ABSTRACT
Mitophagy is a form of autophagy that selectively removes damaged mitochondria and attenuates mitochondrial-dependent apoptosis during viral infection, but how arboviruses balance mitophagy and apoptosis to facilitate persistent viral infection in insect vectors without causing evident fitness cost remains elusive. Here, we identified mitochondrial VDAC1 (voltage-dependent anion channel 1) that could be hijacked by nonstructural protein Pns11 of rice gall dwarf virus (RGDV), a plant nonenveloped double-stranded RNA virus, to synergistically activate pro-viral extensive mitophagy and limited apoptosis in leafhopper vectors. The direct target of fibrillar structures constructed by Pns11 with VDAC1 induced mitochondrial degeneration. Moreover, the degenerated mitochondria were recruited into Pns11-induced phagophores to initiate mitophagy via interaction of VDAC1 with Pns11 and an autophagy protein, ATG8. Such mitophagy mediated by Pns11 and VDAC1 required the classical PRKN/Parkin-PINK1 pathway. VDAC1 regulates apoptosis by controlling the release of apoptotic signaling molecules through its pore, while the anti-apoptotic protein GSN (gelsolin) could bind to VDAC1 pore. We demonstrated that the interaction of Pns11 with VDAC1 and gelsolin decreased VDAC1 expression but increased GSN expression, which prevented the extensive apoptotic response in virus-infected regions. Meanwhile, virus-induced mitophagy also effectively prevented extensive apoptotic response to decrease apoptosis-caused insect fitness cost. The subsequent fusion of virus-loaded mitophagosomes with lysosomes is prevented, and thus such mitophagosomes are exploited for persistent spread of virions within insect bodies. Our results reveal a new strategy for arboviruses to balance and exploit mitophagy and apoptosis, resulting in an optimal intracellular environment for persistent viral propagation in insect vectors.
Abbreviations: ATG: autophagy related; BNIP3: BCL2 interacting protein 3; CYCS/CytC: cytochrome c, somatic; dsGSN: double-stranded RNAs targeting GSN/gelsolin; dsGFP: double-stranded RNAs targeting green fluorescent protein; dsPRKN: double-stranded RNAs targeting PRKN; dsPns11: double-stranded RNAs targeting Pns11; dsRNA: double-stranded RNA; EC: epithelia cell; GST: glutathione S-transferase; LAMP1: lysosomal associated membrane protein 1; Mito: mitochondrion; Mmg: middle midgut; MP, mitophagosome; PG, phagophore. padp: post-first access to diseased plants; PINK1: PTEN induced kinase 1; RGDV: rice gall dwarf virus; SQSTM1: sequestosome 1; TOMM20: translocase of outer mitochondrial membrane 20; TUNEL: terminal deoxynucleotidyl transferase dUTP nick end labeling; VDAC1: voltage dependent anion channel 1.
Introduction
Many arthropod-borne viruses (arboviruses) with an obvious impact on agriculture or human health are persistently transmitted by insect vectors [Citation1,Citation2]. Remarkably, such viral transmission doses not cause evident insect fitness cost, suggesting that the immune tolerance employed by insect vectors has been modulated to ensure persistent viral infection in nature. Apoptosis and macroautophagy/autophagy are essential conserved degradative mechanisms, which participate in the removal of unwanted metabolites or foreign pathogens [Citation3,Citation4]. Numerous lines of research have confirmed that apoptosis and autophagy act synergistically rather than separately to modulate persistent viral infection [Citation5–8]. A recent study identified a phosphatidylethanolamine-binding protein that can be hijacked by the coat protein of tomato yellow leaf curl virus to simultaneously activate apoptosis and autophagy in whiteflies, which finally balances apoptosis and autophagy within the cell [Citation9,Citation10]. Therefore, an in-depth understanding of the balanced interplay between apoptosis and autophagy will help to illustrate how arboviruses escape the intracellular immunity of the vector.
Apoptosis is typically triggered by either an extrinsic death receptor or an intrinsic mitochondrial-dependent pathway [Citation11]. The initial event of mitochondrial-dependent apoptosis is the loss of mitochondrial membrane potential, which leads to the release of apoptosis-related factors associated with the mitochondrial membranes [Citation11]. The crosstalk between autophagy and mitochondrial-dependent apoptosis occurs in mitochondria. Mitophagy is a form of autophagy that is important to maintain the quality of mitochondria by selectively eliminating aged, dysfunctional, damaged, or excessive mitochondria [Citation12]. Several viruses are known to induce mitophagy to eliminate damaged mitochondria, which potentially attenuates mitochondrial-dependent apoptosis [Citation13–17]. The process of mitophagy is initiated by combining the signaling molecules on mitochondria and Atg8-family proteins on the phagophore membrane, thereby allowing the autophagic machinery to come into proximity with the mitochondria. The signaling molecules primarily include PRKN/Parkin-PINK1 (PTEN induced kinase 1) and mitophagy receptors, such as BNIP3 (BCL2 interacting protein 3) and BNIP3L/NIX (BCL2 interacting protein 3 like) [Citation18]. VDAC1/porin (voltage dependent anion channel 1), the key signaling molecule on mitochondria, plays important roles in the dual regulation of mitophagy and apoptosis [Citation4,Citation19,Citation20]. VDAC1 is a protein that is anchored in the outer mitochondrial membrane and forms a pore with a β-barrel structure that consists of 19 beta-strands [Citation20,Citation21]. VDAC1 regulates apoptosis by controlling the opening and closing of its pore to release the apoptotic signaling molecules to the cytosol [Citation20]. As one of the most notable participants in the apoptotic process, CYCS (cytochrome c, somatic) is released from the mitochondrion into the cytosol to initiate the activation of caspase cascade [Citation22]. The anti-apoptotic protein GSN (gelsolin) binds to VDAC1, which terminates the cytosolic transportation of apoptotic signaling molecules, including CYCS [Citation23–25]. Furthermore, VDAC1 also functions as a critical regulator of PRKN/Parkin-PINK1-mediated mitophagy by recruiting the autophagic receptor protein SQSTM1/p62 to the mitochondria [Citation26]. These results suggest that the dual regulation of mitophagy and apoptosis by VDAC1 is highly critical in maintaining mitochondrial quality control. In this study, we report that an important rice reovirus hijacks VDAC1 to simultaneously regulate apoptosis and mitophagy in leafhopper vectors, finally balancing apoptosis and mitophagy and maintaining persistent viral infection in insect vectors.
Rice gall dwarf virus (RGDV), which is transmitted by rice green leafhopper Recilia dorsalis in a persistent-propagative manner, can simultaneously regulate apoptosis and autophagy to facilitate persistent viral propagation [Citation21,Citation27,Citation28]. RGDV, an important rice reovirus, causes epidemic outbreaks and extensive losses in yields in Asian countries that grow rice [Citation21,Citation27,Citation28]. Infection with RGDV induces an apoptotic response in the alimentary canals of leafhoppers, including the degeneration of mitochondria and the collapse of mitochondrial membrane potential [Citation21]. The fibrillar structure formed by the nonstructural protein Pns11 of RGDV targets mitochondria by interacting with VDAC1 [Citation21]. This association of Pns11 with VDAC1 causes the degeneration of mitochondria, which finally triggers the mitochondrial-dependent apoptotic cascade [Citation21]. This apoptotic response promotes viral propagation in leafhopper vectors [Citation21]. However, RGDV-induced apoptotic response appears to be restricted to a low level to avoid serious damage to leafhopper vectors [Citation21]. Moreover, RGDV infection can also induce incomplete autophagy and hijack autophagosomes to traverse the membranes and tissue barriers of the midgut and salivary gland for viral propagation and dissemination [Citation28]. Pns11 alone can trigger incomplete autophagy to promote viral spread in insect vectors [Citation28]. Mechanistically, Pns11 induces the formation of initial autophagosomes by promoting ATG12–ATG5 conjugation and blocks autophagosome degradation by mediating the reduced expression of N-glycosylated LAMP1 (lysosomal associated membrane protein 1) on lysosomal membranes [Citation28]. To our knowledge, this is the first example of a virus that synchronously induces pro-viral autophagy and apoptosis. In this study, we showed that RGDV can trigger mitophagy to attenuate mitochondrial-dependent apoptosis, thus, promoting persistent viral infection in leafhopper vectors. The direct target of the fibrillar structures of Pns11 with VDAC1 induces the degeneration of mitochondria, which decreases VDAC1 expression but upregulates GSN expression to prevent extensive apoptosis in virus-infected regions. Moreover, VDAC1 on the degenerate mitochondrial membranes is the docking site of autophagosomes induced by Pns11, which finally results in the recruitment of degenerate mitochondria into the autophagosomes to induce mitophagy via the interaction of VDAC1 with Pns11 and ATG8. Thus, viral nonstructural protein Pns11 and VDAC1 synergistically activate the limited apoptosis and extensive mitophagy, resulting in an optimal intracellular environment to harbor RGDV. Our results reveal a function of VDAC1 in the dual regulation of the homeostasis of two different immune responses in leafhoppers for vector-arbovirus coexistence.
Results
RGDV infection induces mitophagy in insect vectors
Electron microscopy showed that RGDV infection induced the formation of bundles of virus-associated fibrillar structures in virus-infected midgut epithelium of viruliferous leafhopper vectors (). Such virus-associated fibrillar structures could bind to the outer membranes of intact mitochondria (). These mitochondria that were associated with fibrillar structures subsequently became degenerate, and their cristae were disrupted (). Such virus-associated degenerate mitochondria were close to and then directly sequestered in the autophagosome-like vesicles (). Immunoelectron microscopy showed that Pns11 antibody specifically recognized these mitochondria-associated fibrillar structures (). Furthermore, the fibrillar structures of Pns11 could distribute at the junction between mitochondria and autophagosome-like vesicles (). VDAC1 antibody densely reacted with the intact mitochondria in virus-free cells (). It also reacted with the degenerate mitochondria () and the mitochondria-associated fibrillar structures in virus-infected cells (). The amorphous materials within the double-membraned autophagosomes also reacted with the VDAC1 antibodies (), suggesting that these amorphous materials were the degenerate mitochondrial components. Furthermore, the Pns11 and ATG8 antibodies specifically reacted with the mitochondrial components-containing autophagosomes (). Thus, Pns11 potentially induced mitophagy by bridging autophagosomes and degenerate mitochondria. These data suggest that RGDV infection potentially triggers mitophagy to eliminate defective mitochondria.
Figure 1. Transmission electron micrographs (TEM) show that RGDV infection induced mitophagy in Recilia dorsalis. (A-C) Binding of virus-associated fibrillar structures to the outer membranes of intact mitochondria in virus-infected midgut epithelial cells in viruliferous leafhoppers. (D-F) Binding of virus-associated fibrillar structures to the outer membranes of degenerated mitochondria with indistinct cristae. (G-I) Virus-associated degenerate mitochondria close to or contained in the autophagosome-like vesicles. (J-M) Immunogold labeling of the RGDV Pns11 antibody showing specific reaction with mitochondria-associated fibrillar structures and the junction between mitochondria and autophagosome-like vesicles. (N) to (P) Immunogold labeling of the VDAC1 antibody showing reaction with the intact mitochondria in virus-free cells (N), with the degenerated mitochondria (O) and the mitochondria-associated fibrillar structures in virus-infected cells (P). (Q-S) Immunogold labeling of VDAC1 (Q), Pns11 (R) or ATG8 (S) antibody showing specific reaction with double-membraned autophagosomes. Panel i or ii is the enlargement of the boxed area in panel Q or R. Insert iii is the enlargement of the boxed area in panel S. Blue arrows indicate gold particles. AP, autophagosome. DM, double membrane. FS, fibrillar structure. Mito, mitochondrion. Bars: 500 nm (A – I, L – N, P – S) and 200 nm (J, K and O).
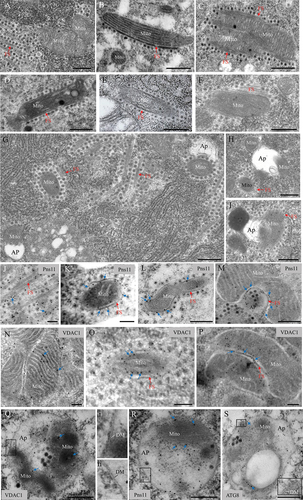
The hallmark of mitophagy is the recruitment of ATG8 from a diffuse localization to the mitochondria. Indeed, in virus-infected midgut epithelial cells, ATG8 redistributed into VDAC1-positive mitochondria (). There was a significant increase in the average number of ATG8 puncta per infected cell compared with those in the uninfected cells (). Furthermore, RGDV Pns11 appeared to form fibrillar or punctate structures, which co-localized with ATG8-, VDAC1- or TOMM20 (translocase of outer mitochondrial membrane 20)-positive puncta in virus-infected regions ( and S1). Taken together, our results demonstrate that autophagosomes induced by Pns11 are formed on the mitochondria and ultimately induce the selective degradation of mitochondria.
Figure 2. RGDV potentially activates PRKN/Parkin-PINK1-mediated mitophagy. (A, B) Immunofluorescence assays showing the colocalization of ATG8-VDAC1, Pns11-ATG8, and Pns11-VDAC1 in virus-infected midgut epithelial cells at 6-day padp. The intestinal tissues of the leafhoppers were fixed and immunostained with ATG8- or Pns11-FITC (green) and VDAC1- or ATG8-rhodamine (red). Immunostained intestinal tissues were then processed for immunofluorescence microscopy. The number of ATG8 puncta in the midgut epithelial cells of nonviruliferous and viruliferous leafhoppers intestinal tissues are shown in B. V−, nonviruliferous leafhoppers. V+, viruliferous leafhoppers. Bars: 5 μm. ****P < 0.0001. (C) The levels of expression of PRKN, ATG8, ATG5 and BNIP31 mRNA at 6-day padp in viruliferous and nonviruliferous leafhoppers as determined by RT-qPCR assays. The data are expressed as the means ± SD from 30 leafhoppers in RT-qPCR assays. The levels of expression were normalized against that of the EEF1A1/EF-1α transcript. The levels of transcripts tested in random nonviruliferous leafhoppers were normalized to 1. The data are representative of three biological replicates. Ns, not significant. **P < 0.01. (D) The levels of expression of the PRKN, ATG8, ATG5, SQSTM1, LAMP1, VDAC1, TOMM20 and P8 proteins in nonviruliferous or viruliferous leafhoppers as determined by western blot assays. The relative intensities of bands for the proteins are shown below. Equal amounts of protein were loaded on the Coomassie Brilliant Blue-stained gels. Data are representative of three biological replicates.
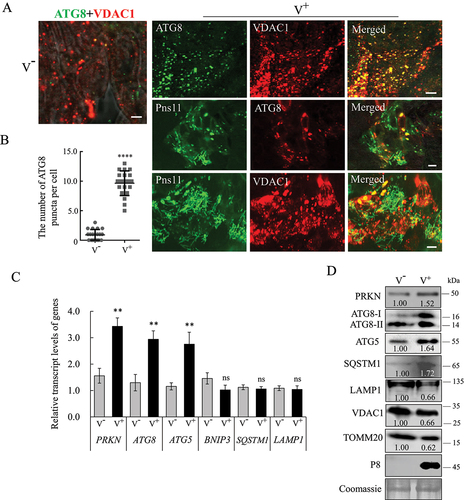
We then examined the expression of mitophagy-related proteins upon viral infection in insect vectors. At 6-day post-first access to diseased plants (padp), RT-qPCR assay showed that the levels of PRKN, ATG8 and ATG5 transcripts increased significantly, while those of BNIP3, SQSTM1 and LAMP1 remained unchanged upon viral infection (). Western blot assays further showed that the accumulation of PRKN, ATG8-II, ATG5 and SQSTM1 increased, while LAMP1 decreased upon viral infection (). Furthermore, the accumulation of mitochondrial proteins VDAC1 and TOMM20 also decreased (), confirming that the mitochondrial contents decreased following viral propagation. Taken together, these results confirm that RGDV activates mitophagy to eliminate damaged mitochondria.
VDAC1 binds directly to RGDV Pns11 and ATG8
VDAC1 is a critical substrate of PRKN in the regulation of mitophagy [Citation26], while RGDV Pns11 could induce autophagy or apoptosis by interaction with ATG5 or VDAC1 [Citation21,Citation28]. We then investigated how the Pns11-VDAC1 interaction activated mitophagy. Yeast two-hybrid (Y2H) and GST (glutathione S-transferase) affinity-isolation assays demonstrated that VDAC1 directly interacted with Pns11 and ATG8; however, Pns11 failed to interact with ATG8 ().
Figure 3. VDAC1 interacts with Pns11 and ATG8 in vitro. (A) Y2H assays that show the interaction of VDAC1 with Pns11 and ATG8. Transformants on SD/-Trp-Leu-Ade-His plates were labeled as follows: +, positive control (pGBKT7-53/pGADT7-T); –, negative control (pGBKT7-Lam/pGADT7-T). VDAC1+ Pns11, pGBKT7-VDAC1/pGADT7-Pns11; VDAC1+ ATG8, pGBKT7-VDAC1/pGADT7-ATG8; Pns11+ ATG8, pGBKT7-Pns11/pGADT7-ATG8. The yeast colonies were blue as shown in the β-galactosidase assay. (B) GST affinity-isolation assay showing VDAC1-Pns11 or VDAC-ATG8 interactions. VDAC1 fused with GST represents the bait; GST represents the control, and Pns11, ATG8 or PRKN fused with His represent the prey. The baits or GST control were incubated with cell lysate that expressed His-fused protein. Input and affinity-isolation samples were detected using antibodies against GST or His on western blot assays. (C) Immunofluorescence assay that shows the colocalization of Pns11-VDAC1, ATG8-VDAC1, or ATG8-Pns11-VDAC1 in Sf9 cells. The Sf9 cells were fixed at 48 hpi and immunolabeled with His-Alexa Fluor 488 (green), MYC-Alexa Fluor 555 (red), Flag-DyLight 488 (green) or MYC-Alexa Fluor 647 (blue). Bars: 10 μm.
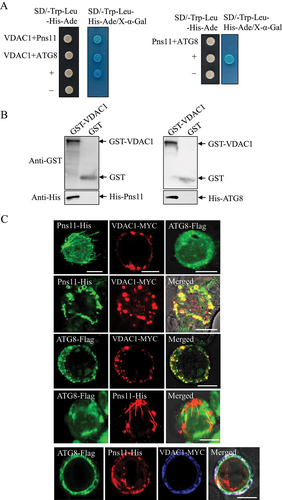
We then used an in vitro baculovirus expression system to investigate the relationship of Pns11, ATG8 and VDAC1 in Sf9 cells. When expressed individually, Pns11 formed the fibrillar structures, VDAC1 formed the punctate structures, and ATG8 remained diffused throughout the cytoplasm of Sf9 cells (). Co-expression led to the recruitment of ATG8 into the punctate structures of VDAC1 (). As expected, Pns11 did not co-localize with ATG8 (). The triple expression of ATG8, Pns11 and VDAC1 resulted in the colocalization of all three proteins in the punctate structures (). Thus, the bridging of phagophores and degenerate mitochondria via the fibrillar structures of Pns11 was mediated by the Pns11-VDAC1-ATG8 interaction.
RGDV-induced mitophagy could effectively remove damaged mitochondria
We then investigated whether the mitophagy induced by RGDV could effectively remove damaged mitochondria. Third instar nymphs of leafhoppers were fed on diseased rice plants for 2 days, which was followed by microinjection with double-stranded RNAs (dsRNAs) that targeted PRKN or GFP (dsPRKN or dsGFP) gene. At 6-day padp, RT-qPCR and western blot assays indicated that the knockdown of PRKN expression decreased viral accumulation in viruliferous leafhoppers (). Western blot assay showed the decreased accumulation of PRKN and ATG8-II, while the increased accumulation of VDAC1 in dsPRKN-treated viruliferous leafhoppers (). Immunofluorescence microscopy showed that the knockdown of PRKN expression effectively inhibited the recruitment of ATG8 to VDAC1-positive mitochondria in virus-infected midgut epithelial cells (). Moreover, the microinjection of VDAC1 antibody into viruliferous leafhoppers also effectively inhibited the recruitment of Pns11 or ATG8 to the VDAC1-positive mitochondria in virus-infected midgut epithelial cells (). Thus, RGDV-induced mitophagy could selectively remove damaged mitochondria and facilitate viral propagation in insect vectors. Furthermore, these results also reveal that VDAC1 is involved in RGDV-induced mitophagy by serving as the docking site for Pns11-induced autophagosomes.
Figure 4. RGDV-induced mitophagy facilitates viral propagation in insect vectors. (A, B) Effect of the knockdown of PRKN gene expression on RGDV infection and ATG8 expression at 6-day padp. RT-qPCR assays show the levels of expression of PRKN and P8 mRNA in dsGFP- or dsPRKN-treated leafhoppers in A. The data are expressed as the means ± SD from 30 leafhoppers in RT-qPCR assays. Western blot assays show the levels of expression of ATG8, PRKN and VDAC1, as well as the accumulation of RGDV, in dsGFP- or dsPRKN-treated leafhoppers in B. Relative intensities of the bands for PRKN, P8 and VDAC1 are shown below. Equal amounts of protein were loaded on the Coomassie Brilliant Blue-stained gels. The data are representative of three biological replicates. ***P < 0.001. (C, D) Effect of the knockdown of PRKN gene expression on ATG8-VDAC1 colocalization in virus-infected midgut epithelial cells as determined by an immunofluorescence assay. The number of ATG8-VDAC1 colocalizing puncta per midgut epithelial cell of dsGFP- or dsPRKN-treated leafhoppers is shown in D. ****P < 0.0001. (E, F) Effect on the colocalization of Pns11 with VDAC1 or ATG8 in virus-infected midgut epithelial cells following treatment with the VDAC1 antibody. Intestinal tissues of the leafhoppers were fixed and immunostained with Pns11- or ATG8-FITC (green), and VDAC1-rhodamine (red). Immunostained intestine tissues were then processed for immunofluorescence microscopy. The number of Pns11-VDAC1 or ATG8-VDAC1 colocalizing puncta per midgut epithelial cell of pre-immune or VDAC1 antibody-treated leafhoppers is shown in F. Bars: 5 μm.
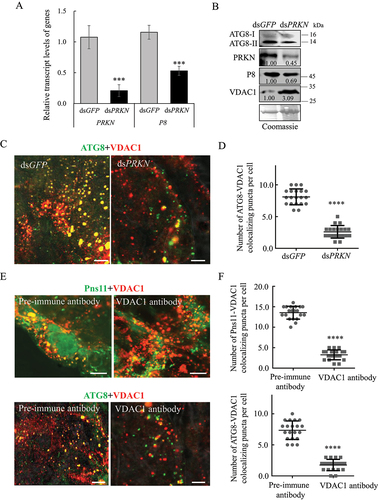
RGDV-induced mitophagy effectively prevents apoptosis to maintain persistent infection
We have shown that RGDV-induced apoptotic response was restricted to a low level in infected insect tissues to avoid serious damage to leafhopper vectors [Citation21]. Because RGDV infection synchronously induced mitophagy and mitochondrial-dependent apoptosis [Citation21], these two mitochondrial immune responses were then compared. Immunofluorescence assays at 6-day padp showed that the ATG8 puncta were extensively distributed along with virus-infected regions; however, the terminal deoxynucleotidyl transferase dUTP nick end labeling (TUNEL) signals were restricted to the limited areas of virus-infected regions in midgut epithelial cells (). Thus, RGDV infection can simultaneously trigger extensive autophagy and limited apoptosis. We then explored whether mitophagy induced by RGDV was responsible for preventing mitochondrial-dependent apoptosis. The well-established apoptosis marker cleaved-CASP2 (caspase 2) and the activity of CASP3 (caspase 3) were upregulated upon RGDV infection at 6-day padp (). However, the suppression of virus-induced mitophagy by the knockdown of PRKN expression significantly enhanced the distribution of TUNEL signals and the accumulation of cleaved-CASP2 in virus-infected regions (). These results, together with the result that the accumulation of VDAC1 increased following the knockdown of PRKN expression (), revealed that virus-induced mitophagy effectively removed damaged mitochondria and prevented apoptosis. In addition, the survival rates calculated based on > 100 individuals were 33.0% and 69.0% for leafhoppers treated with dsPRKN or dsGFP, respectively (), suggesting that virus-induced mitophagy effectively decreased the adverse effect of mitochondrial-dependent apoptosis upon its vectors. Together, these results suggest that virus-induced mitophagy effectively prevents extensive apoptosis to cause serious damage to leafhopper vectors, finally maintaining persistent viral infection in insect vectors.
Figure 5. RGDV-induced mitophagy inhibits the apoptotic response for persistent viral infection in leafhopper vectors. (A) The TUNEL combined with immunofluorescence assay showing mitophagy and limited apoptosis synchronously induced by RGDV infection at 6-day padp. The intestinal tissues of leafhoppers were fixed, incubated with rTdT incubation buffer, and mixed with ATG8-rhodamine (red), and RGDV-647 (blue). The immunostained intestinal tissues were then processed for immunofluorescence microscopy analysis. EC, epithelial cell. V−, nonviruliferous leafhoppers. V+, viruliferous leafhoppers. Bars: 50 μm. (B, C) RGDV infection induces the occurrence of apoptosis as determined by cleaved CASP2 and CASP3 activity in nonviruliferous or viruliferous leafhoppers. Cleaved CASP2 in viruliferous leafhoppers in B was determined by western blot assays. Equal amounts of protein were loaded on the Coomassie Brilliant Blue-stained gels. The data are representative of three biological replicates. CASP3 activity in nonviruliferous or viruliferous leafhoppers is shown in C. Each group of approximately 10 mg of leafhoppers were analyzed to determine the activity. The data are presented as the mean ± SD. *P < 0.05. (D, E) Effect of the knockdown of PRKN gene expression on the apoptotic response in dsGFP- or dsPRKN-treated viruliferous leafhoppers at 6-day padp as determined by TUNEL assays. The intestinal tissues of leafhoppers were fixed and incubated with rTdT incubation buffer, which was mixed with RGDV-647 (blue). Immunostained intestinal tissues were then processed for immunofluorescence microscopic analysis. The percentage of TUNEL-positive cells in midguts of dsGFP- or dsPRKN-treated leafhoppers is shown in E. ****P < 0.0001. Mmg, middle midgut. Bars: 50 μm. (F) Effect of the knockdown of PRKN gene expression on cleaved CASP2 in dsGFP- or dsPRKN-treated viruliferous leafhoppers as determined by western blot assays. Equal amounts of protein were loaded on the Coomassie Brilliant Blue-stained gels. The data are representative of three biological replicates. (G) Effect of the knockdown of PRKN gene expression on the survival of viruliferous leafhoppers at 10-day padp. The means ± SD from three biological replicates are presented. **P < 0.01.
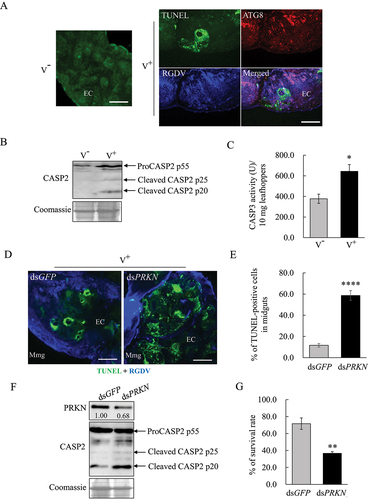
Viral infection upregulates the expression of GSN but inhibits that of VDAC1 to avoid extensive apoptosis
The binding of fibrillar structures of RGDV Pns11 with VDAC1 induced apoptotic responses to facilitate viral propagation in insect vectors [Citation21]. The apoptosis inhibitor GSN could interact with VDAC1 to block mitochondrial membrane potential loss [Citation23–25]. Y2H and GST affinity-isolation assays showed that there were interactions among Pns11, VDAC1 and GSN (). RT-qPCR and western blot assays showed that viral infection significantly increased GSN expression but decreased VDAC1 expression at mRNA and protein levels (. Immunofluorescence microscopy showed that Pns11, GSN and VDAC1 colocalized in virus-infected midgut epithelial cells (). RT-qPCR assay showed that the treatment of dsVDAC1 effectively decreased the mRNA expression levels of VDAC1 and P8 in viruliferous leafhoppers (). Western blot assays showed that the knockdown of VDAC1 expression significantly decreased the accumulation of ATG8-II, cleaved CASP2 and P8 (). Accordingly, the distribution of TUNEL signals in the intestinal tissues of dsVDAC1-treated insects was also reduced (). In contrast, RT-qPCR assay showed that dsGSN treatment effectively increased the mRNA expression levels of GSN and P8 in viruliferous insects (). Western blot assays showed that the knockdown of GSN expression significantly increased the cleavage of CASP2, CASP3 activity and P8 accumulation (). Accordingly, the TUNEL signal distribution in the intestinal tissues of dsGSN-treated insects also increased (). Although increased apoptosis and viral infection were observed in dsGSN-treated viruliferous insects, their survival rates decreased accordingly (). Thus, VDAC1 and GSN exerted antagonistic effects on the regulation of apoptosis during viral propagation. It can be concluded that viral infection significantly upregulates the expression of GSN but inhibits that of VDAC1 to avoid stimulating extensive apoptotic responses, which finally restricts apoptosis in the limited areas of viral-infected regions to maintain persistent viral infection in insect vectors. Furthermore, the results also suggest that the presence of VDAC1 on degenerate mitochondrial membranes is positively required for virus-induced mitophagy in viruliferous insects.
Figure 6. Interaction of GSN with Pns11 and VDAC1 in vitro and in vivo. (A) Y2H assays showing the interaction of GSN with Pns11 or VDAC1. Transformants on the SD/-Trp-Leu-Ade-His plates were labeled as follows: GSN+VDAC1, pGBKT7-GSN/pGADT7-VDAC1; GSN+Pns11, pGBKT7-GSN/pGADT7-Pns11; +, positive control, (pGBKT7-53/pGADT7-T) and –, negative control (pGBKT7-Lam/pGADT7-T). The yeast colonies were grown in SD/-Trp-Leu-His-Ade medium and were blue as shown in the β-galactosidase assay. (B) GST affinity-isolation assays showing the interaction of GSN with Pns11 or VDAC1. Pns11 or VDAC1 fused with GST represent the bait; GST represents the control, and GSN fused with His represents the prey. The baits or the GST control were incubated with cell lysate that expressed His-fused protein. Input and affinity-isolation samples were detected using antibodies against GST or His by western blot assays. (C, D) Effect of RGDV infection on the levels of expression of GSN and VDAC1 at 6-day padp as determined by RT-qPCR and western blot assays. The RT-qPCR assay shows the levels of expression of mRNA of GSN in nonviruliferous or viruliferous leafhoppers. The data are expressed as the means ± SD from 30 leafhoppers in RT-qPCR assays. The levels of expression were normalized against that of the EEF1A1/EF-1α transcript. The levels of transcripts tested in random nonviruliferous leafhoppers were normalized to 1. The levels of expression of GSN and VDAC1 in nonviruliferous or viruliferous leafhoppers were determined by western blot assays. The relative intensities of bands for these proteins are shown below. Equal amounts of protein were loaded on the Coomassie Brilliant Blue-stained gels. The data are representative of three biological replicates. V−, nonviruliferous leafhoppers. V+, viruliferous leafhoppers. *P < 0.05, **P < 0.01. (E) Immunofluorescence microscopy that shows the colocalization of Pns11, GSN and VDAC1 in the midgut epithelial cells of viruliferous leafhoppers in 12-day padp. The intestinal tissues of leafhoppers were fixed and immunostained with GSN- or Pns11-FITC (green), and VDAC1- or GSN-rhodamine (red). Bars: 5 μm.
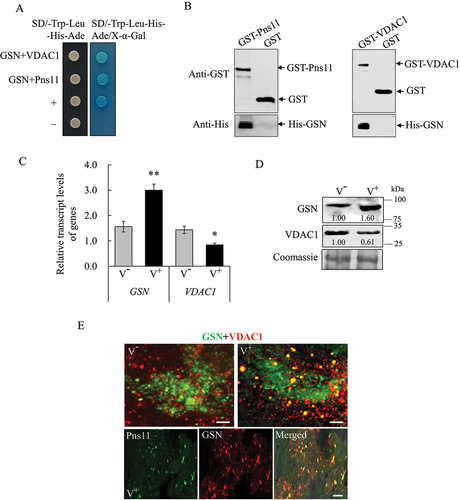
Figure 7. VDAC1 and GSN regulate RGDV-induced apoptosis. (A, B) Effect of the knockdown of VDAC1 on the levels of expression of VDAC1, ATG8 and P8, as well as the cleavage of CASP2, at 6-day padp in viruliferous leafhoppers. RT-qPCR assays show the levels of expression of the mRNA of VDAC1 and P8 in viruliferous leafhoppers in A. The levels of expression of VDAC1, ATG8, P8 and CASP2 in dsGFP- or dsVDAC1-treated viruliferous leafhoppers were determined by western blot assays. Relative intensities of the bands for these proteins are shown below. Equal amounts of protein were loaded on the Coomassie Brilliant Blue-stained gels. The data represent three biological replicates. *P < 0.05, **P < 0.01. (C, D) TUNEL assays showing the levels of apoptosis in the midgut epithelial cells of dsGFP-, dsVDAC1- or dsGSN-treated viruliferous leafhoppers at 6-day padp. The intestinal tissues of leafhoppers were fixed, incubated with rTdT incubation buffer, and then processed for immunofluorescence microscopy analysis. The percentage of TUNEL-positive cells in midguts of dsGFP-, dsVDAC1- or dsGSN-treated leafhoppers is shown in D. EC, epithelial cell. Mmg, middle midgut. Bars: 50 μm. (E, F) Effect of the knockdown of GSN gene on the levels of expression of GSN and P8, as well as the cleavage of CASP2, at 6-day padp in viruliferous leafhoppers. RT-qPCR assays show the levels of expression of GSN and P8 mRNA in viruliferous leafhoppers in E. The data are presented as the mean ± SD. Western blot assays show the levels of expression of GSN, P8 and CASP2 in dsGFP- or dsGSN-treated viruliferous leafhoppers in F. The relative intensities of the bands for these proteins are shown below. Equal amounts of protein were loaded on the Coomassie Brilliant Blue-stained gels. The data are representative of three biological replicates. (G) CASP3 activity in dsGFP-, dsVDAC1- or dsGSN-treated viruliferous leafhoppers. Each group of approximately 10 mg of leafhoppers was analyzed to determine the activity. The data are presented as the mean ± SD. ***P < 0.001. (H) Effect of the knockdown of the expression of GSN on the survival of viruliferous leafhoppers at 10-day padp. The means ± SD from three biological replicates are presented. *P < 0.05.
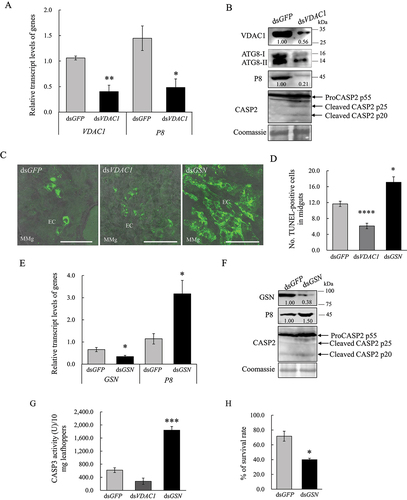
Pns11 inherently mediates mitophagy and apoptosis
We had previously shown that the expression of Pns11 in Sf9 cells had the inherent ability to induce autophagy and apoptosis [Citation21,Citation28]. To determine whether Pns11 could simultaneously induce autophagy and apoptosis, we expressed Pns11 in Sf9 using a baculovirus vector system. The expression of Pns11 in Sf9 cells increased ATG8-II and cleaved CASP2 accumulation but decreased VDAC1 accumulation (). Similarly, the microinjection of purified Pns11 into the leafhoppers also increased ATG8-II and cleaved CASP2 accumulation but decreased VDAC1 accumulation (). Furthermore, the knockdown of Pns11 expression in viruliferous leafhoppers by microinjection with dsRNAs that targeted Pns11 (dsPns11) gene decreased ATG8-II and cleaved CASP2 accumulation but increased VDAC1 accumulation (). Taken together, our results reveal that RGDV Pns11 can simultaneously induce autophagy and apoptosis but decrease the accumulation of mitochondria, suggesting that Pns11 alone inherently triggers mitophagy to remove mitochondria.
Figure 8. Pns11 inherently induces mitophagy and apoptotic responses in Sf9 cells and leafhopper bodies. (A) Effect of Pns11-His expression on the levels of expression of ATG8 and VDAC1, as well as the cleavage of CASP2, in Sf9 cells as determined by western blot assays. (B) Effect of injection with Pns11-His on the levels of expression of ATG8 and VDAC1, as well as the cleavage of CASP2, in leafhoppers as determined by western blot assays. The relative intensities of bands for these proteins are shown below. Equal amounts of protein were loaded on the Coomassie Brilliant Blue-stained gels. The data are representative of three biological replicates. (C, D) Effect of the knockdown of Pns11 gene expression on the levels of expression of Pns11, VDAC1 and ATG8, as well as the cleavage of CASP2, in viruliferous leafhoppers at 6-day padp. RT-qPCR assays show the levels of expression of Pns11 mRNA in viruliferous leafhoppers in C. The data are presented as the mean ± SD. Western blot assays show the levels of expression of Pns11, VDAC1 and CASP2 in dsGFP- or dsPns11-treated viruliferous leafhoppers in D. The relative intensities of the bands for these proteins are shown below. Equal amounts of protein were loaded on the Coomassie Brilliant Blue-stained gels. The data are representative of three biological replicates.
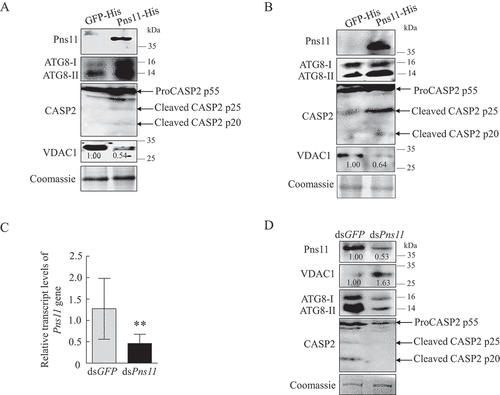
Discussion
Long-term association between arboviruses and their insect vectors results in evolutionary trade-offs that maintain a balance between the fitness cost of viral infection of insects and persistent viral transmission by the insect. Persistent infection by arboviruses in insect vectors induces immune homeostasis to modulate vector-virus coexistence [Citation29–31]. In general, viral infection triggers immune responses, such as autophagy, apoptosis and small interfering RNA antiviral pathway as antiviral defense mechanisms to control the excessive accumulation of viruses in insect vectors [Citation32–35]. How arboviruses modulate the homeostasis of these immune responses during viral persistent infection in insect vectors remains poorly understood.
RGDV simultaneously activates pro-viral apoptosis and autophagy in leafhopper vectors [Citation21,Citation27,Citation28]. We demonstrate that RGDV virions are engulfed within Pns11-induced autophagosomes, which are modified to evade fusion with lysosomes for degradation and exploited for spread virions within insect vectors [Citation28]. Moreover, RGDV Pns11 also triggers the mitochondrial-dependent apoptosis to benefit viral propagation, but such apoptosis has been restricted to a low level to avoid decreasing the fitness of vectors [Citation21]. Thus, RGDV simultaneously activates extensive autophagy and limited apoptosis in leafhopper vectors, which results in an optimal intracellular environment for RGDV propagation. Mitophagy is a form of autophagy for damaged mitochondrial clearance [Citation12], and we show that RGDV-induced autophagosomes could recruit the damaged mitochondria to form mitophagosomes for preventing mitochondrial-dependent apoptosis (). Thus, we reveal a new strategy for arboviruses to balance the different immune responses to promote persistent viral infection in insect vectors.
Figure 9. Proposed model of extensive RGDV-induced mitophagy attenuating mitochondria-dependent apoptosis for persistent viral infection in leafhopper vectors. In RGDV-infected cells, the target of fibrillar structures formed by viral nonstructural protein Pns11 with VDAC1 induces mitochondrial degeneration. The interaction of Pns11-VDAC1-GSN regulates apoptosis by controlling the release of apoptotic signaling molecules such as CYCS through VDAC1 porin. Meanwhile, Pns11 directly interacts with ATG12–ATG5 to induce an autophagic response. The degenerate mitochondria are recruited into Pns11-induced autophagosomes to form the mitophagosome via interaction of VDAC1 with Pns11 and ATG8. Therefore, Pns11 and VDAC1 synergistically activate mitophagy for the elimination of damaged mitochondria, potentially attenuating mitochondria-dependent apoptosis and achieving immune homeostasis in leafhopper vectors. Mito, mitochondrion. MP, mitophagosome. PG, phagophore.
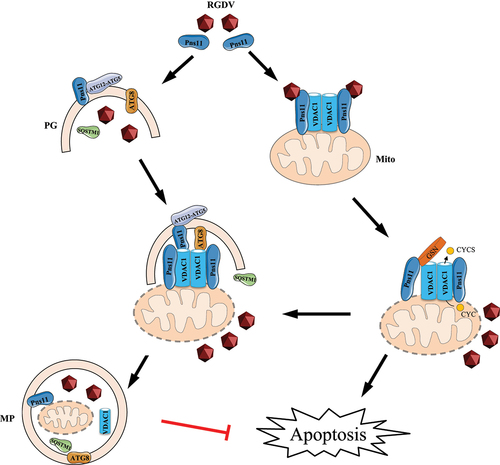
We identify VDAC1 that can be hijacked by Pns11 of RGDV to simultaneously activate apoptosis and mitophagy in leafhopper. VDAC1 is a critical component of the mitochondrial permeability transition pore, and thus, regulates apoptosis by controlling the opening and closing of its pore to release apoptotic signaling molecules, such as CYCS, to activate the caspase cascade [Citation20,Citation22]. The apoptosis inhibitor GSN binds to VDAC1 pore to block the release of CYCS and mitochondrial membrane potential loss [Citation23–25]. The direct target of fibrillar structures of Pns11 with VDAC1 induces mitochondrial degeneration and finally causes apoptotic response in leafhopper [Citation21]. We demonstrate that the fibrillar structures of Pns11 directly bind to VDAC1 and GSN, which decreases VDAC1 expression but increases GSN expression. The knockdown of VDAC1 expression inhibits apoptotic response; however, the knockdown of GSN expression promotes the apoptotic response. Based on these data, we deduce that the decreased expression of VDAC1 but increased expression of GSN during viral infection potentially blocks the release of apoptotic signaling molecules from mitochondria to cytosol, finally preventing the extensive apoptotic response ().
Mitophagy that selectively eliminates damaged mitochondria is an essential mitochondrial quality control mechanism12. Here, we further show that VDAC1 on the degenerate mitochondrial membranes is the docking site of Pns11-induced autophagosomes via the interaction of VDAC1 with Pns11 and ATG8, which finally results in the sequestration of degenerate mitochondria by autophagosomes to form mitophagosomes (). The subsequent fusion of mitophagosomes with lysosomes is prevented by Pns11 by mediating the reduced expression of N-glycosylated LAMP1 on the lysosomal membrane [Citation28]. Therefore, virus-induced incomplete mitophagy contributes to viral propagation by providing autophagic membranes to accommodate and spread virions and inhibiting the extensive apoptotic response. Previous studies showed that VDAC1 can be ubiquitinated by PRKN and then recruit the autophagic adaptor protein SQSTM1 to the mitochondria to induce mitophagy [Citation26]. Typically, SQSTM1 binds both ATG8 and ubiquitinated VDAC1 by PRKN, and thus, the VDAC1-SQSTM1-ATG8 interaction mediates the sequestration of mitochondria by autophagosomes. For the first time, we identify that VDAC1 can directly bind to the autophagy initiation protein ATG8, thus, revealing a new role of VDAC1 in triggering mitophagy by the direct recruitment of mitochondria by ATG8 on the phagophore membrane.
We also determine that the expression of Pns11 alone induces mitochondrial-dependent apoptosis and autophagy. Furthermore, Pns11 alone increases the accumulation of ATG8-II and cleaved CASP2 but decreased that of VDAC1, suggesting that Pns11 can synergistically balance the induction of apoptosis and mitophagy. Taken together, our results reveal that VDAC1 can be hijacked by RGDV Pns11 to balance two different immune responses to maintain persistent viral infection in insect vectors. To the best of our knowledge, this is the first evidence that shows that a viral protein can simultaneously induce pro-viral apoptosis and mitophagy to facilitate persistent viral infection in insect vectors.
Viral-induced mitophagy is closely associated with the suppression of innate immune response. For example, the matrix protein of human parainfluenza virus 3 induces mitophagy independent of the PRKN/Parkin-PINK1 pathway to suppress the interferon response [Citation36]. Recently, we reported that the nonstructural protein P7-1 of southern rice black streaked dwarf virus induces mitophagy mediated by BINP3 to suppress apoptosis in planthopper vectors [Citation17], which is different from current results. These results suggest that activation of different mitophagic responses to attenuate the innate immune response is a universal phenomenon in response to virus infection in eukaryote organisms.
Materials and methods
Insects, viruses, and antibodies
Nonviruliferous individuals of the R. dorsalis leafhopper were collected from Fujian Province in south of China and propagated for several generations in the laboratory. Rice plants infected with RGDV were initially collected from Guangdong Province and propagated by transmission by R. dorsalis under greenhouse conditions.
Rabbit polyclonal antibodies against RGDV P8, VDAC1, ATG8, PRKN, ATG5, SQSTM1, LAMP1 and GSN antigens were prepared by Genscript Biotech Corporation (Nanjing, China) as approved by the Science Technology Department of Jiangsu Province of China. Rabbit polyclonal antibody against CASP2/caspase2 was prepared by ABclonal Technology Corporation (Wuhan, China) as approved by the Science Technology Department of Hubei Province of China. Rabbit polyclonal antibody against Pns11 was provided by Dr. Toshihiro Omura (National Agricultural Research Center, Tsukuba, Japan). Rabbit polyclonal antibody against TOMM20 was purchased from Abcam (ab186735). Mouse monoclonal antibodies against 6× His tag and GST were purchased from TransGen Biotech (HT501 and HT601). The His tag antibody conjugated to Alexa Fluor 488 (His-Alexa Fluor 488); the MYC tag antibody conjugated to Alexa Fluor 555 or 647 (MYC-Alexa Fluor 555 or 647), and the Flag tag antibody conjugated to DyLight 488 (Flag-DyLight 488) were purchased from Thermo Fisher Scientific (MA1-135-A488, MA1-980-A555, MA1-980-A647 and MA1-91,878-D488).
Transmission electron microscopy
Intestines dissected from leafhoppers were fixed, dehydrated, and embedded at 14-day padp as previously described [Citation21]. The ultrathin sections were examined under a transmission electron microscope (TEM) (H-7650; Hitachi, Tokyo, Japan). Immunoelectron microscopy was conducted using ultrathin sections that were immunolabeled with Pns11-, VDAC1-, or ATG8-specific IgG as the primary antibody and were then treated with goat anti-rabbit IgG conjugated with 15-nm diameter gold particles (Abcam, ab27236) as the secondary antibody.
Immunofluorescence microscopy
To determine the localization of proteins in the intestines of leafhoppers, the intestines were first dissected, fixed in 4% (v:v) paraformaldehyde in PBS (Coolaber Science & Technology, PM5090) for 2 h, and then permeabilized in 0.2% Triton X-100 (Vetec, V900502) for 1 h. The intestines were then immunostained with ATG8-, Pns11- or GSN-specific IgG conjugated to FITC (ATG8-FITC, Pns11-FITC or GSN-FITC), ATG8-, VDAC1- or TOMM20-specific IgG conjugated to rhodamine (ATG8-rhodamine, VDAC1-rhodamine or TOMM20-rhodamine), as well as RGDV virus-specific IgG conjugated to Alexa Fluor 647 (RGDV-647). The immunostained intestines were then processed for immunofluorescence microscopy.
RT-qPCR and western blot assays
To relatively quantify the expression of genes, the total RNA was extracted from 30 leafhoppers. RT-qPCR assays were performed using the 2 × RealStar Fast SYBR qPCR Mix (High ROX) (GenStar, A303). The EEF1A/EF-1α transcript of R. dorsalis served as the internal reference, and the relative levels of gene expression were calculated using the 2−ΔΔCT method.
For western blot assays, P8-, Pns11-, PRKN-, ATG8-, ATG5-, SQSTM1-, LAMP1-, VDAC1- TOMM20-, CASP2- and GSN-specific IgG served as the primary antibody, and goat anti-rabbit IgG-peroxidase served as the secondary antibody. GST- and 6× His tag-specific IgG served as the primary antibody, and goat anti-mouse IgG-peroxidase served as the secondary antibody (Sangon Biotech Co., Ltd., D110058). ImageJ software (NIH, Bethesda, MD, USA) was used to quantify the intensities of bands in the western blot assays.
Yeast two-hybrid system
The Matchmaker Gold Yeast-two-hybrid system was used to examine the protein-protein interaction. The ORFs of VDAC1, Pns11 and GSN were separately cloned into the pGBKT7 vector (Clontech, 630, 489) as the bait plasmids. The ORFs of Pns11, ATG8 and VDAC1 were separately cloned into the pGADT7 vector (Clontech, K1612-1) as prey plasmids, respectively. The prey and bait plasmids were then co-transformed into yeast strain of AH109. The β-galactosidase activity was detected on SD/-Ade-His-Leu-Trp (Clontech, 630, 428) medium containing X-α-Gal (20 μg/mL; INALCO SPA, 1758–0200).
GST affinity-isolation and competitive binding assays
A GST affinity-isolation assay was conducted as previously described [Citation21]. The full-length ORFs of VDAC1 and Pns11 were separately cloned into the pGEX-3x vector preserved in our laboratory to construct plasmids that expressed the GST fusion protein as baits (GST-VDAC1 and GST-Pns11, respectively). The full-length ORFs of Pns11, ATG8, PRKN and GSN were separately cloned into the pEASY-Blunt E1 Expression Vector (Transgen Biotech, CE111-01) to construct the plasmids that expressed the His fusion protein as preys (His-Pns11, -ATG8, -PRKN and -GSN). The recombinant proteins fused with a GST tag, as well as GST, were expressed separately in Escherichia coli strain BL21. The lysates were then incubated with glutathione-Sepharose beads (GE Healthcare, 17–0756-01) and subsequently incubated with the recombinant proteins fused with the His tag. Finally, the eluants were analyzed by western blot assays using GST-tag and His-tag antibodies.
Baculovirus expression in Sf9 cells
Pns11, VDAC1 or ATG8 were expressed in a baculovirus according to the manufacturer’s instructions (Thermo Fisher Scientific, 11,827,011). Briefly, the ORF of Pns11, VDAC1 or ATG8 was amplified with reverse primers that contained the coding sequences of the His-tag, MYC-tag or Flag-tag and specific forward primers to construct Pns11-His, VDAC1-MYC or ATG-Flag. The purified PCR products were cloned into the pDEST8 vector (Thermo Fisher Scientific, 11,827,011) to construct recombinant baculoviruses that contained Pns11-His, VDAC1-MYC or ATG-Flag. Recombinant bacmids were generated by transforming E. coli DH10Bac (Thermo Fisher Scientific, 10,359,016) with the recombinant baculovirus.
The purified recombinant bacmids were then transfected into Sf9 cells using Cellfectin II (Thermo Fisher Scientific, 10,362,100) according to the manufacturer’s instructions. At 48 h post infection (hpi), the cells were fixed in 4% (v:v) paraformaldehyde in PBS for 30 min and then permeabilized in 0.2% Triton X-100 for 15 min. The cells were then immunostained with antibodies against His-Alexa Fluor 488, MYC-Alexa Fluor 555 or 647, and Flag-DyLight 488. The cells were then observed directly under a fluorescence microscope.
Effect of synthesized dsRNAs on viral infection, mitophagy or apoptosis
The T7 RNA polymerase promoter with the sequence 5’-ATTCTCTAGAAGCTTAATACGACTCACTATAGGG-3’ was added to the forward primers and reverse primers at the 5’ terminal to amplify a region of approximately 500–700 bp of the PRKN, VDAC1, GSN or Pns11 gene. A T7 RiboMAX Express RNAi System (Promega, P1700) was used to synthesize in vitro dsRNAs that targeted PRKN (dsPRKN), GFP (dsGFP), VDAC1 (dsVDAC1), GSN (dsGSN) or Pns11 (dsPns11). Nonviruliferous second-instar nymphs were fed on diseased rice plants for 2 days and then microinjected with dsPRKN, dsGFP, dsVDAC1, dsGSN or dsPns11 (approximately 200 ng/insect) at the intersegment region of the thorax using a Nanoject II Auto-Nanoliter Injector (Spring). The injected leafhoppers were then transferred to healthy rice seedlings for recovery.
At 6-day padp, 10 each of these leafhoppers were collected to comprise one group. At least five groups were tested for their relative levels of expression of PRKN, P8, VDAC1, GSN or Pns11 using RT-qPCR assays. The levels of expression were normalized against the level of EEF1A1/EF-1α transcript. The levels of gene transcripts in a random group of dsGFP-treated viruliferous leafhoppers were normalized to 1. Approximately 20 of those leafhoppers were collected to determine the level of protein expression using western blot assays.
TUNEL assay
A One Step TUNEL Apoptosis Assay Kit (Beyotime Biotechnology, C1086) was used to detect apoptotic bodies in R. dorsalis leafhoppers. At 14-day padp, the intestines of R. dorsalis were dissected and fixed in 4% (v:v) paraformaldehyde in PBS for 2 h and then permeabilized in 0.2% Triton X-100 for 1 h. The intestines were incubated with rTdT incubation buffer, which was then mixed with ATG8-rhodamine or RGDV-647 at 37°C for 2 h. The immunostained intestines were then processed for immunofluorescence microscopy.
Enzyme activity assay of CASP3
A Caspase-3 Activity Assay Kit (Beyotime Biotechnology, C1116) was used to assay the activity of leafhopper CASP3. Groups of 10 adult leafhoppers were homogenized on ice, resuspended in 100 µL Cell Lysis Buffer, and incubated on ice for 5 min. After centrifugation, the supernatant was transferred to a fresh chilled tube on ice for immediate assay. The protein concentration was measured and adjusted to 1–3 mg/mL for each assay. The caspase reaction mix for each reaction was prepared according to the manufacturer’s instructions. The absorbance at 405 nm of supernatant was measured using a microplate reader (TECAN, Männedorf, Switzerland).
Statistical analysis
All the quantitative data presented in the text and figures were analyzed with two-tailed t-tests in GraphPad Prism 7 (GraphPad Software, San Diego, CA, USA).
Supplemental Material
Download MS Word (3.6 MB)Acknowledgments
We are grateful to Dr. Toshihiro Omura (National Agricultural Research Center, Tsukuba, Japan) for providing the antibodies against intact RGDV particles.
Disclosure statement
The authors declare no competing interests.
Supplementary material
Supplemental data for this article can be accessed online at https://doi.org/10.1080/15548627.2022.2150001
Data availability statement
The authors declare that all the data supporting the findings of this study are available in the manuscript.
Additional information
Funding
References
- Chen Q, Wei T. Cell biology during infection of plant viruses in insect vectors and plant hosts. Mol Plant Microbe Interact. 2020;33(1):18–25.
- Jia D, Chen Q, Mao Q, et al. Vector mediated transmission of persistently transmitted plant viruses. Curr Opin Virol. 2018;28:127–132.
- Chiramel AI, Brady NR, Bartenschlager R. Divergent roles of autophagy in virus infection. Cells. 2013;2(1):83–104.
- Bloemberg D, Quadrilatero J. Autophagy, apoptosis, and mitochondria: molecular integration and physiological relevance in skeletal muscle. Am J Physiol Cell Physiol. 2019;317(1):C111–C130.
- Wang XR, Shao Y, Wang C, et al. Effects of heat stress on virus transmission and virus-mediated apoptosis in whitefly Bemisia tabaci. Arch Insect Biochem Physiol. 2022;110(1):e21857.
- Wang XR, Wang C, Ban FX, et al. Apoptosis in a whitefly vector activated by a begomovirus enhances viral transmission. mSystems. 2020;5(5):e00433–20.
- Wang S, Guo H, Ge F, et al. Apoptotic neurodegeneration in whitefly promotes the spread of TYLCV. Elife. 2020;9:e56168.
- Wang LL, Wang XR, Wei XM, et al. The autophagy pathway participates in resistance to tomato yellow leaf curl virus infection in whiteflies. Autophagy. 2016;12(9):1560–1574.
- Wang S, Guo H, Ge F, et al. Apoptosis and autophagy coordinately shape vector tolerance to arbovirus infection. Autophagy. 2022;18(9):2256–2258.
- Wang S, Guo H, Zhu-Salzman K, et al. PEBP balances apoptosis and autophagy in whitefly upon arbovirus infection. Nat Commun. 2022;13(1):846.
- Sinha K, Das J, Pal PB, et al. Oxidative stress: the mitochondria-dependent and mitochondria-independent pathways of apoptosis. Arch Toxicol. 2013;87(7):1157–1180.
- Sun K, Jing X, Guo J, et al. Mitophagy in degenerative joint diseases. Autophagy. 2021;17(9):2082–2092.
- Xia M, Meng G, Jiang A, et al. Mitophagy switches cell death from apoptosis to necrosis in NSCLC cells treated with oncolytic measles virus. Oncotarget. 2014;5(11):3907–3918.
- Zaghloul HAH, Hice R, Bideshi DK, et al. Mitochondrial and innate immunity transcriptomes from spodoptera frugiperda larvae infected with the spodoptera frugiperda ascovirus. J Virol. 2020;94(9):e01985–19.
- Zhou Y, Zhang Y, Moorman JP, et al. Viral (hepatitis C virus, hepatitis B virus, HIV) persistence and immune homeostasis. Immunology. 2014;143(3):319–330.
- Zhu L, Mou C, Yang X, et al. Mitophagy in TGEV infection counteracts oxidative stress and apoptosis. Oncotarget. 2016;7(19):27122–27141.
- Liang Q, Wan J, Liu H, et al. A plant nonenveloped double-stranded RNA virus activates and co-opts BNIP3-mediated mitophagy to promote persistent infection in its insect vector. Autophagy. 2022;1–16. doi:10.1080/15548627.2022.2091904.
- Turkieh A, El Masri Y, Pinet F, et al. Mitophagy regulation following myocardial infarction. Cells. 2022;11(2):199.
- Roca-Agujetas V, de Dios C, Lestón L, et al. Recent insights into the mitochondrial role in autophagy and its regulation by oxidative stress. Oxid Med Cell Longev. 2019;2019:3809308.
- Shoshan-Barmatz V, De Pinto V, Zweckstetter M, et al. VDAC, a multi-functional mitochondrial protein regulating cell life and death. Mol Aspects Med. 2010;31(3):227–285.
- Chen Q, Zheng L, Mao Q, et al. Fibrillar structures induced by a plant reovirus target mitochondria to activate typical apoptotic response and promote viral infection in insect vectors. PLoS Pathog. 2019;15(1):e1007510.
- Shoshan-Barmatz V, Shteinfer-Kuzmine A, Verma A. VDAC1 at the intersection of cell metabolism, apoptosis, and diseases. Biomolecules. 2020;10(11):1485.
- Kusano H, Shimizu S, Koya R, et al. Human gelsolin prevents apoptosis by inhibiting apoptotic mitochondrial changes via closing VDAC. Oncogene. 2000 Oct 5;19(42):4807–4814.
- Qiao H, McMillan J. Gelsolin segment 5 inhibits HIV-induced T-cell apoptosis via Vpr-binding to VDAC. FEBS Lett. 2007 Feb 6;581(3):535–540.
- Koya RC, Fujita H, Shimizu S, et al. Gelsolin inhibits apoptosis by blocking mitochondrial membrane potential loss and cytochrome c release. J Biol Chem. 2000;275(20):15343–15349.
- Sun Y, Vashisht AA, Tchieu J, et al. Voltage-dependent anion channels (VDACs) recruit Parkin to defective mitochondria to promote mitochondrial autophagy. J Biol Chem. 2012;287(48):40652–40660.
- Chen Y, Chen Q, Li M, et al. Autophagy pathway induced by a plant virus facilitates viral spread and transmission by its insect vector. PLoS Pathog. 2017;13(11):e1006727.
- Jia D, Liang Q, Liu H, et al. A nonstructural protein encoded by a rice reovirus induces an incomplete autophagy to promote viral spread in insect vectors. PLoS Pathog. 2022;18(5):e1010506.
- Brackney DE, Correa MA, Cozens DW. The impact of autophagy on arbovirus infection of mosquito cells. PLoS Negl Trop Dis. 2020;14(5):e0007754.
- Mehrbod P, Ande SR, Alizadeh J, et al. The roles of apoptosis, autophagy and unfolded protein response in arbovirus, influenza virus, and HIV infections. Virulence. 2019;10(1):376–413.
- Gonzalez-Gonzalez A, Wayne ML. Immunopathology and immune homeostasis during viral infection in insects. Adv Virus Res. 2020;107:285–314.
- Lan H, Wang H, Chen Q, et al. Small interfering RNA pathway modulates persistent infection of a plant virus in its insect vector. Sci Rep. 2016;6:20699.
- Lan H, Chen H, Liu Y, et al. Small interfering RNA pathway modulates initial viral infection in midgut epithelium of insect after ingestion of virus. J Virol. 2016;90(2):917–929.
- Liu Y, Mao Q, Lan H, et al. Investigation of alimentary canal ultrastructure following knockdown of the Dicer-2 gene in planthoppers reveals the potential pathogenicity of southern rice black streaked dwarf virus to its insect vector. Virus Res. 2018;244:117–127.
- Myles KM, Wiley MR, Morazzani EM, et al. Alphavirus-derived small RNAs modulate pathogenesis in disease vector mosquitoes. Proc Natl Acad Sci U S A. 2008;105(50):19938–19943.
- Ding B, Zhang L, Li Z, et al. The matrix protein of human parainfluenza virus type 3 induces mitophagy that suppresses interferon responses. Cell Host Microbe. 2017;21(4):538–547.e4.