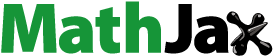
ABSTRACT
Consumer, legal, and technological factors influence the design, performance, and emissions of light-duty vehicles (LDVs). This work examines how design choices made by manufacturers for the UK market result in emissions and performance of vehicles throughout the past decade (2001–2011). LDV fuel consumption, CO2 emissions, and performance are compared across different combinations of air and fuel delivery system using vehicle performance metrics of power density and time to accelerate from rest to 100 km/h (62 mph, tz-62). Increased adoption of direct injection and turbocharging technologies helped reduce spark ignition (SI, gasoline vehicles) and compression ignition (CI, diesel vehicles) fuel consumption by 22% and 19%, respectively, over the decade. These improvements were largely achieved by increasing compression ratios in SI vehicles (3.6%), turbocharging CI vehicles, and engine downsizing by 5.7–6.5% across all technologies. Simultaneously, vehicle performance improved, through increased engine power density resulting in greater acceleration. Across the decade, tz-62 fell 9.4% and engine power density increased 17% for SI vehicles. For CI vehicles, tz-62 fell 18% while engine power density rose 28%. Greater fuel consumption reductions could have been achieved if vehicle acceleration was maintained at 2001 levels, applying drive train improvements to improved fuel economy and reduced CO2 emissions. Fuel consumption and CO2 emissions declined at faster rates once the European emissions standards were introduced with SI CO2 emissions improving by 3.4 g/km/year for 2001–2007 to 7.8 g/km/year thereafter. Similarly, CI LDVs declined by 2.0 g/km/year for 2001–2007 and 6.1 g/km/year after.
1. Introduction
High-volume light-duty vehicle (LDV) manufacturers are subject to financial penalties if their fleet-average emissions ratings exceed limits defined by legislation. These CO2 emissions targets are currently set at 130 g CO2/km by the European Union and will reduce to 95 g CO2/km from 2020 onward (EC Citation2009). While financial penalties can be mitigated with the sale of low emission vehicles (less than 50 g CO2/km), such as electric vehicles (EV) and plug-in hybrid electric vehicles (PHEV), there are strong incentives for manufacturers to improve the fuel economies of vehicles using conventional powertrains. This study investigates how present legislation and other less well-defined influences have affected UK passenger vehicle designs with a focus on rates of technology adoption, vehicle performance, and CO2 emissions. This allows the effectiveness of European LDV emissions standards to be determined and vehicle design trends to be assessed.
Previous assessments of passenger vehicle developments have focused largely on the North American market. In particular, Chon & Heywood (Citation2000) and Heywood & Welling (Citation2009) introduced scaling relationships to quantify the effects of vehicle design changes on spark ignition (SI) naturally aspirated (NatA) engine performance (expressed in terms of brake mean effective pressure and specific power). A subsequent study by An & DiCicco (2007) related trade-offs between US passenger vehicle performance, size, and fuel economy into linearized indices. The relationships highlighted the strong influence of the power-to-mass ratio on vehicle power demands. Similarly, Santini & Anderson (Citation1993) and MacKenzie & Heywood (Citation2012) identified statistical dependencies that relate US vehicle performance to engine characteristics. A comparison between coefficients of determination (R2) revealed the MacKenzie & Heywood (Citation2012) models to be significantly more accurate. This improvement is attributed to the inclusion of additional independent variables. Yet, data limitations frequently hinder efforts to improve the performance of LDV energy-demand models. Indeed, the limited availability of detailed design data has historically hindered the analysis of UK LDV trends, where a particular absence of deductive statistical relations is noted.
This paper examines the impact of vehicle technology in the European market by using a uniquely comprehensive dataset of the 35,000Footnote1 distinct vehicle models, comprising all types of vehicles made available for sale in the UK between 2001 and 2011. The size of this dataset stands in contrast to the number of vehicles used in other studies, such as 150 by Chon & Heywood (Citation2000) and 1,500 by Heywood & Welling (Citation2009).
Background information specific to this historical analysis is presented in Section 2, where the data are summarized and different definitions of vehicles performance are introduced. These data are used to identify how reductions in LDV fuel consumption were achieved between 2001 and 2011 in terms of propulsion system and combination of air and fuel delivery (AFD) system. The results are presented in Section 3 and discuss annual and normalized trends, with a commentary on the effectiveness vehicle design characteristics.
2. Vehicle performance
2.1 Classification
Vehicle performance is represented in a variety of ways including acceleration time, maximum power, or maximum torque. Such metrics depend on a variety of LDV design factors, including: vehicle mass, rotational-engine inertia, aerodynamic drag, rolling resistance, and engine power.
The most obvious differentiator of vehicle performance is by fuel type (gasoline or diesel). Additional differentiation is possible through the AFD system. Indeed, engine air delivery systems are classified by their boosted (turbocharged, T, or supercharged, S) or unboosted, naturally aspirated designsFootnote2. Fuel delivery systems are classified by their methods for fuel injection (indirect injection, II, or direct injection, DI). Such differentiation significantly impacts the rated power, fuel efficiency, and CO2 emissions of an engine (Longbao et al., Citation1999; Patterson et al., Citation1994; Yang & Anderson, Citation1998). For example, SI engines tend to operate less efficiently than CI due both to their operation while throttled, which reduces volumetric efficiency, and their use of lower compression ratios to limit knock.
2.2 Data
The dataset compiled for analysis from UK government sources (DfT, Citation2014) and CAP Consulting (CAP, 2013) consisted of more than 35,000 vehicle models available for sale on the UK market representing more than 95% of vehicle sales. LDVs are referred to as “available vehicles” and are not sales-weighted. The particular variants of vehicles are referred to as “models,” such as Audi A6 Saloon. The CAP dataset includes design metrics (bore, stroke, number of cylinders, compression ratio, vehicle mass, fuel type, and fuel delivery method); performance metrics (maximum torque, maximum power, and acceleration time from 0 to 100 km/h); market data (period of sale and suggested retail prices); and fuel economy over the New European Driving Cycle (NEDC). The UK government data provided additional auxiliary information and was used as a quality check for model type and UK availability, which allowed for elimination of spurious entries with the CAP dataset. Semi-complete model data, whereby model and make were confirmed, were used for available design metrics even if other metrics were absent, as was common within the dataset. An example of the dataset is shown in the supporting information (Table S1).
2.3 Derivation of scaling relationships
To facilitate the analysis of vehicle performance trends across engines with different geometries, LDV metrics are normalized relative to their displacement volume (). For example, maximum power density measures use of the displaced volume to deliver maximum brake power () [12]:
(1)
(1)
The maximum engine torque divided by the engine displacement is another comparative relationship, which is represented by the brake mean effective pressure (BMEP) (Gupta, Citation2006). For a four-stroke internal combustion engine (ICE), BMEP is related to brake work per cycle (), the number of crank revolutions for each power stroke (
= 2), and the maximum rated torque ():
(2)
(2)
Finally, a distinct dependency exists between vehicle acceleration (), velocity (
), and power-to-vehicle mass ratios (), as derived by An & DeCicco (Citation2007) and shown in Equation (Equation3
(3)
(3) ). Building on this relationship, the trade-off between vehicle fuel consumption and the provision of LDV utilities is distilled into a single Performance Fuel-Economy Index (
) in Equation (Equation4
(4)
(4) ). Here, fuel-economy (
) is represented using the NEDC ratings, while performance is represented using either the time to accelerate from 0 to 100 km/h (0 to 62mph),
or the power-to-mass ratio
(3)
(3)
(4)
(4)
3. Results
3.1 Availability of vehicle technologies
3.1.1 Availability by fuel-type
The numbers of passenger vehicles available for sale are reviewed to identify how LDV availability has affected fleet-average fuel consumption. Manufacturers increased the absolute number of passenger vehicle models between 2001 and 2011 from 892 to 3,387 for CI LDVs and 2,792 to 3,512 for SI LDVs. The percentage of SI vehicles available to the market decreased from 74% to 50% across the decade as CI vehicle numbers simultaneously increased from 24% to 49%. This result provides anecdotal evidence of efforts by manufacturers to sell more efficient CI vehicles with mean fuel consumption that is consistently lower than that of an SI vehicle ().
Figure 1. Annual mean fuel consumption (l/100 km) for vehicles by fuel type, as presented in the raw vehicle data. Disjoint correlations for EV and SI (E85) vehicles are attributed to sample bias (i.e. low availability). SI/LPG and SI/CNG refer to bi-fuel vehicles.
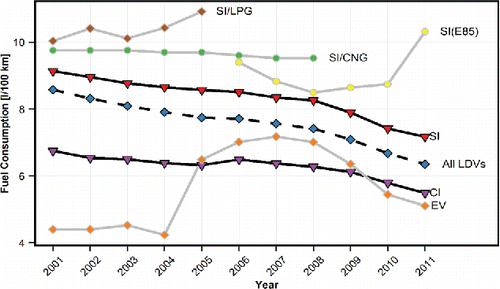
depicts the fuel consumption throughout the decade of vehicles with different engine technologies. Rates of vehicle fuel economy improvements increased after the introduction of mandatory emissions standards in 2007. SI fuel consumption decreased at an annual mean rate of 2.2% between 2001 and 2007 and 3.5% between 2007 and 2011. By comparison, CI fuel consumption declined by 1.9% and 3.5% per annum across the same periods, respectively. Such improvements helped mean fuel consumption to fall by 26% for all LDVs, from 8.6 l/100 km in 2001 to 6.4 l/100 km by 2011 (blue diamonds in ).
Finally, the number of vehicles using non-conventional propulsion systems available to the market (defined as EVs, HEVs and those using liquid petroleum gas (LPG), compressed natural gas (CNG) systems and ethanol) peaked at 2.2% in 2007. While ethanol fuel-blend (E85) LDVs were the most common non-conventional LDV for sale between 2001 and 2007, their non-ICE market share declined from 83% in 2007 to 32% by 2011. At the same time, the percentage of EVs and PHEVs increased from 4% to 68% between 2001 and 2011 due to government incentives to promote low-emissions EV sales.
3.1.2 Availability by air and fuel delivery system
Engine designers have primarily used four combinations of AFD technologies since 2001, spark ignited, naturally aspirated vehicles with indirect (SI-NatA II) and direct injection (SI-NatA DI), and turbocharged spark and compression ignition vehicles with direct injection (SI-T DI and CI-T DI, respectively). The market share for these systems was 97% in 2011 as shown in . The market share of CI-T DI systems increased from 22% to 53% as that of SI-NatA II systems decreased from 59% to 27% over the decade. The annual percentage of turbocharged vehicle models available increased from 31% to 68% between 2001 and 2011. This is attributed to manufacturers' attempts to increase their range of CI LDVs. Simultaneously, the availability of SI-DI vehicles increased from 3% to 11% and SI-II availability decreased from 57% to 26%. shows that SI-DI systems were $14,700 more expensive than SI-II systems in 2011, representing both an increased cost of manufacture and penetration of new technology into higher-priced vehicles. The 2011 fuel consumption by SI-DI vehicles was 2% higher, while acceleration time was 27% faster and vehicle mass was 21% lower, compared to SI-II vehicles. Therefore, the rise in availability of DI vehicles at higher cost demonstrates the manufacturers' response to consumer demands of improved acceleration while maintaining comparable fuel economy.
Figure 2. Relative availability of LDVs by air-delivery systems, fuel type, and year. CI-NatA vehicles are represented in the “Other” category and NotAv refers to SI vehicles whose fuel delivery system was not specified in the CAP data.
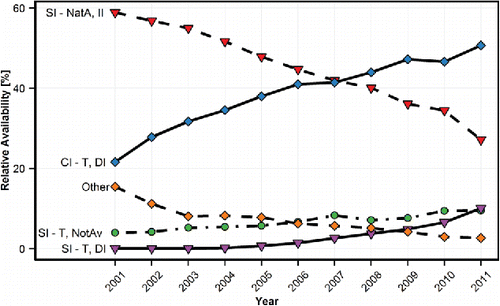
Table 1. Average characteristics of DI and II fuel delivery systems in 2001 and 2011, by fuel type.
Consumer demands and regulatory pressures have brought about reductions in fuel consumption for nearly all vehicle designs. As shown in , the mean fuel consumption of CI-TDI and SI-NatA II systems declined by 18% and 22%, respectively, across the decade, with fuel consumption by the former being lower consistently. SI-T, DI vehicles saw a dramatic reduction in fuel consumption as more vehicles adopted this design (cause of discontinuity). While DI engines are typically more efficient than II, the higher fuel consumption of DI vehicles in 2011 represents the technology being adopted in more massive vehicles with faster acceleration (e.g. luxury brands) as is typical of new automotive technologies. The rise in fuel consumption for SI-NatA, DI is a result of deviations within a relatively small sample (<20).
3.2 Vehicle design trends
Annual changes in UK LDV compression ratios, engine sizes, and mean piston speeds were reviewed to determine how reductions in fuel consumption were achieved. Normalized annual changes were also considered to assess how vehicle design characteristics have changed over time relative to their 2001 baseline values.
3.2.1 Compression ratio
High compression ratios are desired to improve fuel conversion efficiencies (Heywood, Citation1988), yet high in-cylinder pressures are limited by engine knock in SI LDVs. Over the past decade, mean SI compression ratios increased by 3.7% to improve efficiencyFootnote3. During that period boosted (turbo and super charged) engines maintained 11% lower compression ratios than un-boosted SI engines to limit engine knock (Gromping, Citation2006; Gupta, Citation2006; Lindeman and Lindeman, Citation1980). Likewise, demonstrates that the compression ratios increased for all combinations of SI AFD systems, except those with DI. SI DI compression ratios declined 13% between 2006 and 2011 due to the increased use of boosted air-delivery systems, i.e. the number of SI-T DI vehicles increased from 29% in 2006 to 86% in 2011.
Figure 4. Annual mean compression ratios for SI and CI engines, segregated by air (dashed lines) and fuel delivery (solid line) system.
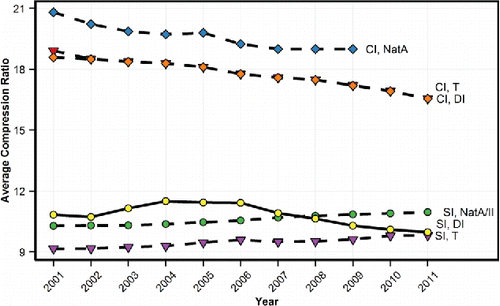
By comparison, high in-cylinder pressures are limited by requirements for stronger, heavier materials in CI vehicles. shows CI compression ratios declined at an annual mean rate of 1.3% between 2001 and 2011. Mean acceleration times and fuel consumption each improved (i.e. decreased) by 19% during this period. The reduced compression ratios can be attributed to higher utilization of common rail injection, which allows lower compression ratios and increased power output (Stumpp & Ricco, Citation1996), i.e. diesel vehicles with common rail injection had a 9% higher power output (BMEP) than the mean of all CI vehicles. Additionally, a concentrated effort by manufacturers to adhere to the increasingly stringent European noxious emissions standards resulted in reduced compression ratios to lower the peak combustion temperatures and associated formation of nitrous oxides (NOx) (EC, Citation2012).
3.2.2 Engine geometry
Mean engine volumes declined 6–7% for all LDVs between 2001 and 2011. SI and CI acceleration times improved (e.g. reduced) by 11% and 19%, respectively, across the decade as the power-to-mass ratio increased. The improvement in acceleration performance is linked to manufacturers' increased use of DI and turbocharged technologies. These technologies allow vehicle performance to be decoupled from the size of the engine, thus increasing engine power while reducing engine displacement volume. shows the number of engine cylinders declined by 5% in SI LDVs and 1% in CI between 2001 and 2011. This change in number of cylinders occurred independently to changes in engine size (mean displacements increased in some engines but decreased in others), as shown in .
Figure 5. (a) Number of cylinders and (b) mean volume of SI and CI engines by the number of cylinders.
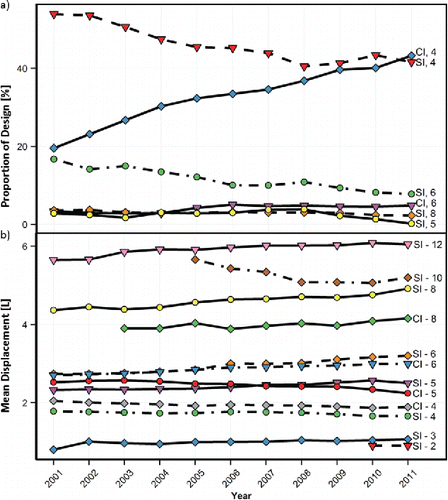
The annual mean bore-to-stroke ratios remained relatively constant in CI vehicles (−0.7% change) and decreased by 5.1% in SI vehicles between 2001 and 2011 (see SI ). Lower bore-to-stroke ratios favor high tumble generation in modern SI LDVs, which ensures more complete in-cylinder mixing (Mattarelli et al., Citation2013). Therefore, the reductions in bore-to-stroke ratios suggest an attempt by SI engine designers to reduce emissions and increase efficiencies (Lee, Bae, & Kang, Citation2007).
3.2.3 Power density
Normalized engine design metrics allow for comparison of technological LDV advancements, independent of engine size. Power density increased between 2001 and 2011, as engine designers utilized volumetric efficiency improvements for all combinations of AFD systems and in-cylinder charge cooling within direct injection systems. As shown in , changes in power density were most pronounced for turbocharged and DI systems, increasing by 38% and 29%, respectively, across the decade. The mean specific power in 2001 was consistently higher for boosted over comparable un-boosted engines, at 23% for SI-T LDVs and 38% for CI-T LDVs. The mean specific power in 2001 was consistently higher for the DI over comparable II system, at 25% for SI-DI engines and 11% for CI-DI engines.
3.2.4 Brake mean effective pressure
The normalized engine work, BMEP, of SI and CI vehicles reveals their engine capacity to produce work increased by 38% and 18%, respectively, since 2001 (see ). The mean BMEP was 11% higher for the mean turbocharged LDV, relative to non-boosted engines (NatA), due to improved volumetric efficiencies. BMEP was 45% higher for the mean SI-DI, relative to SI-II, engines in 2011 due to reduced throttling losses in lean-burn DI engines and the adoption of turbochargers. Overall, the BMEP increased for all combinations of AFD system between 2001 and 2011, as shown in . This was attributed to increases in torque, while engine size decreased 6–7% across the decade, as seen in 4 cylinder vehicles within . Differences between mean BMEP at maximum power and torque decreased for CI vehicles (from 21% in 2001 to 18% in 2011). This is the likely consequence of increased adoption of turbocharged air-delivery systems and reductions in CI frictional losses (Xin, Citation2011).
Table 2. BMEP at maximum torque by AFD system.
3.3 Design trade-offs
The advancement of vehicle engine technologies resulted in an overall improvement in fuel consumption and performance over the decade, while vehicle mass remained roughly constant. The degree to which manufacturers choose to apply engine improvements manifest to fuel consumption reductions (equivalent to CO2 emissions reduction), or improved performance (decreased acceleration time) is a result of design choice, which is influenced by consumer demand and government regulations (Cheah et al., Citation2008). As shown in , the acceleration time decreased in proportion to the mass-to-power ratio over the decade with 2.3% per year reduction from 2001 to 2007 (14.2% over period), which reduced to 1.2% per year from 2007 to 2011 (4.7% over period). Conversely, fuel economy improved at a rate of only 0.9% per year from 2001 to 2007, which increased to 5.4% per year increase from 2007 to 2011. The change in manufacturer emphasis from performance to fuel economy and reduced CO2 emissions correspond to the EU's mandatory emissions standards which were introduced in 2007 (EC, Citation2009). These results highlight the degree to which regulatory standards drive improvements in fuel economy and CO2 emissions that do not result from market forces alone.
In order to assess the overall rate of technology improvement in the automotive sector, the PFI [Equation (Equation4(4)
(4) )] is shown to increase at a near-constant rate regardless of emphasis on performance or fuel economy. The annual improvement in PFI for LDV designs from 2001 to 2011 is estimated to be 4.9% for the mean CI LDV. From this rate of improvement roughly half (54%) of engine improvements were devoted toward increased efficiency with the remaining being devoted toward improved acceleration. As a result it is estimated that fuel consumption and CO2 emissions could have been reduced by nearly twice the amount (49% reduction projected vs. 21.5% actual) achieved for new vehicles had manufacturers' optimized vehicles for fuel economy improvements over the decade. This would have resulted in the mean 2011 CI fuel consumption of 4.4 L/100 km (5.5 L/100 km actual) and CO2 emissions of 117 g/km (145 g/km actual), which would have been equivalent to meeting 2015 legislated values (120 g/km without biofuel credit) 4 years in advance.
Similar to CI vehicles, the overall technology improvement for SI vehicles over the decade represented by PFI was 3.7% per year as shown in . The mean SI fuel economy increased by 29% between 2001 and 2011, where the rate of improvement was higher from 2007–2011 (4.6% per year) than 2001–2007 (1.8%). The SI vehicle fuel economy improvements were a result of increased compression ratios (3.6%), reduced mean vehicle mass (1.1%) and reduced mean engine size (5.7%) across the decade. Designers also introduced turbochargers and DI systems, which reduced mean SI vehicle acceleration times by 9.4% and increased both power and torque by 17% and 15%, respectively, over the same period. The PFI increased by 43% over the decade, indicating that technology improvements in SI were comparable to CI vehicles. Over the decade, SI vehicles had a majority (76%) of technology improvements dedicated to fuel economy rather than performance. Had the entirety of SI improvements been dedicated to fuel efficiency, the mean 2011 CI fuel consumption and CO2 emissions would be 6.2 L/100 km and 149 g/km, respectively, compared to the observed values of 7.1 L/100 km and 173 g/km. Note that the mean CO2 emissions of SI vehicles alone would still exceed the 2015 values (130 g/km with biofuel credit), which indicates why diesel vehicles (CI) have been viewed by automobile manufacturers as necessary to meet legislative targets.
Figure 8. Normalized (2001 reference) trends for SI vehicle characteristics and performance fuel-economy index (PFI), where the blue line represents the PFI regression.
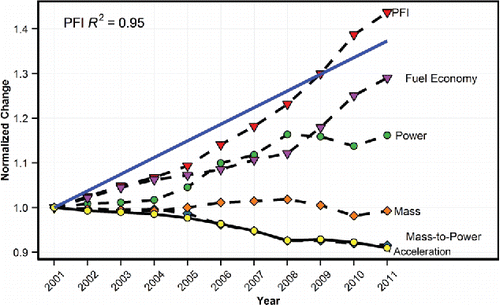
In addition to trading power for fuel economy, vehicle manufacturers also trade vehicle mass for performance and fuel economy. As shown in , the mass-to-power ratio is directly correlated (1:1) to the vehicle acceleration times with higher mass resulting in slower acceleration. Additionally, vehicle mass is linked to fuel consumption, which can also be correlated (SI ). Regression analysis indicates that the fuel consumption scales linearly with vehicle mass (R2 > 0.73). These results indicate that each 100-kg reduction in vehicle mass led to reduced fuel consumption by a mean 0.62 L/100 km in CI LDVs (orange diamonds) and 0.42 L/100 km in SI LDVs (green circles). Such results highlight the fuel economy improvements that are possible through vehicle “light weighting” by means of incorporation of light-weight composites, engine downsizing, and reduced vehicle footprint. Despite the adoption of these technologies to some degree over the decade, masses remained roughly constant as increased requirements for safety features and consumer demands for larger interior volume counteracted such measures.
4. Conclusion
The impact of LDV technology drivers and design trends on performance, fuel economy, and CO2 emissions was investigated by analysis of national engineering data for the UK vehicle fleet (>99% of available vehicles) during the past decade (2001–2011). This allowed for the success of the European emissions standards to be objectively identified, as manufacturers increased their efforts to reduce fuel consumption after 2007. Annual improvements in SI vehicle fuel efficiency went from 1.8% between 2001 and 2007 to 4.6% between 2007 and 2011, which corresponds to an equivalent reduction in CO2 emissions. CI fuel and CO2 savings similarly increased from 0.9% to 5.4% during the same periods.
Mean LDV fuel consumption declined 26% across the decade. Manufacturers modified ICE designs across the decade to ensure such reductions were achieved, as availability of turbochargers, CI engines, and DI fuel-delivery systems increased from 31% to 68%, 24% to 49%, and 23% to 60%, respectively. Moreover, different design strategies were used to improve fuel economies in SI and CI LDVs: SI manufacturers increased compression ratios (4%) and reduced engine size (6%); and CI manufacturers increased the use of turbochargers from 23% in 2001 to 49% in 2011. Indeed, design modifications were predominantly evolutionary, as the availability of vehicles with non-conventional powertrains (e.g. electric vehicles) peaked at 2% in 2007 and cumulative reductions in fuel consumption amounted to 22% (SI) and 19% (CI).
Finally, LDV performance increased across all AFD systems. Turbocharged CI-DI vehicles had the highest BMEP at 1,800 kPa and turbocharged SI-DI vehicles had the highest power density at 71 kW/l. The trade-off between vehicle performance and fuel consumption is combined into a single-performance fuel-economy index, showing that cumulative technological improvements (i.e. acceleration time and fuel economy) increased at annual mean rates of 4.3% for SI vehicles and 4.9% for CI, which places manufacturers' efforts to reduce fuel consumption into context.
UJST_A_1243282_Supplementary_materials.pdf
Download PDF (1.7 MB)Funding
The authors acknowledge the EPSRC funding provided for this work under the Centre for Sustainable Road Freight Transport (EP/K00915X/1) and the Energy Efficient Cities Initiative (EP/F034350/1).
Notes
1 16% of vehicles had an unspecified AFD system and were removed.
2 For the same performance, a turbocharged engine is significantly smaller than the equivalent naturally aspirated engine, and will have lower frictional losses.
3 The overall increase in the mean SI compression ratios was linked to the high availability of II technologies as the most common method for fuel delivery from 2001 to 2011 ().
References
- An, F., & DeCicco, J. (2007). Trends in technical efficiency trade-offs for the U.S. light vehicle fleet. SAE Technical Paper. Retrieved from http://papers.sae.org/2007-01-1325/
- CAP Automotive. (2013). CAP. Leeds. Retrieved from http://www.cap.co.uk
- Cheah L. W., Bandivadekar, A. & Bodek, K. (2008). The Trade-off between Automobile Acceleration Performance, Weight, and Fuel Consumption. SAE Technical Paper. Retrieved from http://www.sae.org/technical/papers/2008-01-1524.
- Chon, D. M., & Heywood, J. B. (2000). Performance scaling of spark-ignition engines: Correlation and historical analysis of production engine data. Reprinted from: Modeling of SI Engines. SAE World Congress 724.
- DfT. (2014). Vehicle licensing statistics. London. Retrieved from https://www.gov.uk/government/collections/vehicles-statistics.
- EC. (2009). Regulation (EC) No 443/2009 of the European Parliament and of the Council of 23 April 2009, pp. 1–15. Retrieved from http://eur-lex.europa.eu/LexUriServ/LexUriServ.do?uri=OJ:L:2009:140:0001:0015:EN:PDF
- EC. (2012). Commission Regulation (EU) No 630/2012 of 12 July 2012 amending Regulation (EC) No 692/2008, as regards type-approval requirements for motor vehicles fuelled by hydrogen and mixtures of hydrogen and natural gas with respect to emissions, and the inclusion.
- Gupta, H. N. (2006). Fundamentals of Internal Combustion Engines. PHI Learning Pvt. Ltd., Delhi Retrieved from http://books.google.co.in/books/about/Fundamentals_of_Internal_Combustion_Engi.html?id=MFx4VRErHNoC&pgis=1
- Gromping, U. (2006). Relative importance for linear regression in R: The package relaimpo. Journal of Statistical Software 17(1), 1–27.
- Heywood, J. (1988). Internal combustion engine fundamentals, vol. 21. McGraw-Hill. Retrieved from http://www.lavoisier.fr/livre/notice.asp?id=OOLWROAOK66OWE.
- Heywood, J. B., & Welling, O. Z. (2009). Trends in performance characteristics of modern automobile SI and diesel engines. SAE International 2, 1650–1662.
- Lee, K., Bae, C., & Kang, K. (2007). The effects of tumble and swirl flows on flame propagation in a four-valve S.I. engine. Applied Thermal Engineering 27, 2122–2130.
- Lindeman, R., Merenda, P. F., & Gold, R. Z. (1980). Introduction to bivariate and multivariate analysis. Scott, Foresman & Company, Glenview. Retrieved from http://www.sidalc.net/cgi-bin/wxis.exe/?IsisScript=COLPOS.xis&method=post&formato=2&cantidad=1&expresion=mfn=005517.
- Longbao, Z., Hewu, W., Deming, J., & Zuohua, H. (1999). Study of Performance and Combustion Characteristics of a DME-Fueled Light-Duty Direct-Injection Diesel Engine. International Fuels & Lubricants Meeting & Exposition. Retrieved from http://papers.sae.org/1999-01-3669/
- MacKenzie, D., & Heywood, J. B. (2012). Acceleration performance trends and the evolving relationship among power, weight, and acceleration in US light-duty vehicles: A linear regression analysis. Transportation Research Board 91st Annual Meeting. Retrieved from http://web.mit.edu/sloan-auto-lab/research/beforeh2/files/MacKenzie&Heywood-TRB-12-1475.pdf (accessed Feb. 28, 2013).
- Mattarelli, E., Cantore, G., & Rinaldini, C. A. (2013). Advances in Internal Combustion Engines and Fuel Technologies. Advances in Internal Combustion Engines and Fuel Technologies. Retrieved from http://www.intechopen.com/books/advances-in-internal-combustion-engines-and-fuel-technologies
- Patterson, M. A., Kong, S., Hampson, G.,& Reitz, R. (1994). Modeling the Effects of Fuel Injection Characteristics on Diesel Engine Soot and NOx Emissions. International Congress & Exposition. Retrieved from http://papers.sae.org/940523/
- Santini, D. J., & Anderson, J. (1993). Determinants of multiple measures of acceleration. SAE Technical Paper. Retrieved from http://www.osti.gov/energycitations/servlets/purl/10181845-IIuDKk/10181845.pdf.
- SMMT. (2013). Motor industry facts 2012. Retrieved from http://www.smmt.co.uk/2012/03/motor-industry-facts-2012/.
- Stumpp, G., & Ricco, M. (1996). Common rail—An attractive fuel injection system for passenger car DI diesel engines. SAE Technical Paper. Available: http://papers.sae.org/960870/.
- UN. (2005). UN Regulation (1958 Agreement) Addendum 100: Regulation No. 101, no. March 1958. UN Economic Commission for Europe. Retrieved from http://www.unece.org/fileadmin/DAM/trans/main/wp29/wp29regs/r101r2e.pdf
- Xin, Q. (2011). Diesel engine system design (1st ed.). Delhi, India: Woodhead Publishing. Retrieved from http://sutlib2.sut.ac.th/sut_contents/H140796.pdf
- Yang, J., & Anderson, R. W. (1998). Fuel injection strategies to increase full-load torque output of a direct-injection SI engine. International Congress & Exposition. Retrieved from http://papers.sae.org/980495/.