Abstract
Background
Ingestion of agricultural organophosphorus insecticides is a significant cause of death in rural Asia. Patients often show acute respiratory failure and/or delayed, unexplained signs of neuromuscular paralysis, sometimes diagnosed as “Intermediate Syndrome”. We tested the hypothesis that omethoate and cyclohexanol, circulating metabolites of one agricultural formulation, cause muscle weakness and paralysis.
Methods
Acetylcholinesterase activity of insecticide components and metabolites was measured using purified enzyme from eel electroplaque or muscle homogenates. Mechanomyographic recording of pelvic limb responses to nerve stimulation was made in anaesthetized pigs and isometric force was recorded from isolated nerve-muscle preparations from mice. Omethoate and cyclohexanol were administered intravenously or added to physiological saline bathing isolated muscle. We also assessed the effect of MgSO4 and cooling on neuromuscular function.
Results
Omethoate caused tetanic fade in pig muscles and long-lasting contractions of the motor innervation zone in mouse muscle. Both effects were mitigated, either by i.v. administration of MgSO4 in vivo or by adding 5 mM Mg2+ to the medium bathing isolated preparations. Combination of omethoate and cyclohexanol initially potentiated muscle contractions but then rapidly blocked them. Cyclohexanol alone caused fade and block of muscle contractions in pigs and in isolated preparations. Similar effects were observed ex vivo with cyclohexanone and xylene. Cyclohexanol-induced neuromuscular block was temperature-sensitive and rapidly reversible.
Conclusions
The data indicate a crucial role for organophosphorus and solvent metabolites in muscle weakness following ingestion of agricultural OP insecticide formulations. The metabolites omethoate and cyclohexanol acted conjointly to impair neuromuscular function but their effects were mitigated by elevating extracellular Mg2+ and decreasing core temperature, respectively. Clinical studies of MgSO4 therapy and targeted temperature management in insecticide-poisoned patients are required to determine whether they may be effective adjuncts to treatment.
Introduction
Attempted suicide or self-harm by consumption of agricultural organophosphorus (OP) insecticides has led to millions of deaths since the advent of the agricultural Green Revolution in the 1960s, especially among communities living in rural Asia, and continues to kill tens of thousands each year [Citation1–3]. The toxicity of OP compounds is also a general matter of global concern, underscored by recent instances of malicious uses of potent nerve agents [Citation4,Citation5].
At a molecular level, OP pesticides kill insects by inhibiting acetylcholinesterase (AChE) activity, which normally terminates the action of neurotransmitter at cholinergic synapses, including neuromuscular junctions [Citation6–10]. When humans swallow these insecticides, they may experience symptoms and show signs of severe OP poisoning, including pulmonary edema, acute respiratory failure, abnormal weakness in proximal muscles (myasthenia), and neuromuscular synaptic dysfunction [Citation11–13]. Mitigation of autonomic signs may occur following prompt administration of the muscarinic antagonist atropine [Citation14]. However, muscarinic antagonists have no substantive effect on nicotinic acetylcholine receptors (nAChR) and are therefore of no benefit to patients who develop skeletal muscle paralysis, which may develop within minutes to more than 72 hrs after hospital presentation [Citation15,Citation16]. A common occurrence is the delayed-onset myasthenic syndrome referred to clinically as “Intermediate Syndrome” (IMS) [Citation17–20], which affects about 10% to more than 50% of patients in some case series [Citation15,Citation21].
In both early and delayed onset OP-induced respiratory failure, clinical signs of muscle weakness are notable in respiratory and neck musculature. Potential treatments that promote reactivation of AChE or reduce the sensitivity of nAChR have been unsuccessful, indicating that inhibition of AChE is not a sufficient explanation [Citation22]. Electromyographic recordings indicate failure of neuromuscular transmission [Citation23,Citation24]. However, there is marked variation in severity, with some patients showing an impaired function of neuromuscular junctions (NMJs) detectable only with neurophysiological analysis, others developing mild muscle fatigue, and others developing profound weakness requiring mechanical ventilation for several weeks [Citation20]. Acute myasthenia and IMS, therefore, lie on a broad spectrum of onset and severity of neuromuscular dysfunction in these patients [Citation15,Citation16]. Prognosis and outcomes are better for those presenting with acute or early paralysis compared to the late or intermediate syndrome. Following the onset of mechanical ventilation, fatality in severe cases of either acute or delayed respiratory failure is 20–50% [Citation14,Citation18,Citation21,Citation23].
The mechanisms of OP insecticide-induced respiratory failure are poorly understood. The clinical presentation correlates with the level and duration of inhibition of AChE but not all cases of OP insecticide toxicity lead to muscle weakness or paralysis, implicating other factors [Citation2,Citation25]. Of note, IMS has been recorded in patients after ingesting agricultural OP insecticides that include quantities of solvents equal to the OP active ingredient but not after occupational exposure to insecticides diluted in water [Citation17,Citation23,Citation26].
Previously, we obtained evidence utilising an anaesthetised pig model that ingestion (gavage) of the agricultural insecticide dimethoate EC40 gave rise to progressive loss of muscle force in response to nerve stimulation, leading to almost complete paralysis within 6–12 h, thus mimicking early myasthenic signs of OP insecticide ingestion in humans [Citation27]. Gavage of purified dimethoate alone did not produce these effects, implicating the principal organic solvent (cyclohexanone) as a proximate cause. However, measurements of plasma concentrations indicated, as expected, that cyclohexanone was metabolised to cyclohexanol [Citation28,Citation29]. The serum concentration of cyclohexanol progressively increased while cyclohexanone concentration declined. Both compounds reached steady state levels about 6–12 h after ingestion. Thus, rising levels and progressive serum accumulation of cyclohexanol were associated more strongly with the onset and progression of paralysis than falling levels of cyclohexanone [Citation27]. Concurrently, however, the OP ingredient dimethoate was metabolised to the more potent anticholinesterase, omethoate, whose plasma levels also increased steeply and persistently during that study.
In the present study, we tested directly the hypothesis that muscle weakness following agricultural insecticide consumption is caused by the combined effects of its main constituent metabolites, omethoate and cyclohexanol. We first examined for, and ruled out, any significant effects of relevant concentrations of cyclohexanol on AChE enzymic activity. We then used force recordings to measure the effects of intravenous (i.v.) administration of omethoate and cyclohexanol on muscle strength in anaesthetised pigs, or after adding those compounds to solutions bathing isolated mouse muscles. Our findings provide new insight into the causes and mechanisms of paralysis following ingestion of insecticide which have eluded explanation for more than 40 years. We also found effective mitigating treatments that may have clinical utility.
Materials and methods
Acetylcholinesterase (AChE) assay
Quantitative measurements of AChE enzymic activity were made using a modified Ellman method [Citation30,Citation31]. Stock solutions were acetylthiocholine iodide (ATH; 1.7397 mg ml−1 in PBS), used as the enzymic substrate; 5,5′-dithio-bis(2-nitrobenzoic acid) (DTNB, 0.7872 mg ml−1 in standard 0.2 M phosphate-buffered saline, PBS, pH 7.4). Purified eel AChE solution (Sigma-Aldrich, 0.06 U ml−1 in PBS) or muscle homogenates from 2 to 5 mice (1 g tissue blended in 8 ml PBS). Briefly, 10–50 µl of either purified eel AChE or muscle homogenate were aliquoted into each well of a 96 –well plate, then an aliquot of test compound (e.g., omethoate) was added to the desired final concentration and the volume made up to 200 µl with PBS, then incubated for 20 min at room temperature. DTNB (50 µl from stock) was added, followed by 50 µl of ATH substrate, also from stock. Measurement of absorption at 450 nm began immediately and was monitored over time using an MRX microplate reader (Dynex Technologies, Chantilly, USA). Thiocholine production in the test wells was expressed in units of nmoles per minute, calibrated with reference to the absorbance change using glutathione as the DTNB reactant, over the range of concentrations that gave a linear response [Citation32]. Some isolated mouse muscles were stained for AChE activity, using a modified Karnovsky-Roots method, as described in detail previously [Citation33,Citation34].
Animals and tissues
Experiments were performed on anaesthetised Landrace cross male pigs weighing approximately 15 kg and 1.2–1.5 months of age. Isolated nerve-muscle preparations were made from adult (1–8-month-old) mice of C57Bl6 or CD01 strains. Some experiments were performed using mice of the C57Bl-WldS strain that were crossbred to double homozygosity with the transgenic line thy1.2YFP16 [Citation35,Citation36]. All animals were obtained from stocks bred and maintained in University of Edinburgh animal care facilities.
Pigs
The pig studies were conducted after approval by the Institutional (Roslin) Animal Welfare and Ethical Review Board and licensing by the UK Home Office (PPL: PCF8 D45D0). They were housed, fed, watered and exposed to light: dark cycles in accordance with Home Office requirements. Pre-anaesthetic medication in all cases was: ketamine (Nareketan; Vetoquinol UK Ltd.) 5 mg kg−1; midazolam (Hypnovel; Roche) 0.5 mg kg−1; morphine (Morphine sulfate; Martindale Pharma) 0.5 mg kg−1; and medetomidine (Sedator; Dechra Veterinary Products) mixed in the same syringe and injected intramuscularly. Once profound sedation was achieved, after approximately 10 min, anaesthesia was induced with isoflurane (Isoflo; Zoetis UK Ltd) carried in a 1:2 O2:N2O mixture delivered by a Hall’s mask attached to a Bain breathing system. When the jaw was relaxed, the laryngeal mucosa was desensitized with a lidocaine (lidocaine hydrochloride; Hameln Pharmaceuticals Ltd) spray and the trachea intubated with a cuffed endotracheal tube. Anaesthesia was maintained thereafter until the end of the experiments using isoflurane (end-tidal concentrations of 1.5–2.0%) in an O2: medical air mixture producing inspired O2 fractions of 0.5. A medetomidine/morphine/ketamine mixture equivalent to doses of 5, 100 and 600 µg kg−1 h−1 was also infused i.v. Depth of anaesthesia was based on standard evaluations including the absence of cranial nerve and other reflexes, cardiovascular variables and bispectral index. The lungs were ventilated mechanically and variables (tidal volume and rate) adjusted to ensure end-tidal concentrations of CO2 of 5.2–5.4 kPa. These values and end-tidal isoflurane concentrations were recorded throughout anaesthesia while core and rectal temperatures were monitored using thermistor probes. Normothermia was preserved by operating in ambient temperatures of 22–24 °C, with the pigs enveloped in a hot air blowing system (Bair Hugger; Arizant UK Limited, UK). The temperature of the skin overlying m. tibialis cranialis was not monitored but the exposed limb was covered with an insulating layer of polythene bubble-wrap. Ringer's lactate solution was infused i.v. at 5 mL kg−1 h−1 throughout anaesthesia. Cooling was induced by applying ice packs to the torso and in one pig by rectal infusion of room-temperature physiological saline while monitoring core temperature with thermistor probes. Test compounds were administered via an i.v. catheter. Omethoate was given as a 20 or 40 mg kg−1 bolus (dissolved in 1 mL kg−1 of saline) over 2 min. followed by a continuous infusion of 0.08 mg kg−1 min−1 (0.1 mL kg−1 min−1 of an 0.8 mg mL−1 solution in saline). A cyclohexanol solution (3.5% v:v in saline) was infused at rates to give 3.6–14.3 mL kg−1 over 30 min, equivalent to the required doses of 125–500 mg kg−1 of cyclohexanone. Venous blood samples were collected at 30 min intervals into 4 mL K2EDTA tubes, immediately centrifuged (2500×g for 5 min) and the plasma aliquoted into duplicate 2 mL storage tubes which were stored at −80 °C. Batches dispatched on dry ice to Anatune Ltd (Cambridge, UK) for analysis by selected ion flow tube mass spectrometry (SIFT-MS). A lethal dose (100 mg kg−1) of pentobarbital was administered i.v. on completion of all experiments.
Mice
For isolation of nerve-muscle preparations, mice were sacrificed in accordance with UK Home Office Schedule 1, by anaesthetic overdose followed by cervical dislocation. Nerve-muscle preparations of flexor digitorum brevis (FDB), hemidiaphragm, or triangularis sterni (TS) [Citation34,Citation37] were promptly dissected and maintained in mammalian physiological saline (MPS) with the following composition (mM): Na+ (158); K+ (5); Ca2+ (2); Mg2+ (1); Cl− (169); glucose (5); HEPES (5); pH 7.2–7.4. Solutions were bubbled with air for at least 20 min. Most experiments were conducted at room temperature (19–25 °C). Dimethoate, omethoate, cyclohexanone, cyclohexanol or xylene (all Sigma-Aldrich) were added directly from aqueous stock solutions to bathing solutions to give the concentrations required. Aliquots (10–100 µl) were either pipetted directly and thoroughly mixed into MPS in the recording chamber to the required concentration, or solutions containing the required final concentration in 50 ml volumes were rapidly exchanged with the solution in the recording chamber (volume approximately 10 ml) using coupled back-to-back 20–50 ml syringes connected to ports at opposite ends of the chamber. Baffles built into the chamber facilitated laminar flow and complete solution exchange occurred within 5–20 s.
Mechanomyography
Mechanomyographic (MMG) recordings from pig extensor muscles in vivo were made using a bespoke limb fixation device which allowed the twitch responses of the pelvic limb digital extensor muscles to be measured by force-displacement transduction [Citation38]. The force transducer was connected to a Powerlab 26 T interface (AD Instruments, Oxford, UK). Tension data were acquired and analysed using Labchart 7 or 8 (AD Instruments) software running on an Apple Macbook Pro laptop or desktop Hewlett Packard PC computers. Neuromuscular “fade” and block [Citation39,Citation40]. were assessed from responses to supramaximal train-of-four (TOF) stimulation of the common peroneal nerve with pulses up to 0.6 ms in duration and nominally 10 V in magnitude [Citation38], or during tetanic stimulation delivered at 50 Hz for 1–2 s. Stimulus pulses were delivered via a DS2 constant voltage stimulator (Digitimer, Welwyn Garden City, UK).
Imaging muscle contraction
Muscle contractions were imaged directly in isolated mouse triangularis sterni (TS) muscles. The TS muscle is advantageous for direct ex-vivo imaging since it comprises a flat sheet, 1–2 muscle fibres thick, with intercostal innervation clearly discernible by bright field or fluorescence microscopy, with or without phase/interference contrast enhancement [Citation37,Citation41]. The isolated muscles were mounted on the stage of an Olympus BX50WI upright fluorescence microscope fitted with 10×–40× water dipping objectives. Muscle nerves were stimulated with supramaximal (10 V, 0.2 ms) pulses via a glass suction pipette connected to a Digitimer DS2 stimulator triggered by a 4030 Programmer (Digitimer, Welwyn Garden City, UK). Either brightfield or fluorescence images were acquired with a Qimaging OptiMOS 2.1 MP camera (Photometrics, Marlow, UK) at up to 100 frame per second (fps) during stimulation, using Micromanager public domain software[Citation42] (downloadable from https://micro-manager.org/). Images were analysed using tools embedded in ImageJ (downloadable from https://imagej.nih.gov/ij/) or FiJi (downloadable from https://fiji.sc/).
Muscle force measurements from isolated muscles
Force measurements were made from isolated mouse tibial nerve-FDB muscle preparations, pinned by their distal tendons to the base of a Sylgard-lined chamber. The proximal tendon was connected by 6/0 silk suture to an MLT0202 force transducer (AD Instruments, Oxford, UK). The preparations were bathed in 10 ml of MPS and the tibial nerve was aspirated into a glass suction electrode. Nerve stimuli (0.1–0.2 ms duration, nominally up to 10 V) were delivered via a DS2 stimulator (Digitimer, Welwyn Garden City, UK) triggered by the Powerlab 26 T interface. Direct stimulation (100 V, 1 ms pulses) was delivered through a pair of silver wires positioned on either side of the muscle. Force recordings were captured and digitised at 1 kHz and analysed using Labchart 7 software running on a Macintosh iMac or Hewlett-Packard PCs. In some experiments, bath temperature was regulated by passing the bathing solution (with or without added cyclohexanol) through a 2HCB inline heater and TC344B heating control unit (Warner Instruments, Harvard Apparatus, Cambridge, UK). Bathing solution temperature was monitored by a thermocouple probe (MLT1400, AD Instruments, Oxford, UK) whose tip was placed adjacent to the muscle and connected via an ML312 pod to a Powerlab 26 T interface (both AD Instruments) and Apple iMac running Labchart 7 software (AD Instruments), which was also used simultaneously to program stimulation and recording neuromuscular contractile responses. TOF twitch and tetanic (20–50 Hz) responses were analysed in Labchart software. For measurement of tetanic “aftercontractions” we used a customised algorithm implemented using Microsoft Excel spreadsheet functions. Briefly, the peak tetanic response following administration of omethoate was normalised to the peak tetanic response in control solution (before adding omethoate), the area under the curve (AUC) of the control trace was subtracted, and the residual AUC was then expressed as a percentage of the total area under the curve in the experimental trace. Thus, for example, if there were no aftercontraction the residual AUC would have been 0% of total AUC, while an aftercontraction of equivalent magnitude and duration to that associated with the tetanic response would yield an aftercontraction AUC value of 50% of total area.
Drugs and toxins
Omethoate and dimethoate were obtained from Toronto Research Chemicals (Abcam, Cambridge, UK) or Sigma-Aldrich (Irvine, UK) and either made up as stock solutions dissolved in aqueous media or in dimethyl sulfoxide (DMSO). Cyclohexanol, cyclohexanol xylene, d-tubocurarine and neostigmine were obtained from Sigma-Adrich. Required concentrations were made up by dilution in MPS.
Statistics and graphics
Data were analysed and graphed using Excel (Microsoft, Reading, UK) and Prism 7 for Mac (Graphad, La Jolla, USA). Student’s t-tests or ANOVA were used respectively to assess the significance of mean differences of continuous parametric data between two groups (paired or unpaired as appropriate) or multiple groups. When Gaussian distributions in the test and control data were assumed we applied Tukey’s, Sidak’s, Dunnett’s or Bonferroni post-hoc evaluations, as appropriate, in those instances when ANOVA indicated significant differences between groups. Mann–Whitney U-test, Wilcoxon signed-rank tests, or Kruskal–Wallis tests were used to assess non-parametric data or data for which Gaussian distributions were not assumed. Dunn’s post-hoc test for comparison of means was used in cases where Kruskal–Wallis tests indicated a significant difference between multiple groups. Linear and non-linear regression fits and coefficients were calculated using functions built into Graphpad Prism. Power calculations were not generally made except for pig experiments, where the number of animals used to validate an apparent effect size of at least 20% was based on variance observed in pilot experiments. For ex vivo and in vitro experiments, the number of replicates was chosen arbitrarily and significance estimated from calculations of the relevant statistics at α = 0.05 .
Results
Omethoate inhibits AChE activity but cyclohexanol does not
We first established the relative sensitivity of AChE to omethoate, cyclohexanol and their parent compounds dimethoate and cyclohexanone in order to establish whether the effects of solvent could be attributed to any additive anticholinesterase effects. The following data appear to rule out this hypothesis.
As expected, the OP metabolite omethoate inhibited the activity of purified AChE from eel electroplaque with an approximate IC50 of 3 µM (), in agreement with previous reports [Citation43,Citation44]. At concentrations above 50 µM, omethoate almost completely abolished AChE activity. The parent compound, dimethoate was a much weaker inhibitor, with an apparent IC50 three orders of magnitude greater (). By contrast, neither cyclohexanol nor its parent compound cyclohexanone substantially inhibited purified eel AChE at concentrations that we previously observed following OP insecticide gavage in vivo (). However, AChE activity of purified enzyme was significantly reduced in 20 mM cyclohexanol, by 19.6 ± 4.6% (mean ± S.E.M. of triplicate measurements in 9 experiments; p = 0.0019, t-test; ).
Figure 1. Omethoate inhibits AChE more strongly than dimethoate, cyclohexanone or cyclohexanol. (A–D) Activity of eel AChE is inhibited by (A) omethoate (IC50 about 3 µM) but much less so by (B) dimethoate (IC50 about 5 mM), (C) cyclohexanol (IC50 about 25 mM) and (D) cyclohexanone (IC50 about 40 mM, about 40-fold greater than plasma concentration measured after insecticide gavage [Citation25]). Red arrows indicate the peak circulating concentrations of these compounds following insecticide gavage [Citation27]. (E,F) Cyclohexanol (5 mM) was a weak inhibitor of AChE activity in (E) diaphragm muscle extracts and (F) FDB muscle extracts. Bars show mean ± SEM. Surprisingly, cyclohexanol reduced the inhibitory effect of 10 µM omethoate by 50% but had no effect at 100 µM omethoate. (G–I) Omethoate, but not cyclohexanol, blocked histochemical staining for AChE at motor endplates in mouse FDB muscle. (G) control endplates showing strong histochemical AChE staining (arrows); (H) pre-incubation in cyclohexanol (20 mM) did not block AChE staining at NMJs while (I) pre-incubation in 100 µM omethoate blocked all AChE staining.
![Figure 1. Omethoate inhibits AChE more strongly than dimethoate, cyclohexanone or cyclohexanol. (A–D) Activity of eel AChE is inhibited by (A) omethoate (IC50 about 3 µM) but much less so by (B) dimethoate (IC50 about 5 mM), (C) cyclohexanol (IC50 about 25 mM) and (D) cyclohexanone (IC50 about 40 mM, about 40-fold greater than plasma concentration measured after insecticide gavage [Citation25]). Red arrows indicate the peak circulating concentrations of these compounds following insecticide gavage [Citation27]. (E,F) Cyclohexanol (5 mM) was a weak inhibitor of AChE activity in (E) diaphragm muscle extracts and (F) FDB muscle extracts. Bars show mean ± SEM. Surprisingly, cyclohexanol reduced the inhibitory effect of 10 µM omethoate by 50% but had no effect at 100 µM omethoate. (G–I) Omethoate, but not cyclohexanol, blocked histochemical staining for AChE at motor endplates in mouse FDB muscle. (G) control endplates showing strong histochemical AChE staining (arrows); (H) pre-incubation in cyclohexanol (20 mM) did not block AChE staining at NMJs while (I) pre-incubation in 100 µM omethoate blocked all AChE staining.](/cms/asset/aad59f8c-5861-4705-b1c4-eb1f1cf674e3/ictx_a_1916519_f0001_c.jpg)
As in the eel preparations, omethoate (100 µM) abolished enzymic activity of AChE in both diaphragm and FDB muscle homogenates. In diaphragm homogenates, a small but significant decrease in AChE activity was found with 5 mM cyclohexanol () but we did not find this concentration to be effective in FDB muscle homogenates. In these, AChE activity was about half that of the purified eel enzyme incubations and muscle homogenates were less sensitive to omethoate (). For instance, eel AChE activity was reduced about 10-fold in 10 µM omethoate but by only about 50% in mouse FDB muscle homogenates. However, when omethoate (10 µM) and cyclohexanol (15 mM) were added together to FDB homogenates, AChE inhibition was actually less than with omethoate alone by about 20%, (p = 0.036, unpaired t-test, n = 3 preparations of 4–6 homogenised FDB muscles; ). But when AChE was completely inhibited with omethoate (100 µM) there was no evidence of mitigation by cyclohexanol.
Omethoate also inhibited histochemical staining of mouse NMJs for AChE. Cyclohexanol (20 mM) had no discernible effect on histochemical staining of NMJs for AChE, however (), consistent with the measurements of AChE inhibition based on biochemical measurements.
Taken together, these measurements confirm that micromolar concentrations of omethoate strongly inhibit AChE but millimolar concentrations of cyclohexanone or cyclohexanol only modestly reduce AChE activity.
Omethoate impairs neuromuscular responses to tetanic stimulation in vivo
Next, we measured the functional effects of i.v. administration of omethoate. We attached a force transducer to one pelvic limb of eleven anaesthetised pigs and used this transducer to obtain MMG recordings of contractions of pelvic limb muscles, in response to stimulation of the common peroneal nerve. We continuously monitored the MMG responses of muscles to train-of-four (TOF) stimulation (2 Hz, every 20–30 s). Tetanic responses were tested in nine of the animals by brief stimulation (50 Hz, 0.5–2 s duration) delivered at 15–30 min intervals. Omethoate was infused i.v. in 11 animals at doses of 2–4 mg kg−1 h−1 , following bolus i.v. injections of 20–40 mg kg−1. This resulted in plasma concentrations of omethoate ranging from 87.3 to 817 µM, overlapping the range we measured following dimethoate EC40 gavage in our previous study (50–249 µM) [Citation27]. The anaesthetised animals all showed overt clinical signs of AChE poisoning as infusion of omethoate progressed, notably profuse spontaneous salivation.
shows representative responses to TOF and tetanic stimulation before and approximately 30 min after i.v. administration of omethoate. Omethoate did not inhibit MMG twitch responses (). Rather, as reported by others in studies using isolated rodent muscle [Citation45,Citation46], the immediate effect was the opposite. Overall, the first (T1) twitch contraction in TOF responses increased 2.3 ± 0.2 fold (mean ± S.E.M., range, 1.1–3.6 fold; p < 0.001; paired t-test; ), an increase that may have been partly due to double firing of motor axons in response to single stimuli [Citation47,Citation48]. This potentiation was initially accompanied by a 25 ± 3% decrease in the relative response amplitude during TOF stimulation (T4/T1 amplitude “fade”: range 21–40%, p < 0.001; paired t-test) but in all experiments the T4/T1 ratio either partially or completely recovered as omethoate infusion continued ().
Figure 2. Omethoate potentiates twitch contraction but induces tetanic fade.(A,B) MMG responses of pelvic limb extensor muscle contractions in an anaesthetized pig in response to 2 Hz TOF stimulation (T1–T4) before (A) and 30 min after (B) i.v. infusion of 40 mg kg−1 omethoate. Twitch force increased for all four stimuli (T1–T4) with omethoate but also showed some T1–T4 fade. (C,D) Summary data showing (C) potentiation of T1 twitch force in the MMG and (D) T1–T4 fade, with increasing doses of i.v. omethoate. Fade was consistently reduced after omethoate was increased from 20 mg kg−1 to 40 mg kg−1. Each connected set of points represents data from one animal. (E,F) Averaged tetanic MMG responses with 95% confidence limits (shading) from 8 pigs before (E) and 30 min after (F) omethoate infusion. Before omethoate, tetani were well sustained. However, 30 min after omethoate, the initial peak tetanic force was reduced and showed marked fade during stimulation. (G) Summary data showing peak tetanic force before (CON) and 30 min after i.v. infusion of 40 mg kg−1 omethoate (Ometh). Peak force was less after omethoate in 7/8 animals. (H) Summary data showing peak tetanic force 30 min after i.v. infusion of 40 mg kg−1 omethoate, expressed as a percentage of the peak tetanic response before infusion (%CON) and the amount of tetanic fade at 250, 500 and 750 ms expressed as a % of each muscle’s peak tetanic force. Consistency of tetanic fade between muscles was confirmed by paired Wilcoxon test (p < 0.002 comparing tetanic force at 250 ms and 750 ms in each case).
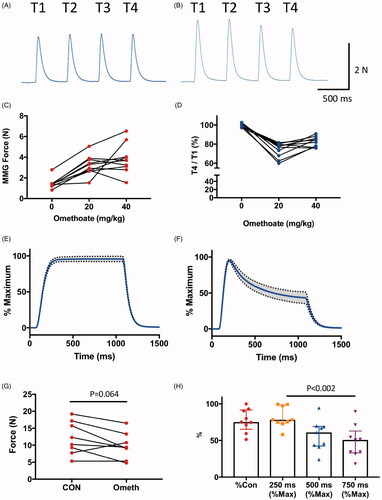
By contrast, tetanic responses were consistently impaired following i.v. omethoate infusion (). Before infusion, tetanic stimulation (50 Hz, 1 s) produced peak MMG force of 12.9 ± 1.7 N (mean ± SEM, n = 8 pigs, ) which was usually sustained (to within 5–10% of peak response) while the nerve was being stimulated. Following i.v. administration of omethoate (40 mg kg−1), peak tetanic force declined by about 20%, to 9.8 ± 1.4 N (p = 0.065, paired t-test) but, more overtly, force production showed consistent and marked fade (). For instance, between 250 ms and 750 ms after the onset of each tetanic stimulus train, overall tension fade represented a decrease of 42 ± 7.2% of peak tetanic force (p = 0.002, Wilcoxon test) ().
MgSO4 infusion mitigated impaired MMG responses
A previous report showed that elevating extracellular concentrations of Mg2+ mitigates deterioration of tetanic responses to OP compounds in isolated rodent preparations [Citation49]. We, therefore, asked whether i.v. infusion of Mg2+, would rescue tetanic fade in our pig model. Six pigs infused with omethoate (40 mg kg−1 boluses) subsequently received 50 mg kg−1 boluses of MgSO4, which increased overall plasma concentration of Mg2+ by about 1–2 mM. In all experiments, loss of peak tetanic force and fade were mitigated (). In one of the pigs, a further dose of omethoate (40 mg kg−1) was administered, which induced additional tetanic fade a few minutes later (). When another bolus of MgSO4 was instilled, tetanic responses partially recovered again. Overall, Mg2+ infusion reduced omethoate-induced tetanic fade by 20–50%. Thus, i.v. administration of Mg2+ partially but reproducibly restored overall neuromuscular function in this model.
Figure 3. Infusion of MgSO4 in isotonic saline mitigated tetanic fade induced by omethoate. (A) MMG recordings of pig pelvic limb extensor muscle contractions in response to tetanic (50 Hz, 1 s) stimulation in an anaesthetized pig receiving two infused doses of omethoate (40 mg kg−1), each followed by a MgSO4 bolus (50 mg kg−1). The first omethoate dose produced modest tetanic fade, improved by MgSO4. A second omethoate dose 1 h later gave rise to more marked tetanic fade by 30 min; following a further MgSO4 dose, there was substantial albeit incomplete recovery and maintenance of the tetanic MMG response. (B,C) Summary data from six experiments showing recovery of peak tetanic force (B) and fade (C) after MgSO4 infusion. Reduced %Peak represents greater fade. p = 0.31 in both B and C, Wilcoxon Test.
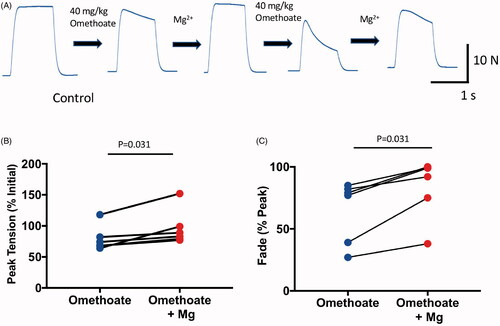
Cyclohexanol impairs neuromuscular function
Next, we asked whether i.v. administration of cyclohexanol, the principal metabolite of the insecticide solvent cyclohexanone, would impair MMG responses in a fashion that mimicked the effects of insecticide gavage [Citation27]. We measured peak response and TOF fade as indices of normal function (see Methods).
We first infused omethoate i.v. in one pig with a bolus i.v. injection of 40 mg kg−1 supplemented by continuous infusion at 2 mg kg−1 h−1. This was followed by increasing doses of cyclohexanol, simulating the effects of metabolism of dimethoate and cyclohexanone (the main constituents of dimethoate EC40). As expected (see ), following omethoate infusion, the first twitch response (T1) was initially potentiated () and this was simultaneously accompanied by a transient decrease of approximately 25% in T4/T1 ratio, which partially recovered over time (). We then infused i.v. cyclohexanol in four successive doses (125 mg kg−1, 250 mg kg−1, 375 mg kg−1 then 500 mg kg−1). shows that initially twitch responses increased but this was followed by progressive decline in twitch amplitude. Following the last dose (500 mg kg−1), MMG responses became very weak, reducing to only 13% of the initial T1 (). Thus, in contrast to omethoate, cyclohexanol caused marked depression of muscle contractile responses.
Figure 4. Cyclohexanol causes concentration-dependent attenuation of neuromuscular responses. (A) Graph of peak MMG twitch responses during TOF (T1–T4) stimulation in an anaesthetised pig. The change in the apparent line thickness throughout the trace in (A,G) indicates the amount of T1–T4 fade. Omethoate potentiated twitch contractions and initially produced discernible TOF fade but this decreased with time. Infusion of cyclohexanol at times and doses indicated progressively abolished TOF contractions. (B–F) Examples of TOF responses obtained 30 min after each omethoate or cyclohexanol dose indicated by arrows in (A). After initial omethoate infusion, twitch force increased with marked T1–T4 fade. Twitch force increased with further omethoate but fade reduced. Cyclohexanol reduced twitch force almost immediately and enhanced T1–T4 fade. A larger dose of cyclohexanol substantially reduced and equilibrated all four twitch responses. (G) Graph of peak MMG twitch responses during TOF (T1–T4) stimulation in an anaesthetised pig receiving only cyclohexanol. Infusion of increasing doses of cyclohexanol progressively abolished TOF contractions. (H–J) Traces obtained 30 min after each cyclohexanol dose indicated in (G). Twitch depression was apparent after each dose with progressive decrease in the T4/T1 ratio but there was partial recovery with time. Responses were substantially reduced in amplitude and showed marked T4/T1 fade after the highest dose (500 mg kg−1) of cyclohexanol. (K) MMG response to tetanic stimulation (40 Hz, 2 s) after administration of 500 mg kg−1 cyclohexanol, immediately prior to euthanasia at the study’s end, showing marked and rapid fade following an initial tension summation (Compare with response to omethoate, ). (L–M) Summary data from 7 pigs, showing significant reduction in T1 twitch force and T4/T1 fade ratio 30 min after infusion of increasing doses of cyclohexanol (**p < 0.02; ANOVA post hoc Dunnett’s test). Bars show mean ± SEM. Recordings from one of the seven pigs showed no apparent change but, overall, fade increased by about 50% (p < 0.001, paired t-test).
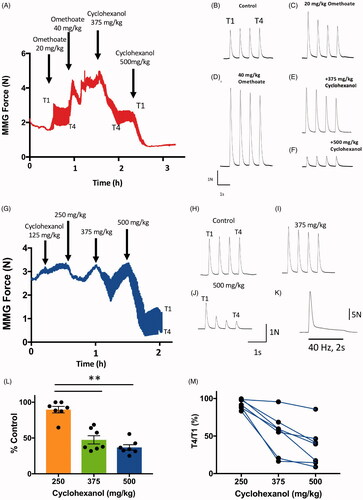
Next, we examined the effects of i.v. administration of cyclohexanol alone (). We measured MMG responses In 7 anaesthetised pigs after infusion of increasing i.v. doses up to 500 mg kg−1, administered over a 30–60 min period, mimicking the serum accumulation of cyclohexanol after gavage of cyclohexanone that we reported in our previous study [Citation27]. Analysis of venous blood samples showed that by the end of the experiments the median serum concentration of cyclohexanol in these pigs was 11.9 mM (range 7–39 mM) but there was no discernible correlation with the strength of the effect over this final range of concentrations (data not shown). In contrast to the hypersalivation effect of omethoate, pigs infused with cyclohexanol showed cutaneous vasodilation (flushing), hypotension (counteracted by i.v. injection of therapeutic doses of noradrenaline) and quiescent EEG, suggesting multiple effects on peripheral and central nervous system function. However, in the present study we focused on neuromuscular function.
MMG responses to TOF stimulation declined progressively after infusion of cyclohexanol, conspicuously at doses greater than 375 mg kg−1 (). Consistent reduction of muscle responses was observed with administration of cyclohexanol at a dose of 500 mg kg−1 (). Within 15–30 min of this highest dose, the first twitch contraction (T1) in the TOF decreased to a median of 35% of the pre-infusion T1 twitch response (IQR = 32–43%; p = 0.0014, repeated measures ANOVA; ). Simultaneously, there was substantial and consistent fade in the TOF responses (median T4/T1 ratio = 39%, IQR 15–45%; p = 0.009, repeated measures ANOVA; ), qualitatively similar to what we had previously observed following insecticide gavage [Citation27]. Higher frequency stimulation (40–50 Hz) caused steep fade and failure to sustain tetanic muscle force (; compare with ).
Cyclohexanol-induced myasthenia is temperature sensitive
Experiments in rodents suggest that the lethality of alcohols, such as ethanol, is temperature dependent [Citation50,Citation51]. We therefore asked whether reducing core temperature would alleviate neuromuscular block following infusion with cyclohexanol (500 mg kg−1). In four pigs that received cyclohexanol i.v., we waited until partial block and fade in the TOF had developed and then applied cooling measures (see Methods). shows data from these four individual pigs, to indicate the consistent and variable aspects of the responses.
Figure 5. Reducing core temperature mitigated effects of cyclohexanol on MMG response. (A–D) Continuous records of peak MMG tension during TOF stimulation in four anaesthetised pigs following infusion of cyclohexanol (500 mg kg−1) then application of cooling measures to reduce core temperature to 34–35 °C, showing partially reversal of (A–C) or arrested (D) decline in MMG tension. Deterioration in the MMG resumed on re-warming. (E–L) Measurements of MMG twitch duration, based on rise times (E–H) and half-width (I–L), during the same experiments. The recordings show that cooling reversibly increases these measures of muscle function. Line thicknesses indicate the upper (T1) and lower (T4) bounds of these measures of MMG responses.
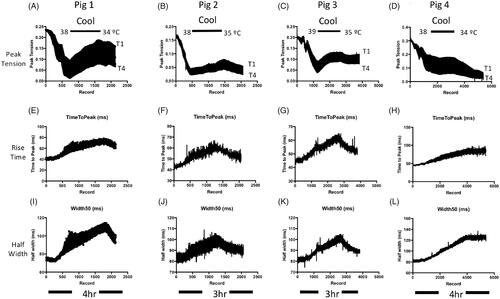
By 30 min, the T1 response had reduced to 45 ± 7% (mean ± SEM) of initial tension and fade (T4/T1 ratio) was 38 ± 5%. We then induced mild hypothermia, reducing core temperature to 34–35 °C from the initial core temperature of 38–39 °C. In three of the animals, the peak (T1) MMG response improved, by 40–70%, as core temperature was reduced (). In the fourth pig, the decline in the T1 response was arrested (). T1 responses deteriorated again in all four animals during passive rewarming, decreasing by a further 10–57% as surface and core temperature gradually recovered (). T4/T1 ratio also improved with cooling, by 5–26% in three of the pigs although in the fourth, T4/T1 fade deteriorated by an additional 11%. In all four pigs, as expected, cooling was accompanied by consistent slowing of twitch duration [Citation52,Citation53], indicated by partially reversible increases in T1 twitch rise time () and half width (). That is, T1 rise time transiently increased, by 26 ± 4%, and half width increased by 18 ± 5% (p < 0.05, paired t-tests). These additional effects on twitch duration were also reversed or arrested upon passive rewarming, providing an independent indicator of the effect of cooling on MMG responses.
In sum, elevating plasma levels of cyclohexanol, with or without conjoint OP administration, replicated qualitatively what we had observed previously following dimethoate insecticide gavage in vivo [Citation27]. The data therefore support the hypothesis that conversion of cyclohexanone solvent to cyclohexanol is the most likely proximate cause of acute neuromuscular paralysis following ingestion of insecticides that contain cyclohexanone as the principal solvent. For a more detailed and quantitative analysis, we turned to the effects of omethoate and cyclohexanol, separately and together, in isolated nerve-muscle preparations from mice.
Mg2+ mitigates muscle contractures ex vivo
We initially made force recordings from isolated mouse phrenic nerve-hemidiaphragm muscle preparations and these showed distinctive fade during tetanic stimulation after 20–60 min exposure to omethoate (data not shown), similar to MMG recordings we obtained from pigs (see ). We also noted localised contractures of the motor endplate region in the mouse hemidiaphragm preparations. Direct observation of muscle fibres and motor endplate responses in triangularis sterni (TS), a thin costal respiratory muscle more favourable for muscle fibre imaging, showed that nerve stimulation at 20–50 Hz in omethoate caused long-lasting contractures localised to about 200 µm either side of the motor endplate region; these contractures persisted for several seconds beyond the period of stimulation (Supplementary Figure 1; Supplementary Movies 1 and 2). We refer to the period of prolonged contracture beyond that of stimulation as “aftercontraction”. These phenomena are consistent with previous studies of the effects of OP compounds on muscle responses to repetitive stimulation [Citation45,Citation54–56].
Force recordings from isolated mouse FDB nerve-muscle preparations – which comprise short muscle fibres, about 500 µm in total length, including their motor endplates – turned out to provide a robust method for quantifying the endplate aftercontractions. shows tetanic tension responses of an isolated FDB muscle before and about 1 h after adding omethoate (150 µM) to the MPS bathing solution, then stimulated at 20 Hz for 1 s. In this example, the brisk tetanic response during stimulation in control MPS solution was transformed to a sustained tetanus and aftercontraction that diminished slowly over the following 20–30 s.
Figure 6. Aftercontractions of isolated FDB muscles are reversibly mitigated by increasing extracellular Mg2+. (A–F) Isometric force recordings in response to 50 Hz stimulation for 1 s, from a mouse FDB muscle following omethoate (150 µM) incubation for 1 h showing marked aftercontractions (B) compared to control (A). Increasing Mg2+ concentration to 2 mM (C), 3 mM (D) and 5 mM (E) progressively reduced, then abolished, the aftercontraction while preserving the tetanic response. (F) Aftercontractions immediately resumed after restoring Mg2+ to 1 mM. (G) Summary data showing magnitude and decay of aftercontractions following 1 h incubation in 150 µM omethoate (t = 0 on x-axis) with 1 mM Mg2+ (red symbols) or 5 mM Mg2+ (cyan symbols). Preparations incubated only in MPS showed no aftercontraction (black line). (H) Summary data showing aftercontractions after incubation for 1 h in a solution containing omethoate and 1 mM Mg2+ (red) or 5 mM Mg2+ (cyan). Aftercontractions were restored 30 min after reducing the 5 mM Mg2+ concentration to 1 mM (magenta).
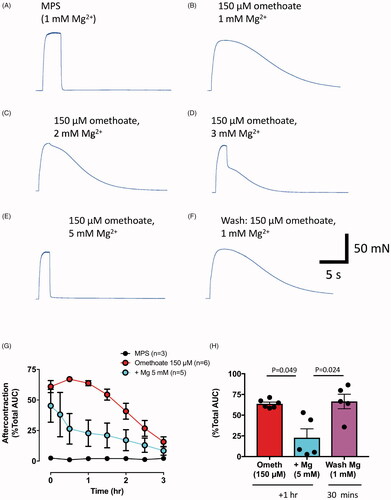
shows the reversible decrease in aftercontraction in a mouse FDB preparation, preincubated for 1.5 hr in omethoate (150 µM), as the concentration of Mg2+ in the bathing medium was increased by 1–4 mM. (A concentration of 2 mM Ca2+ was maintained in these experiments, preserving the safety margin for neuromuscular transmission.) Remarkably, aftercontractions were completely and selectively abolished when Mg2+ concentration was increased to 5 mM, while the tetanic response during stimulation was preserved (compare ). As shown in , after restoring [Mg2+] to 1 mM, with omethoate still present, aftercontractions promptly returned (which we refer to as “rebound”).
The data shown in summarise the time course of decay of aftercontractions in omethoate and the effect of increasing extracellular Mg2+ to 5 mM. After 1–2 h incubation in omethoate, the AUC of the aftercontraction represented about 70% of the total response. Mg2+(5 mM) reduced the median AUC of the aftercontraction about five-fold, to 14% of the response to omethoate in MPS containing 1 mM Mg2+ (p = 0.049, Kruskal–Wallis test with Dunn’s posthoc test). In normal MPS, the magnitude of the aftercontraction slowly declined during a 2–4 hr period after incubation with omethoate. In preparations with increased [Mg2+], however, aftercontractions re-appeared after returning to omethoate solution in normal MPS (). The AUC of these rebounds was not significantly different from the initial aftercontraction, before increasing Mg2+ (p = 0.99, Dunn’s posthoc test).
In sum, in isolated preparations omethoate-induced inhibition of AChE resulted in prolonged nerve-evoked muscle contractures, focused on neuromuscular junctions. Differences in the amount of passive compliance of extrajunctional muscle in long and short muscle fibres (pig pelvic limb and mouse diaphragm or TS muscles, compared with mouse FDB) are likely to have determined whether overall muscle force during these localised contractures are registered by a force transducer as tetanic fade or prolonged tetanic aftercontraction. None the less, as in recordings from pigs (), normal or near-normal functional responses were restored by increasing extracellular (or circulating) [Mg2+].
Cyclohexanol exacerbates then blocks effects of omethoate ex vivo
Next, we examined the combined effects of omethoate and cyclohexanol on isolated preparations, mimicking the simultaneous or sequential effects of OP and solvent metabolism that occurs following OP insecticide ingestion or after i.v. infusion in vivo (compare with ). We measured functional responses to TOF stimulation in isolated FDB preparations.
As in vivo, cyclohexanol initially enhanced TOF potentiation caused by omethoate. Specifically, 1 h after increasing the bath concentration of omethoate to 150 µM, peak twitch force was increased (). Remarkably, within 1–2 min of adding 10 mM cyclohexanol, twitch magnitude and duration during TOF stimulation underwent a further marked increase, resulting in temporal summation of the TOF twitch responses (). Overall, the integrated tension response (AUC) evoked by TOF stimulation was 21.82 ± 7.76 mN.s (n = 4 muscles) before adding cyclohexanol and more than twice as much, 51.57 ± 14.31 mN.s, 1 min later (p = 0.0176, paired t-test; ). However, after 1–10 min, tension amplitude in these recordings began progressively to decline and in seven of the muscles this resulted in complete neuromuscular block (<5% of initial tension; ). In two further muscles we observed similar transient potentiation then block of the TOF tension responses after adding cyclohexanol when neostigmine (5 µM) was used to block AChE activity rather than omethoate (data not shown). Thus, potentiation then block was an effect of cyclohexanol that was evidently not specific to preparations poisoned with OP anti-AChE.
Figure 7. Cyclohexanol enhances then rapidly blocks twitch responses. (A–E) TOF tension responses of a mouse FDB nerve-muscle preparation showing twitch potentiation before (A) and after (B) incubation in omethoate (150 µM) for 1.5 h. (C) Twitches were further potentiated and prolonged by tension summation within 1 min of adding 10 mM cyclohexanol; (D,E) however, by 2 min, the tension responses, while still prolonged, became markedly reduced. (F) Summary of continuous, integrated tension responses in four experiments similar to A–D. Each point is the mean ± SEM of integrated 2 Hz TOF responses sampled at 10 s intervals, showing initial potentiation then depression and block of the evoked tension responses.
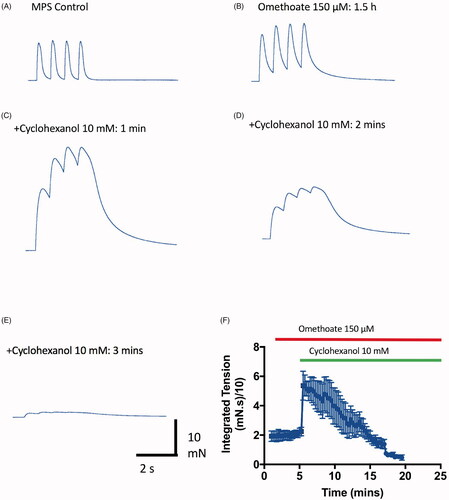
Figure 8. Cyclohexanol causes concentration-dependent and temperature sensitive neuromuscular fade and block in isolated mouse FDB muscles. (A–F) TOF responses of an isolated mouse FDB nerve-muscle preparation showing (A–E) progressive, rapidly reversible decrease in twitch tension and T4/T1 fade ratio at progressively higher concentrations of cyclohexanol, followed by (F) complete recovery on washing with control MPS. (G) Continuous recording of peak tension responses with increasing cyclohexanol concentrations (arrows indicate progressive 5 mM increments) , followed by flushing with MPS (Wash). Upper and lower bounds of the trace indicate the amplitudes of T1 and T4 responses in each TOF response. (H) Continuous recording of peak tension during TOF stimulation showing time course of both decrease in tension and T4/T1 fade ratio following addition of 20 mM cyclohexanol to the recording chamber. (I) Summary data showing cumulative decrease in T1 tension (black symbols) and T4/T1 fade (red symbols) as cyclohexanol concentration was progressively increased in 5 mM increments (as per A–F). Each point shows the mean ± SEM (n = 5 muscles). (J–M) TOF responses of an isolated mouse FDB nerve-muscle preparation incubated in 15 mM cyclohexanol for 10 min at 25 °C (J). The preparation showed only slight decrease in tension and fade; increasing the bathing medium temperature from 30 °C to 32 °C (K,L) was associated with marked decreases. (M) Tension recovered when the bath temperature was restored to 25 °C. (N) Summary data showing the decrease in initial (T1) peak twitch responses (% initial T1 responses) after incubation in cyclohexanol (filled symbols, mean ± S.E.M; n = 4 muscles) versus MPS only (open symbols, n = 6 muscles).
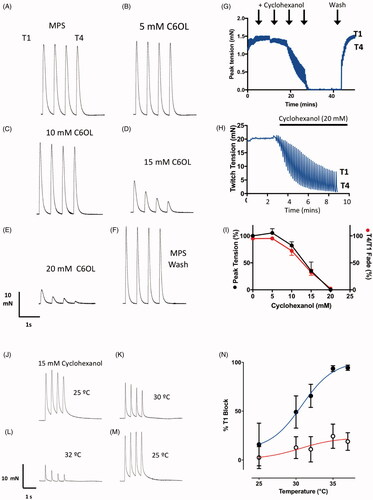
Cyclohexanol alone depresses neuromuscular function ex vivo
Next, we measured the effects of cyclohexanol alone on isolated nerve-muscle preparations. We employed the same TOF pattern of nerve stimulation (2 Hz every 10–30 s) in these murine preparations that we had used in anaesthetised pigs.
Cumulative increases in cyclohexanol (5–25 mM) mimicked the MMG TOF responses we had recorded in pigs. illustrates how cyclohexanol caused progressive, concentration-dependent decreases in FDB muscle twitch tension (T1) and an increase in the amount of T4/T1 fade. Similar effects were observed in isolated rat FDB muscle preparations (data not shown). T1 attenuation and T4/T1 fade developed within 1–5 min of adding cyclohexanol to the bathing medium and were equally rapidly and almost completely reversed on washing the preparations with control MPS solution (). Twitch responses in most preparations were completely blocked in 20–25 mM cyclohexanol, although weak muscle contractions persisted in some muscles (). At lower concentrations, the effect of cyclohexanol was quite variable between muscles. For instance, in three of six preparations in the group of experiments summarised in , 15 mM cyclohexanol almost completely blocked twitch contractions (<5% of initial tension) and in the other three the T1 responses ranged from 48% to 75% of initial control values (overall mean, 23.5%, median 9%, IQR 0–55%; n = 6 preparations). In those muscles that still evoked a T1 response, the median T4/T1 fade ratio in 15 mM cyclohexanol was 38% (range 37–40%). By comparison, in control MPS solution T4 amplitude was consistently sustained at 95 ± 1.4% (mean ± SEM, n = 6) of the initial T1 response (). As in anaesthetised pigs (see ), higher frequency stimulation (up to 50 Hz) in isolated rodent preparations caused sustained responses in control solutions but marked depression after adding cyclohexanol (data not shown).
Cyclohexanol block is temperature dependent
In our previous study [Citation27] we found that cyclohexanol blocked functional neuromuscular responses in vivo when the serum concentration was about 5 mM. However, isolated mouse nerve-muscle preparations initially appeared to require higher concentrations of cyclohexanol than the serum concentrations that inhibited MMG responses in anaesthetised pigs. A key difference in the experimental conditions was that our mouse tissue experiments were performed at ambient laboratory temperature (19–25 °C), whereas the core temperature of the anaesthetised pigs was maintained at 38–39 °C, the normal physiological range for this species. As reported above, TOF fade and block that we observed following i.v. instillation of cyclohexanol in pigs was temperature-sensitive. We therefore examined the temperature sensitivity of neurally-evoked functional responses to cyclohexanol in isolated mouse muscles ().
First, we added cyclohexanol (15 mM) to six mouse FDB preparations. Four of the preparations in this group of experiments showed only a small decrease (less than 25%) in T1 response at room temperature and we tested these for temperature sensitivity. The other two preparations showed complete neuromuscular block at room temperature, as in some other experiments (see above) and these two preparations were therefore not examined further. In the four functioning preparations, we observed a steep and consistent decrease in twitch amplitude and a consistent decrease in T4/T1 ratio as the temperature of the MPS superfusate was progressively increased (). Overall, T1 twitch responses in cyclohexanol were reduced by about 95% within 1–2 min of increasing the temperature of the bathing medium to 35 °C (). This effect was almost completely reversed on rapidly restoring the temperature of the bathing medium to 25 °C (). Twitch responses in control preparations showed only weak sensitivity to temperature.
Fade and block are characteristic muscle force responses observed after incubating muscles with pharmacological blockers of neuromuscular transmission. But cyclohexanol also produced concentration-dependent inhibition of responses to direct muscle stimulation, after blocking neuromuscular transmission with 5 µM d-tubocurarine (Supplementary Figure 2). The dependence of T1 twitch amplitude on cyclohexanol concentration was similar to that of indirect, nerve-evoked responses. With direct stimulation, however, all four responses to TOF stimulation were attenuated equally: there was no fade. These recordings therefore suggest a complex set of underlying cellular and molecular mechanisms of cyclohexanol toxicity, in addition to block of neuromuscular transmission. We did not examine the temperature sensitivity of the responses to direct stimulation in the present study.
Cyclohexanone and xylene also impair neuromuscular function
Cyclohexanone, the principal solvent in dimethoate EC40 and the metabolic precursor of cyclohexanol, also caused neuromuscular fade and block in isolated mouse FDB preparations but at higher concentrations (20–40 mM) than cyclohexanol (). These concentrations were also at least tenfold higher than the peak serum concentration of cyclohexanone reached following insecticide gavage in vivo [Citation27].
Figure 9. Cyclohexanol and xylene also cause neuromuscular fade and block. Representative TOF responses (A,D) and data summarising effects on peak (T1) amplitude (B,E) and T4/T1 fade (C,F), from isolated mouse FDB muscles with increasing amounts of (A–C) cyclohexanone or (D–F) xylene to the bathing medium. Points on the graphs are means ± SEM for four muscles.
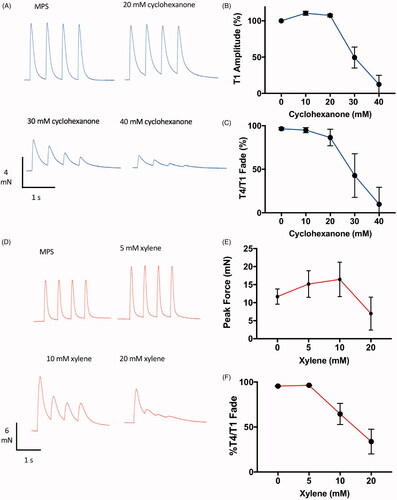
Xylene is another common solvent in industrial formulations of OP insecticides, although making up only 5% w/v in dimethoate EC40 [Citation27]. Xylene also caused marked inhibition, fade and prolongation of TOF twitch responses at concentrations above 10 mM (). We were unable to locate any published data on plasma concentration of xylene or its metabolites in either humans or animal models of pesticide ingestion. This observation therefore merits further investigation.
Discussion
Ingestion of toxic quantities of agricultural OP insecticides continues to be a significant public health issue in rural Asia, where it has led to tens of thousands of deaths annually [Citation3,Citation57]. Patients who initially survive the acute cholinergic crisis caused by AChE poisoning may nevertheless remain very ill for a considerable period and many require intensive care, including those that experience either acute respiratory paralysis or delayed-onset IMS [Citation2,Citation15,Citation17,Citation18]. As in other acute conditions requiring prolonged assisted ventilation [Citation58,Citation59], about half of these patients do not survive. Debate over the causes of acute forms of insecticide-induced paralysis and the more delayed forms including IMS has been unresolved for over 40 years, hindering the development of effective treatments. The present experiments demonstrate compound effects of OP and solvent metabolites on neuromuscular function, potentially reconciling this long-standing debate, and indicate potential treatments.
Our previous research indicated that circulating metabolites of one specific agricultural insecticide, dimethoate EC40, may combine to inhibit neuromuscular function and, specifically, that solvent metabolites are of crucial significance [Citation27]. It was unclear from our previous study, however, whether omethoate or cyclohexanol – respective metabolites of the principal OP and solvent components – were individually responsible or acted in concert. In that study, insecticide was administered in our pig model by gavage, which was important for simulating the ingestion of insecticide by humans. The present study demonstrates the toxicity of the principal metabolites of dimethoate EC40 for neuromuscular function directly, following administration of omethoate or cyclohexanol i.v. in vivo or by adding these compounds to the medium bathing isolated nerve-muscle preparations.
Our main experimental findings were, first, that exposure of muscle to toxic levels of omethoate gave rise to strong contractures of muscle fibres in the region of NMJs, with consequential effects on nerve-evoked muscle contractility. Second, these effects were mitigated by modest increases in extracellular [Mg2+]. Thus, bolus instillation of MgSO4 in vivo, or increasing [Mg2+] (as MgCl2) in extracellular medium bathing isolated preparations facilitated recovery of normal functional responses to repetitive nerve excitation. Third, at concentrations relevant to its toxicity in vivo, cyclohexanol initially exacerbated the effect of blocking AChE by omethoate but neuromuscular responses became blocked as cyclohexanol concentration was increased. Fourth, the myasthenic effects of cyclohexanol were mitigated by reducing core temperature in vivo, and rapidly reversible in vitro.
We discuss first the implications of our findings for the management of acute respiratory failure after patients have intentionally swallowed an OP insecticide. We then consider the implications for understanding and management of delayed-onset neuromuscular paralysis (IMS) in a sub-set of these patients.
Implications for acute respiratory failure after insecticide ingestion
Taking the present and previous studies together, the data strongly suggest that early signs of muscle weakness (myasthenia) after swallowing agricultural insecticides containing cyclohexanone as the main solvent are due mainly to a neuromuscular blocking effect of the principal solvent metabolite, cyclohexanol, rather than AChE inhibition. Cyclohexanol consistently reduced TOF responses and induced TOF fade, both in vivo and in isolated nerve-muscle preparations, thus mimicking the responses to insecticide gavage or signs of neuromuscular weakness in patients who have swallowed insecticide[Citation27]. These functional impairments are also consistent with the decrement-increment characteristics of compound muscle action potentials observed in EMG recordings during neurophysiological investigation of patients who have ingested insecticide [Citation16,Citation24]. TOF fade is a consequence of a reduced safety margin for neuromuscular transmission at NMJs, most likely in this instance to be postsynaptic and due, for example, to inhibition of nicotinic AChR [Citation39,Citation40,Citation60]. In addition, as shown in Supplementary Figure 2, cyclohexanol has direct effects on excitation-contraction coupling or muscle contractility, since adding it to bathing solutions inhibited contractions evoked by direct muscle stimulation. This observation does not detract from the effects of cyclohexanol on neuromuscular transmission but it merits further investigation.
The effects of cyclohexanol in vitro were rapid in onset and rapidly reversible. While rapid clearance of cyclohexanol in patients may not be readily achievable in a clinical context, blocking metabolism of the parent compound (cyclohexanone) using drugs like fomepizole, an inhibitor of the key enzyme alcohol dehydrogenase that catalyses the reduction of cyclohexanone to cyclohexanol [Citation61,Citation62], might be an effective adjunct to treatment. A potential contraindication, however, is that the parent molecule cyclohexanone also inhibits neuromuscular function, albeit at higher concentrations than its metabolite (see ). However, we also showed a strong temperature sensitivity to the myasthenic effects of cyclohexanol. Perhaps targeted temperature management, a similar treatment to that presently utilised for patients with out-of-hospital cardiac arrest [Citation63,Citation64], may therefore also prove beneficial in reversing the acute muscle weakness or paralysis seen in many patients after ingestion of formulated OP insecticides. Solvent may also be responsible for some of the CNS effects associated with the onset of acute respiratory failure. It is not clear whether antagonising the effects on the CNS with cooling will reduce these central effects, as well as the effects on muscle strength.
Cyclohexanol is normally metabolised further and the products then excreted, as 1,2- and 1,4-cyclohexanediols [Citation28,Citation65]. Xylene, another common solvent in agricultural insecticide formulations and which the data in show also impairs neuromuscular function, is normally metabolised then excreted as methyl hippuric acid [Citation66]. Establishing whether these other solvent metabolites also inhibit normal neuromuscular transmission and function in vivo and clinically could prove important for generalising our conclusions regarding toxicity of solvents or their metabolites that we have shown here.
The data also show that, after acutely inhibiting AChE with omethoate, high frequency nerve stimulation in the normal physiological range (20–50 Hz) caused distinctive fade in muscle tension responses in vivo. Our recordings in vitro suggest this is most likely due to localised overstimulation of NMJs. In both instances, Mg2+ treatment partially or completely restored normal neuromuscular responses. Previous studies have also shown that increasing the extracellular concentration of Mg2+ in vitro restores normal characteristics of tetanic tension responses in isolated rodent preparations treated with OP compounds [Citation6]. The mechanism by which Mg2+ mitigates the aberrant tension responses is unclear from the data presented here. It could arise from a combination of effects in damping evoked release of ACh from nerve terminals [Citation67–70] or more direct antagonistic effects on influx of Ca2+ via AChR or regulation of Ca2+ release from postsynaptic intracellular stores in the motor endplate region of muscle fibres [Citation71–75]. Nevertheless, MgSO4 therapy has proved clinically effective in treatment of eclampsia [Citation11–13]. MgSO4 therapies for OP poisoning have been reported in several clinical trials [Citation6–10] and is now the subject of our ongoing study (CCBOC RCT; trial registration number NCT03925025).
Implications for IMS
The data show that cyclohexanol toxicity is likely to be an important contributory factor in early paralysis. However, the formation of this solvent metabolite after swallowing insecticide is unlikely to explain IMS, whose onset may be delayed by up to 72 hrs. As shown by our ex vivo recordings, the acute effects of cyclohexanol are rapid in onset and rapidly reversible. Clearance and further metabolism might therefore be expected to limit the effects of cyclohexanol to just a few hours after ingestion. However, cyclohexanol levels may still be important for delayed induction of neuromuscular paralysis. Our data show that, initially, cyclohexanol exacerbates and prolongs the effects of inhibiting AChE on tension responses (see and ). Thus, depending on the amount and potency of the OP active ingredient, a low dose of OP – from which the patient may otherwise be expected to recover – could become cytotoxic if circulating cyclohexanol also reaches a critical concentration. It is noteworthy that In their original description of IMS, Senanayake and Karalliedde speculated that delayed myopathic changes in the region of motor endplates, described in animal models but not yet definitively shown after OP poisoning in humans, might account for the delayed onset and pathophysiology of IMS [Citation17]. Focal myopathy is mediated by prolonged and increased local Ca2+ influx at NMJs that, acutely, produce focal contractions like those we have also shown here [Citation56,Citation71,Citation73,Citation76–78]. Endplate myopathy also triggers degeneration of motor nerve terminals [Citation79–81]. Thus, by enhancing and prolonging responses of NMJs to relatively low doses of OP inhibitors of AChE, agents like cyclohexanol could facilitate the onset of myopathy and synaptic degeneration, giving rise to clinical signs of IMS. Early intervention to mitigate combined actions of OP and solvent, before delayed-onset/IMS signs develop, may therefore be of crucial benefit to patients vulnerable to IMS. For example, MgSO4 therapy, calcium channel blockade and/or cooling may also reduce the severity of NMJ damage and thereby reduce the incidence or duration of delayed respiratory failure after OP insecticide poisoning as well.
Conclusions
Our findings provide new insight into the causes and mechanisms of acute myasthenia that often follows ingestion of insecticide and which have eluded explanation for more than 40 years. They also offer a new perspective for investigating the causes of delayed-onset neuromuscular paralysis (IMS) and provide a clinically-motivated rationale for obtaining further, detailed mechanistic insights into mechanisms of OP and solvent metabolite toxicity. Our study also indicates novel interventions that could mitigate the harmful effects of insecticide components and/or their metabolites. Two potential therapies, i.v. magnesium sulfate and targeted temperature management, might readily be implemented, at low cost, in regions of the world where the logistics and availability of necessary intensive care, together with the cost of treatment, may inform life-changing clinical decisions.
Supplemental Material
Download MP4 Video (406.3 KB)Supplemental Material
Download QuickTime Video (1.8 MB)Supplemental Material
Download MP4 Video (386.8 KB)Supplemental Material
Download QuickTime Video (2.1 MB)Supplemental Material
Download PDF (221.8 KB)Supplemental Material
Download PDF (4 MB)Acknowledgments
We thank Professors David Beeson, John Harris, Clarke Slater, Angela Vincent, David Wyllie, and Drs John Tattersall, Richard Webster and Charlotte Whitmore for valuable discussions and comments on earlier drafts of the manuscript; Dr Stephen Greenhalgh, Ms Rachael Gregson and support staff at the Wellcome Trust Critical Laboratory in the Roslin Institute for veterinary assistance with in vivo recordings; and Drs Natalie Homer and Scott Denham for plasma measurements of insecticide metabolites.
Disclosure statement
None of the authors have any competing financial and/or non-financial interests in relation to the work described.
Additional information
Funding
References
- Gunnell D, Eddleston M, Phillips MR, et al. The global distribution of fatal pesticide self-poisoning: systematic review. BMC Public Health. 2007;7:357.
- Vale A, Lotti M. Organophosphorus and carbamate insecticide poisoning. Handb Clin Neurol. 2015;131:149–168.
- Karunarathne A, Gunnell D, Konradsen F, et al. How many premature deaths from pesticide suicide have occurred since the agricultural Green Revolution? Clin Toxicol. 2019;389:1–6.
- John H, van der Schans MJ, Koller M, et al. Fatal sarin poisoning in Syria 2013: forensic verification within an international laboratory network. Forensic Toxicol. 2018;36:61–71.
- Chai PR, Hayes BD, Erickson TB, et al. Novichok agents: a historical, current, and toxicological perspective. Toxicol Commun. 2018;2:45–48.
- Bradley RJ. Calcium or magnesium concentration affects the severity of organophosphate-induced neuromuscular block. Eur J Pharmacol. 1986;127:275–278.
- Basher A, Rahman SH, Ghose A, et al. Phase II study of magnesium sulfate in acute organophosphate pesticide poisoning. Clin Toxicol. 2013;51:35–40.
- Philomena J, Sathi V, Prathiba P. A case control study of intravenous magnesium sulphate in treatment of acute organophosphate poisoning. JEMDS. 2016;5:2290–2294.
- Vijayakumar HN, Kannan S, Tejasvi C, et al. Study of effect of magnesium sulphate in management of acute organophosphorous pesticide poisoning. Anesth Essays Res. 2017;11:192–196.
- Jamshidi F, Yazdanbakhsh A, Jamalian M, et al. Effect of adding magnesium sulfate in treatment of organophosphorus poisoning. Open Access Maced. J Med Sci. 2018;6:2051–2056.
- Fawcett WJ, Haxby EJ, Male DA. Magnesium: physiology and pharmacology. Br J Anaesth. 1999;83:302–320.
- Crowther C. Magnesium sulphate versus diazepam in the management of eclampsia: a randomized controlled trial. Br J Obstet Gynaecol. 1990;97:110–117.
- Duley L, Gülmezoglu AM, Henderson-Smart DJ. Magnesium sulphate and other anticonvulsants for women with pre-eclampsia. Cochrane Database Syst Rev. 2003;49:CD000025.
- Eddleston M, Buckley NA, Eyer P, et al. Management of acute organophosphorus pesticide poisoning. Lancet. 2008;371:597–607.
- Eddleston M, Mohamed F, Davies JOJ, et al. Respiratory failure in acute organophosphorus pesticide self-poisoning. QJM. 2006;99:513–522.
- Jayawardane P, Senanayake N, Buckley NA, et al. Electrophysiological correlates of respiratory failure in acute organophosphate poisoning: evidence for differential roles of muscarinic and nicotinic stimulation. Clin Toxicol. 2012;50:250–253.
- Senanayake N, Karalliedde L. Neurotoxic effects of organophosphorus insecticides. An intermediate syndrome. N Engl J Med. 1987;316:761–763.
- He F, Xu H, Qin F, et al. Intermediate myasthenia syndrome following acute organophosphates poisoning–an analysis of 21 cases. Hum Exp Toxicol. 1998;17:40–45.
- Karalliedde L, Baker D, Marrs TC. Organophosphate-induced intermediate syndrome: aetiology and relationships with myopathy. Toxicol Rev. 2006;25:1–14.
- Jayawardane P, Dawson AH, Weerasinghe V, et al. The spectrum of intermediate syndrome following acute organophosphate poisoning: a prospective cohort study from Sri Lanka. PLoS Med. 2008;5:e147.
- Hulse EJ, Davies JOJ, Simpson AJ, et al. Respiratory complications of organophosphorus nerve agent and insecticide poisoning. Implications for respiratory and critical care. Am J Respir Crit Care Med. 2014;190:1342–1354.
- Bird SB, Krajacic P, Sawamoto K, et al. Pharmacotherapy to protect the neuromuscular junction after acute organophosphorus pesticide poisoning. Ann N Y Acad Sci. 2016;1374:86–93.
- Wadia RS, Sadagopan C, Amin RB, et al. Neurological manifestations of organophosphorous insecticide poisoning. J Neurol Neurosurg Psychiatry. 1974;37:841–847.
- Alahakoon C, Dassanayake TL, Gawarammana IB, et al. Prediction of organophosphorus insecticide-induced intermediate syndrome with stimulated concentric needle single fibre electromyography. PLoS One. 2018;13:e0203596.
- Karalliedde LD, Edwards P, Marrs TC. Variables influencing the toxic response to organophosphates in humans. Food Chem Toxicol. 2003;41:1–13.
- De BJ. The intermediate syndrome in organophosphate poisoning: an overview of experimental and clinical observations. J Toxicol Clin Toxicol. 1995;33:683–686.
- Eddleston M, Street JM, Self I, et al. A role for solvents in the toxicity of agricultural organophosphorus pesticides. Toxicology. 2012;294:94–103.
- Mráz J, Gálová E, Nohová H, et al. Uptake, metabolism and elimination of cyclohexanone in humans. Int Arch Occup Environ Health. 1994;66:203–208.
- Buratti FM, Testai E. Evidences for CYP3A4 autoactivation in the desulfuration of dimethoate by the human liver. Toxicology. 2007;241:33–46.
- Ellman GL, Courtney KD, Andres V, et al. A new and rapid colorimetric determination of acetylcholinesterase activity. Biochem Pharmacol. 1961;7:88–95.
- Rosenfeld C, Kousba A, Sultatos LG. Interactions of rat brain acetylcholinesterase with the detergent Triton X-100 and the organophosphate paraoxon. Toxicol Sci. 2001;63:208–213.
- Eyer P, Worek F, Kiderlen D, et al. Molar absorption coefficients for the reduced Ellman reagent: reassessment. Anal Biochem. 2003;312:224–227.
- Harris JB, Ribchester RR. The relationship between end-plate size and transmitter release in normal and dystrophic muscles of the mouse. J Physiol. 1979;296:245–265.
- Ribchester RR, Thomson D, Wood NI, et al. Progressive abnormalities in skeletal muscle and neuromuscular junctions of transgenic mice expressing the Huntington's disease mutation. Eur J Neurosci. 2004;20:3092–3114.
- Feng G, Mellor RH, Bernstein M, et al. Imaging neuronal subsets in transgenic mice expressing multiple spectral variants of GFP. Neuron. 2000;28:41–51.
- Wong F, Fan L, Wells S, et al. Axonal and neuromuscular synaptic phenotypes in Wld(S), SOD1(G93A) and ostes mutant mice identified by fiber-optic confocal microendoscopy. Mol Cell Neurosci. 2009;42:296–307.
- McArdle JJ, Angaut-Petit D, Mallart A, et al. Advantages of the triangularis sterni muscle of the mouse for investigations of synaptic phenomena. J Neurosci Methods. 1981;4:109–115.
- Clutton RE, Dissanayake K, Lawson H, et al. The construction and evaluation of a device for mechanomyography in anaesthetized Göttingen minipigs. Vet Anaesth Analg. 2013;40:134–141.
- Bowman WC. Neuromuscular block. Br J Pharmacol. 2006;147(Suppl 1):S277–S286.
- McGrath CD, Hunter JM. Monitoring of neuromuscular block. Continuing education in anaesthesia. Critical Care Pain. 2006;6:7–12.
- Ribchester RR, Mao F, Betz WJ. Optical measurements of activity-dependent membrane recycling in motor nerve terminals of mammalian skeletal muscle. Proc Biol Sci. 1994;255:61–66.
- Edelstein AD, Tsuchida MA, Amodaj N, et al. Advanced methods of microscope control using μManager software. J Biol Methods. 2014;1:10.
- Khajehali J, Van Leeuwen T, Grispou M, et al. Acetylcholinesterase point mutations in European strains of Tetranychus urticae (Acari: Tetranychidae) resistant to organophosphates. Pest Manag Sci. 2010;66:220–228.
- Ben ON, Bakas I, Istamboulié G, et al. Acetylcholinesterase immobilized on magnetic beads for pesticides detection: application to olive oil analysis. Sensors. 2012;12:7893–7904.
- Burd PF, Ferry CB. A prolonged contraction at the end-plate region of the diaphragm of rats and mice after anticholinesterases in vitro. J Physiol. 1987;391:429–440.
- Clark AL, Hobbiger F. Twitch potentiation by organophosphate anticholinesterases in rat phrenic nerve diaphragm preparations. Br J Pharmacol. 1983;78:239–246.
- Clark AL, Hobbiger F, Terrar DA. Nature of the anticholinesterase-induced repetitive response of rat and mouse striated muscle to single nerve stimuli. J Physiol. 1984;349:157–166.
- Ribchester RR, Taxt T. Motor unit size and synaptic competition in rat lumbrical muscles reinnervated by active and inactive motor axons. J Physiol. 1983;344:89–111.
- Brvar M, Chan MY, Dawson AH, et al. Magnesium sulfate and calcium channel blocking drugs as antidotes for acute organophosphorus insecticide poisoning – a systematic review and meta-analysis. Clin Toxicol. 2018;56:725–736.
- Malcolm RD, Alkana RL. Temperature dependence of ethanol lethality in mice. J Pharm Pharmacol. 1983;35:306–311.
- Finn DA, Boone DC, Alkana RL. Temperature dependence of ethanol depression in rats. Psychopharmacology. 1986;90:185–189.
- Bennett AF. Thermal dependence of muscle function. Am J Physiol. 1984;247:R217–R229.
- Holewijn M, Heus R. Effects of temperature on electromyogram and muscle function. Eur J Appl Physiol Occup Physiol. 1992;65:541–545.
- Chang CC, Hong SJ. A regenerating release of acetylcholine from mouse motor nerve terminals treated with anticholinesterase agents. Neurosci Lett. 1986;69:203–207.
- Hong SJ, Chang CC. Transmitter-mediated local contracture of the endplate region of the focally innervated mouse diaphragm treated with anticholinesterase. Br J Pharmacol. 1993;109:1178–1185.
- Ferry CB, Cullen MJ. Myopathic changes in indirectly stimulated mouse diaphragm after ecothiopate in vitro. Int J Exp Path. 1991;72:329–343.
- Eddleston M. Patterns and problems of deliberate self-poisoning in the developing world. QJM. 2000;93:715–731.
- Botta M, Tsonas AM, Pillay J, et al. Ventilation management and clinical outcomes in invasively ventilated patients with COVID-19 (PRoVENT-COVID): a national, multicentre, observational cohort study. Lancet Respir Med. 2021;9:139–148.
- Hazard D, Kaier K, Cube von M, et al. Joint analysis of duration of ventilation, length of intensive care, and mortality of COVID-19 patients: a multistate approach. BMC Med Res Methodol. 2020;20:206–209.
- Wood SJ, Slater CR. Safety factor at the neuromuscular junction. Prog Neurobiol. 2001;64:393–429.
- Cronholm T. Isotope effects and hydrogen transfer during simultaneous metabolism of ethanol and cyclohexanone in rats. Eur J Biochem. 1974;43:189–196.
- Mráz J, Gálová E, Nohová H, et al. Effect of ethanol on the urinary excretion of cyclohexanol and cyclohexanediols, biomarkers of the exposure to cyclohexanone, cyclohexane and cyclohexanol in humans. Scand J Work Environ Health. 1999;25:233–237.
- Schwartz BG, Kloner RA, Thomas JL, et al. Therapeutic hypothermia for acute myocardial infarction and cardiac arrest. Am J Cardiol. 2012;110:461–466.
- Arrich J, Holzer M, Havel C, et al. Hypothermia for neuroprotection in adults after cardiopulmonary resuscitation. Cochrane Database Syst Rev. 2016;2:CD004128.
- Mráz J, Gálová E, Nohová H, et al. 1,2- and 1,4-Cyclohexanediol: major urinary metabolites and biomarkers of exposure to cyclohexane, cyclohexanone, and cyclohexanol in humans. Int Arch Occup Environ Health. 1998;71:560–565.
- Choi CY, Cho N, Park SY, et al. Urine methyl hippuric acid levels in acute pesticide poisoning: estimation of ingested xylene volume and association with clinical outcome parameters. J Korean Med Sci. 2017;32:2051–2057.
- Elmqvist D, Hofmann WW, Kugelberg J, et al. An electrophysiological investigation of neuromuscular transmission in myasthenia gravis. J Physiol. 1964;174:417–434.
- Maselli RA, Wollman RL, Leung C, et al. Neuromuscular transmission in amyotrophic lateral sclerosis. Muscle Nerve. 1993;16:1193–1203.
- Hubbard JI, Jones SF, Landau EM. On the mechanism by which calcium and magnesium affect the release of transmitter by nerve impulses. J Physiol. 1968;196:75–86.
- Bennett MR, Florin T, Hall R. The effect of calcium ions on the binomial statistic parameters which control acetylcholine release at synapses in striated muscle. J Physiol. 1975;247:429–446.
- Fucile S, Sucapane A, Grassi F, et al. The human adult subtype ACh receptor channel has high Ca2+ permeability and predisposes to endplate Ca2+ overloading. J Physiol. 2006;573:35–43.
- Hess P, Lansman JB, Tsien RW. Calcium channel selectivity for divalent and monovalent cations. Voltage and concentration dependence of single channel current in ventricular heart cells. J Gen Physiol. 1986;88:293–319.
- Zayas R, Groshong JS, Gomez CM. Inositol-1,4,5-triphosphate receptors mediate activity-induced synaptic Ca2+ signals in muscle fibers and Ca2+ overload in slow-channel syndrome. Cell Calcium. 2007;41:343–352.
- Piccari V, Deflorio C, Bigi R, et al. Modulation of the Ca(2+) permeability of human endplate acetylcholine receptor-channel. Cell Calcium. 2011;49:272–278.
- Moriconi C, Di Castro MA, Fucile S, et al. Mechanism of verapamil action on wild-type and slow-channel mutant human muscle acetylcholine receptor. J Neurochem. 2010;114:1231–1240.
- Leonard JP, Salpeter MM. Agonist-induced myopathy at the neuromuscular junction is mediated by calcium. J Cell Biol. 1979;82:811–819.
- Mosca B, Eckhardt J, Bergamelli L, et al. Role of the JP45-calsequestrin complex on calcium entry in slow twitch skeletal muscles. J Biol Chem. 2016;291:14555–14565.
- Meshul CK, Boyne AF, Deshpande SS, et al. Comparison of the ultrastructural myopathy induced by anticholinesterase agents at the end plates of rat soleus and extensor muscles. Exp Neurol. 1985;89:96–114.
- Laskowski MB, Olson WH, Dettbarn WD. Ultrastructural changes at the motor end-plant produced by an irreversible cholinesterase inhibitor. Exp Neurol. 1975;47:290–306.
- Rich M, Lichtman JW. Motor nerve terminal loss from degenerating muscle fibers. Neuron. 1989;3:677–688.
- Duxson MJ, Vrbová G. Inhibition of acetylcholinesterase accelerates axon terminal withdrawal at the developing rat neuromuscular junction. J Neurocytol. 1985;14:337–363.