robertsii. The obtained mutants were verified by PCR using the YF/YR primers (Table S3). At least three independent null mutants were selected during deletion of each gene, and one stable strain was randomly selected for different experiments.
Protein fusions and localization assays
To determine the localization of MrAtg8 in different cells, we fused the MrAtg8 protein with either a red fluorescent protein (RFP) or a green fluorescent protein (GFP) at its N terminus. The fusion gene cassettes were cloned into the vector pDHt-Ben under the control of either the MrATG8 gene promoter or an HSP70 gene constitutive promoter of M. robertsii [Citation62]. The LD-specific protein Mpl1 was fused with a GFP protein at N terminus. The putative lipase MrAtg15 was fused with the GFP protein at C terminus [Citation50]. Each cassette was cloned into the vector pDHt-Sur (modified from pDHt-SK with a Sur gene for conferring sulfonylurea resistance) with the control of a TEF-gene promoter of M. robertsii [Citation63]. The constructed vectors were used to individually transform the WT strain, and MrATG null mutants to determine the difference of protein localization in fungal conidia, appressoria, or germlings under various nutrient conditions. As a control, the RFP gene was also made under the control of the HSP70 promoter of M. robertsii to transform the WT and Mratg8∆ strains.
Insect bioassays
Insect bioassays were conducted using the newly emerged last instar larvae of the wax moth (G. mellonella; Keyun Biotech, GM01) larvae to examine of the effect of MrATG gene deletion on fungal virulence. In brief, conidia of the WT, MrATG and MrTGL mutants were collected from the two-week-old CM plates and suspended in 0.05% Tween-20 (v:v; Sigma-Aldrich, P2287). For topical infection, the insects were immersed in each suspension containing 2 × 107 conidia/ml for 30 s. The treated insects were kept at a high moisturized condition for the first 24 h [Citation64]. Immersion assays were performed in two separate batches by including the WT strain as a control due to a large number of mutant strains (23 in total). The mutant strains with a significant reduction in topical infection were further subject to injection assays. Thus, 20 μl of spore suspensions (1 × 104 conidia/ml) were injected at the second proleg of each insect. Each treatment had three replicates with 20 insects each, and the experiments were repeated three times. The insects treated with 0.05% Tween-20 were used as a mock control. Insect mortality was recorded every 12 h. The medial lethal time values were calculated by Kaplan-Meier analysis for each strain, and the survival differences between WT and individual MrATG null mutant were estimated by the Log-rank test [Citation65]. Conidia of the WT and virulence-related mutants harvested from the two-week-old PDA plates were stained with the LD-specific fluorescent dye Bodipy (Thermo Fisher Scientific, D3922).
Appressorium induction and penetration assay
For appressorium induction on the hydrophobic surfaces, conidia from the WT and virulence-related MrATG deletion strains were inoculated into a sterile polystyrene Petri dish (6 cm in diameter, Corning, 430166) containing 2 ml of 0.0125% yeast extract (Oxoid, LP0021) at a final concentration of 1 × 106 conidia/ml [Citation66]. For appressorium induction on insect wings, the transparent wings of the cicada (Cryptotympana atrata) collected from the field were used for microscopic examination of appressorium formation rates, and the thick front wings of mealworm (Tenebrio molitor; Xincheng Bio, 003) were used for preparing ultrathin sections for TEM analysis. The insect wings were immersed in an aqueous suspension containing 4 × 107 conidia/ml for 30 s and then lined on the 0.8% water agar. After incubation for 20 h (hydrophobic plastic surface) or 18 h (insect wings), appressorium differentiation rates of different strains were estimated by examining at least 300 spores per strain under different fields of the microscope. For cellophane membrane penetration assay, 2 μl of the WT and mutant conidial suspension (1 × 106 conidia/ml) were inoculated on the sterilized cellophane membrane (Dingguo Biotech, XH444-1) lined on the MM medium. After four or seven days, the membranes together with fungal cultures were carefully removed and the plates were kept for incubation for another three or six days to determine fungal penetration ability [Citation67]. For penetration of cicada wings, the intact wings were surface sterilized with H2O2 and lined on the MM medium prior to the inoculation of conidial suspension (1 μl each; 2 × 106 conidia/ml) in the middle. After incubation for two or four days, the wings with fungal cultures were gently removed and the plates were kept for incubation for another four or six days to determine the penetration difference between WT and mutant strains.
Western blotting analysis
The WT and virulence-related mutant strains were cultured under different nutrient conditions for protein extraction and western blotting analysis. Conidia of different strains were harvested from 14-day-old CM plates for inoculations, and the mycelia grown in different nutritional conditions were collected and washed three times with sterile water prior to protein extraction. For nitrogen starvation, conidia were inoculated in SDB for 2 d and mycelia were harvested by filtration, washed thrice with sterile water and then inoculated into the MM-N medium for another 16 h. Fungal samples were homogenized with a tissue crusher for total protein extraction with the RIPA lysis buffer (Beyotime, P0013K) containing 1 mM protease inhibitor PMSF. The total protein concentration of each sample was estimated using a bicinchoninic acid protein assay kit (Beyotime, P0010). Different protein samples were adjusted to equal amounts: 15 μg per conidial proteins or 20 μg per mycelial proteins for sodium dodecyl sulfate-polyacrylamide gel electrophoresis (SDS-PAGE) and western blotting analysis. The protein sample of each strain was boiled with the SDS loading buffer, separated by 15% SDS-PAGE gel and then transferred to the polyvinylidene difluoride membranes (Merck, P2938). Membrane probing was conducted with the antibodies against Mpl1 and MrAtg8 raised before [Citation26,Citation54]. The anti-GFP antibody (Beyotime, AF1483) was also used for blotting analysis to evaluate the degradation pattern of the GFP-Mpl1 and GFP-MrAtg8 fused proteins after the fungi were grown in different nutrient media. The parallel-running SDS-PAGE gels were stained and imaged as loading controls.
Fluorescent staining and glycogen staining
The WT and virulence-related mutant cells were subjected to fluorescent staining with different dyes. For vacuole staining, the WT and gene-fusion mutant conidia were induced on the hydrophobic surfaces, and germling samples were washed three times with phosphate-buffered saline (PBS; Sangon Biotech, B548117, pH 7.4) and then stained with a fluorescent dye 7-amino-4-chloromethylcoumarin (CMAC; Thermo Fisher Scientific, C2110) [Citation2]. The dye was dissolved in dimethyl sulfoxide to obtain a 10 mM stock solution and samples were incubated with 10 μM of CMAC dye for 30 min. The samples were washed thrice with PBS prior to imaging with a fluorescence microscope (Nikon, DS-Ri2). For glycogen staining, fungal conidia and appressoria were washed twice with PBS prior to the treatment with the staining solution consisting of 10 mg/ml of I2 and 60 mg/ml of KI. The samples were then subjected to the microscope examination for imaging the yellowish-brown glycogen deposits [Citation42].
TEM analysis
TEM analysis was conducted to examine the vacuolar engulfment of LDs, formation or non-formation of autophagic bodies within the vacuoles of the WT and mutant cells. Thus, the WT strain spores were inoculated on the front wings of mealworm for 24 h for appressorium induction. Alternatively, conidia of different strains collected from the 14-day-old CM plates were grown in SDB for two days and then regrown in the MM-N medium for 16 h. The samples were collected, washed and were fixed overnight in 2.5% glutaraldehyde in 0.1 M PBS (pH 7.4) at 4°C. The samples were washed thrice with the PBS buffer prior to the fix in 1% osmium tetroxide buffered with 0.1 M cacodylate (pH 7.0) at 4°C overnight. After washing with the PBS buffer for three times, the samples were dehydrated in a gradient ethanol series and infiltrated with a series of epoxy resin in epoxy propane prior to the embedment in Epon-812 resin (Merck, 45435). Finally, the ultrathin sections of each sample were stained in 2% uranium acetate followed by lead citrate and visualized under a transmission electron microscope (Hitachi, H-7650) operating at 80 kV [Citation64].
Glycerol and TAG quantification assays
The WT and selected virulence-related mutants were grown in SDB for two days and then subjected to nitrogen starvation by growing in the MM-N medium for 16 h. The mycelia were harvested and washed thrice with sterile water. Glycerol or triglyceride content in the samples was extracted and assayed using the glycerol assay kit (Applygen, E1012) or triglyceride assay kit (Applygen, E1013). The protein concentration of each sample was quantified using a BCA Protein Assay Kit (Beyotime, P0012). The concentration of glycerol and triglyceride was expressed as the unit micromolar of glycerol or triglyceride per milligram of total protein [Citation31]. All experiments were repeated twice with three independent replicates per strain.
Yeast two-hybrid assays
To test the potential interactions between MrAtg8 and other MrAtgs, or between Mpl1 and other proteins, we used the bait vector to generate pGBKT7-MrAtg8 by cloning MrATG8 cDNA into the vector pGBKT7 (Takara Bio, 630443). The vectors pGADT7-MrATGs were constructed as preys by cloning individual MrATG gene cDNA into the plasmid pGADT7 (Takara Bio, 630442) [Citation62]. The bait and prey fusion constructs were co-transformed into the yeast strain Y2H Gold by following the manufacturer’s protocols (Takara Bio, 630489). The plasmids pGADT7 and pGBKT7 were co-transformed into the yeast cells as a positive control [Citation26], while the paired vectors (pGADT7 and pGBKT7; pGADT7 and pGBKT7-MrATG8; pGADT7 and pGBKT7-MPL1) co-transformed into the yeast cells as negative controls. Positive clones from the synthetic dropout-Leu-Trp medium were transferred to the synthetic dropout-Leu-Trp-Ade-His medium to assess protein interactions.
Statistical analyses
The two-tailed Student’s t-test was conducted to determine the difference of statistics between WT and different mutants. The significance level of difference between strains was classified as follows: *, P < 0.05; **, P < 0.01; and ***, P < 0.001, which are noted in the figure legends.
Supplemental Material
Download Zip (11.3 MB)Acknowledgments
We thank Qiang Gao for thoughtful discussions, and Zhiping Zhou and Xiaoyan Gao for help with transmission electron microscopy analysis.
Disclosure statement
No potential conflict of interest was reported by the author(s).
Supplementary material
Supplemental data for this article can be accessed here.
Additional information
Funding
References
- Klionsky DJ. Autophagy: from phenomenology to molecular understanding in less than a decade. Nat Rev Mol Cell Biol. 2007;8:931–937.
- Klionsky DJ, Abdel-Aziz AK, Abdelfatah S, et al. Guidelines for the use and interpretation of assays for monitoring autophagy (4th edition). Autophagy. 2021;17:1–382.
- Ohsumi Y. Historical landmarks of autophagy research. Cell Res. 2014;24:9–23.
- Galluzzi L, Baehrecke EH, Ballabio A, et al. Molecular definitions of autophagy and related processes. EMBO J. 2017;36:1811–1836.
- Mizushima N, Yoshimori T, Ohsumi Y. The role of Atg proteins in autophagosome formation. Annu Rev Cell Dev Biol. 2011;27:107–132.
- Schuck S. Microautophagy - distinct molecular mechanisms handle cargoes of many sizes. J Cell Sci. 2020;133:jcs246322.
- Sieńko K, Poormassalehgoo A, Yamada K, et al. Microautophagy in plants: consideration of its molecular mechanism. Cells. 2020;9:887.
- van Zutphen T, Todde V, De Boer R, et al. Lipid droplet autophagy in the yeast Saccharomyces cerevisiae. Mol Biol Cell. 2014;25:290–301.
- Wang CW, Miao YH, Chang YS. A sterol-enriched vacuolar microdomain mediates stationary phase lipophagy in budding yeast. J Cell Biol. 2014;206:357–366.
- Garcia EJ, Liao PC, Tan G, et al. Membrane dynamics and protein targets of lipid droplet microautophagy during ER stress-induced proteostasis in the budding yeast, Saccharomyces cerevisiae. Autophagy. 2020;1–21. doi: 10.1080/15548627.2020.1826691
- Vevea JD, Garcia EJ, Chan RB, et al. Role for lipid droplet biogenesis and microlipophagy in adaptation to lipid imbalance in yeast. Dev Cell. 2015;35:584–599.
- Hashemi HF, Goodman JM. The life cycle of lipid droplets. Curr Opin Cell Biol. 2015;33:119–124.
- Goodman JM. The importance of microlipophagy in liver. Proc Natl Acad Sci USA. 2021;118:e2024058118.
- Farré JC, Subramani S. Mechanistic insights into selective autophagy pathways: lessons from yeast. Nat Rev Mol Cell Biol. 2016;17:537–552.
- Wen X, Klionsky DJ. An overview of macroautophagy in yeast. J Mol Biol. 2016;428:1681–1699.
- Hofius D, Li L, Hafrén A, et al. Autophagy as an emerging arena for plant-pathogen interactions. Curr Opin Plant Biol. 2017;38:117–123.
- Palmer GE, Askew DS, Williamson PR. The diverse roles of autophagy in medically important fungi. Autophagy. 2008;4:982–988.
- Ying SH, Feng MG. Insight into vital role of autophagy in sustaining biological control potential of fungal pathogens against pest insects and nematodes. Virulence. 2019;10:429–437.
- Veneault-Fourrey C, Barooah M, Egan M, et al. Autophagic fungal cell death is necessary for infection by the rice blast fungus. Science. 2006;312:580–583.
- Kershaw MJ, Talbot NJ. Genome-wide functional analysis reveals that infection-associated fungal autophagy is necessary for rice blast disease. Proc Natl Acad Sci USA. 2009;106:15967–15972.
- Lv W, Wang C, Yang N, et al. Genome-wide functional analysis reveals that autophagy is necessary for growth, sporulation, deoxynivalenol production and virulence in Fusarium graminearum. Sci Rep. 2017;7:11062.
- Zhao X, Feng W, Zhu X, et al. Conserved autophagy pathway contributes to stress tolerance and virulence and differentially controls autophagic flux upon nutrient starvation in Cryptococcus neoformans. Front Microbiol. 2019;10:2690.
- Jiang ST, Chang AN, Han LT, et al. Autophagy regulates fungal virulence and sexual reproduction in Cryptococcus neoformans. Front Cell Dev Biol. 2020;8:374.
- Wang CS, Wang SB. Insect pathogenic fungi: genomics, molecular interactions, and genetic improvements. Annu Rev Entomol. 2017;62:73–90.
- Wang CS, Feng MG. Advances in fundamental and applied studies in China of fungal biocontrol agents for use against arthropod pests. Biol Control. 2014;68:129–135.
- Duan ZB, Chen YX, Huang W, et al. Linkage of autophagy to fungal development, lipid storage and virulence in Metarhizium robertsii. Autophagy. 2013;9:538–549.
- Liu XH, Gao HM, Xu F, et al. Autophagy vitalizes the pathogenicity of pathogenic fungi. Autophagy. 2012;8:1415–1425.
- Hu X, Xiao G, Zheng P, et al. Trajectory and genomic determinants of fungal-pathogen speciation and host adaptation. Proc Natl Acad Sci USA. 2014;111:16796–16801.
- Wilson RA, Talbot NJ. Under pressure: investigating the biology of plant infection by Magnaporthe oryzae. Nat Rev Microbiol. 2009;7:185–195.
- Thines E, Weber RW, Talbot NJ. MAP kinase and protein kinase A-dependent mobilization of triacylglycerol and glycogen during appressorium turgor generation by Magnaporthe grisea. Plant Cell. 2000;12:1703–1718.
- Gao Q, Lu Y, Yao H, et al. Phospholipid homeostasis maintains cell polarity, development and virulence in Metarhizium robertsii. Environ Microbiol. 2016;18:3976–3990.
- Chen YX, Li B, Cen K, et al. Diverse effect of phosphatidylcholine biosynthetic genes on phospholipid homeostasis, cell autophagy and fungal developments in Metarhizium robertsii. Environ Microbiol. 2018;20:293–304.
- Wang CS, St Leger RJ. The Metarhizium anisopliae perilipin homolog MPL1 regulates lipid metabolism, appressorial turgor pressure, and virulence. J Biol Chem. 2007;282:21110–21115.
- Gaspar ML, Hofbauer HF, Kohlwein SD, et al. Coordination of storage lipid synthesis and membrane biogenesis: evidence for cross-talk between triacylglycerol metabolism and phosphatidylinositol synthesis. J Biol Chem. 2011;286:1696–1708.
- Kurat CF, Natter K, Petschnigg J, et al. Obese yeast: triglyceride lipolysis is functionally conserved from mammals to yeast. J Biol Chem. 2006;281:491–500.
- Klionsky DJ, Abdelmohsen K, Abe A, et al. Guidelines for the use and interpretation of assays for monitoring autophagy (3rd edition). Autophagy. 2016;12:1–222.
- Ryder LS, Talbot NJ. Regulation of appressorium development in pathogenic fungi. Curr Opin Plant Biol. 2015;26:8–13.
- Ortiz-Urquiza A, Keyhani NO. Action on the surface: entomopathogenic fungi versus the insect cuticle. Insects. 2013;4:357–374.
- Yan X, Talbot NJ. Investigating the cell biology of plant infection by the rice blast fungus Magnaporthe oryzae. Curr Opin Microbiol. 2016;34:147–153.
- Zhu XM, Li L, Wu M, et al. Current opinions on autophagy in pathogenicity of fungi. Virulence. 2019;10:481–489.
- Reggiori F, Tucker KA, Stromhaug PE, et al. The Atg1-Atg13 complex regulates Atg9 and Atg23 retrieval transport from the pre-autophagosomal structure. Dev Cell. 2004;6:79–90.
- Yin Z, Chen C, Yang J, et al. Histone acetyltransferase MoHat1 acetylates autophagy-related proteins MoAtg3 and MoAtg9 to orchestrate functional appressorium formation and pathogenicity in Magnaporthe oryzae. Autophagy. 2019;15:1234–1257.
- Foster AJ, Ryder LS, Kershaw MJ, et al. The role of glycerol in the pathogenic lifestyle of the rice blast fungus Magnaporthe oryzae. Environ Microbiol. 2017;19:1008–1016.
- Xie Z, Nair U, Klionsky DJ. Atg8 controls phagophore expansion during autophagosome formation. Mol Biol Cell. 2008;19:3290–3298.
- Yu ZQ, Ni T, Hong B, et al. Dual roles of Atg8-PE deconjugation by Atg4 in autophagy. Autophagy. 2012;8:883–892.
- Kraft C, Martens S. Mechanisms and regulation of autophagosome formation. Curr Opin Cell Biol. 2012;24:496–501.
- Schulze RJ, Krueger EW, Weller SG, et al. Direct lysosome-based autophagy of lipid droplets in hepatocytes. Proc Natl Acad Sci USA. 2020;117:32443–32452.
- Yin Z, Feng W, Chen C, et al. Shedding light on autophagy coordinating with cell wall integrity signaling to govern pathogenicity of Magnaporthe oryzae. Autophagy. 2020;16:900–916.
- Ward C, Martinez-Lopez N, Otten EG, et al. Autophagy, lipophagy and lysosomal lipid storage disorders. Biochim Biophys Acta. 2016;1864:269–284.
- Maeda Y, Oku M, Sakai Y. A defect of the vacuolar putative lipase Atg15 accelerates degradation of lipid droplets through lipolysis. Autophagy. 2015;11:1247–1258.
- Wang CW, Miao YH, Chang YS. Control of lipid droplet size in budding yeast requires the collaboration between Fld1 and Ldb16. J Cell Sci. 2014;127:1214–1228.
- Schmidt C, Ploier B, Koch B, et al. Analysis of yeast lipid droplet proteome and lipidome. Methods Cell Biol. 2013;116:15–37.
- Krahmer N, Mann M. Catching lipid droplet contacts by proteomics. Contact. 2019;2:2515256419859186.
- Chen YX, Cen K, Lu Y, et al. Nitrogen-starvation triggers cellular accumulation of triacylglycerol in Metarhizium robertsii. Fungal Biol. 2018;122:410–419.
- Huang W, Hong S, Tang G, et al. Unveiling the function and regulation control of the DUF3129 family proteins in fungal infection of hosts. Philos Trans R Soc Lond B Biol Sci. 2019;374:20180321.
- Sánchez-Wandelmer J, Kriegenburg F, Rohringer S, et al. Atg4 proteolytic activity can be inhibited by Atg1 phosphorylation. Nat Commun. 2017;8:295.
- Noda NN, Ohsumi Y, Inagaki F. Atg8-family interacting motif crucial for selective autophagy. FEBS Lett. 2010;584:1379–1385.
- Yamaguchi M, Noda NN, Nakatogawa H, et al. Autophagy-related protein 8 (Atg8) family interacting motif in Atg3 mediates the Atg3-Atg8 interaction and is crucial for the cytoplasm-to-vacuole targeting pathway. J Biol Chem. 2010;285:29599–29607.
- Nakatogawa H, Ohbayashi S, Sakoh-Nakatogawa M, et al. The autophagy-related protein kinase Atg1 interacts with the ubiquitin-like protein Atg8 via the Atg8 family interacting motif to facilitate autophagosome formation. J Biol Chem. 2012;287:28503–28507.
- Gao Q, Shang YF, Huang W, et al. Glycerol-3-phosphate acyltransferase contributes to triacylglycerol biosynthesis, lipid droplet formation, and host invasion in Metarhizium robertsii. Appl Environ Microbiol. 2013;79:7646–7653.
- Luo FF, Hong S, Chen B, et al. Unveiling of swainsonine biosynthesis via a multi-branched pathway in fungi. ACS Chem Biol. 2020;15:2476–2484.
- Huang W, Shang Y, Chen P, et al. Basic leucine zipper (bZIP) domain transcription factor MBZ1 regulates cell wall integrity, spore adherence, and virulence in Metarhizium robertsii. J Biol Chem. 2015;290:8218–8231.
- Huang A, Lu M, Ling E, et al. A M35 family metalloprotease is required for fungal virulence against insects by inactivating host prophenoloxidases and beyond. Virulence. 2020;11:222–237.
- Tang GR, Shang YF, Li SQ, et al. MrHex1 is required for Woronin body formation, fungal development and virulence in Metarhizium robertsii. J Fungi. 2020;6:172.
- Cen K, Li B, Lu YZ, et al. Divergent LysM effectors contribute to the virulence of Beauveria bassiana by evasion of insect immune defenses. PLoS Pathog. 2017;13:e1006604.
- Wang CS, St Leger RJ. Developmental and transcriptional responses to host and nonhost cuticles by the specific locust pathogen Metarhizium anisopliae var. acridum. Eukaryot Cell. 2005;4:937–947.
- Shang JM, Shang YF, Tang GR, et al. Identification of a key G-protein coupled receptor in mediating appressorium formation and fungal virulence against insects. Sci China Life Sci. 2021;64:466–477.