Abstract
Histone acetylation modulates gene expression and has been described as increased in systemic lupus erythematosus (SLE). We investigated interferon regulatory factor 1 (IRF1) interactions that influence H4 acetylation (H4ac) in SLE. Intracellular flow cytometry for H4 acetylated lysine (K) 5, K8, K12, and K16 was performed. Histone acetylation was defined in monocytes and T cells from controls and SLE patients. RNA-Seq studies were performed on monocytes to look for an imbalance in histone acetyltransferases and histone deacetylase enzyme expression. Expression levels were validated using real-time quantitative RT-PCR. IRF1 induction of H4ac was evaluated using D54MG cells overexpressing IRF1. IRF1 protein interactions were studied using co-immunoprecipitation assays. IRF1-dependent recruitment of histone acetyltransferases to target genes was examined by ChIP assays using p300 antibody. Flow cytometry data showed significantly increased H4K5, H4K8, H4K12, and H4K16 acetylation in SLE monocytes. HDAC3 and HDAC11 gene expression were decreased in SLE monocytes. PCAF showed significantly higher gene expression in SLE than controls. IRF1-overexpressing D54MG cells were associated with significantly increased H4K5, H4K8, and H4K12 acetylation compared to vector-control D54MG cells both globally and at specific target genes. Co-immunoprecipitation studies using D54MG cells revealed IRF1 protein-protein interactions with PCAF, P300, CBP, GCN5, ATF2, and HDAC3. ChIP experiments demonstrated increased p300 recruitment to known IRF1 targets in D54MG cells overexpressing IRF1. In contrast, p300 binding to IRF1 targets decreased in D54MG cells with IRF1 knockdown. SLE appears to be associated with an imbalance in histone acetyltransferases and histone deacetylase enzymes favoring pathologic H4 acetylation. Furthermore, IRF1 directly interacts with chromatin modifying enzymes, supporting a model where recruitment to specific target genes is mediated in part by IRF1.
Introduction
Interferon regulatory factors (IRFs) are a family of transcription factors that are the intracellular mediators for type I interferons (IFNs). Type I IFNs have been strongly implicated in the pathogenesis of systemic lupus erythematosus (SLE). IRFs bind to the interferon stimulated responsive element (ISRE) and modulate IFN genes themselves as well as IFN-inducible genes. All nine members of the IRF family (IRFs 1–9) share a highly conserved N-terminal DNA-binding domain characterized by 5 tryptophan repeats forming a helix-loop-helix motif that binds to ISRE found in target promoters. IRF members have distinct roles in the regulation of early immune response to pathogens, hematopoiesis, carcinogenesis, cell growth, and differentiation. Aberrant function and gene expression of IRFs are increasingly linked to SLE pathophysiology. Genetic variations of IRFs 5, 7, and 8 have been associated with SLE susceptibility.
In our previous work using ChIP arrays with an antibody that recognized multiple acetylated lysine residues on H4, we observed increased H4ac specifically at genes predicted to be regulated by IRF1, as well as higher expression of the same IRF1-regulated genes in the monocytes of patients with SLE,Citation1 thereby associating IRF1 with the dysregulated gene expression in SLE. This study was designed to investigate whether IRF1 directly participated in histone acetylation. IRF1 is the first member of the IRF family to be identified and it is ubiquitously expressed at a low basal level in most cell types. It is induced by type I and II IFNs, as well as dsRNA, cytokines, and hormones, such as prolactin. IRF1 is centrally involved in the regulation of host defense and the development and differentiation of cells in the immune system.Citation2 It can stimulate type I IFN promoters and activate the transcription of genes involved in both innate and adaptive immunity.Citation3
To understand the relationship of IRF1 with hyperacetylation of H4 in SLE, we investigated IRF1 interactions with enzymes involved in H4 acetylation. We found a background of dysregulated histone acetyltransferase (HAT) and histone deacetylase (HDAC) expression in SLE, favoring histone acetylation, but we also identified IRF1 interactions with several HAT enzymes, allowing for targeting of HATs to interferon (IFN)-inducible genes.
Patients and Methods
Sample collection
This study was given approval by the Institutional Review Board. All patients and controls consented to participate in the study. Female patients with SLE, age >18 years, were recruited from the Hopkins Lupus Cohort, an ongoing long-term SLE cohort consisting of approximately 2000 patients, where patient inclusion is based on a clinical diagnosis of SLE.Citation4 We minimized potential confounders due to immunosuppressive medications by recruiting only patients with mild-moderate disease who were on no medications other than low-dose prednisone (≤5 mg/day), non-steroidal anti-inflammatories and/or hydroxychloroquine. Patients with severe anemia, disease flare or on cyclophosphamide or other immunosuppressives were not enrolled. Controls were comparable to patients in gender and age. Blood from female controls were obtained from a campus core facility and handled to mimic the SLE samples.
D54MG in vitro models
D54MG, a human glioma-derived cell line, was cultured in Dulbecco's modified Eagle's medium (Life Technologies, Grand Island, NY, USA) plus 10% HycloneTM Cosmic CalfTM serum in a 37°C incubator with 5% CO2.
For the IRF1 overexpression experiments, the IRF1-puromycin plasmid was constructed by subcloning the IRF1 human cDNA ORF clone (Origene, Rockville, MD, USA) into pCMV6-A-Puro vector (Origene). Transfection of D54MG cells with IRF1-puromycin plasmid was performed using the AmaxaTM Cell Line NucleofectorTM Kit V (Lonza, Basel, Switzerland). The D54MG cells were also transfected with empty pCMV6-A-puro vector as a control.
A silencing vector expressing a siRNA targeting the human IRF1 gene was used for the IRF1 knockdown experiments. psiRNA-hIRF1 plasmid and its empty vector control si-LucGL3 (InvivoGen, San Diego, CA, USA) were transfected into D54MG cells using the AmaxaTM Cell Line NucleofectorTM Kit V (Lonza). Knockdown and overexpression were confirmed by Western blot.
Histone 4 acetylation
Detection of acetylated H4 lysines K5, K8, K12, and K16 in peripheral blood mononuclear cells (PBMCs) and D54MG cells was performed by flow cytometry. PBMCs were separated from whole blood samples by Ficoll-Paque® (GE Healthcare Life Sciences, Piscataway, NJ, USA) density centrifugation. The PBMCs were surface stained using mouse anti-human surface markers for T cells, monocytes and B cells (anti-CD3 FITC, anti-CD14 PE, and anti-CD19 PE-CY7– BD Biosciences, San Jose, CA, USA). The cells were then fixed and permeabilized using the BD Cytofix/Cytoperm™ Kit (BD Biosciences) to allow for intracellular staining of H4 lysines by rabbit anti-human antibodies to total H4ac, H4K5ac, H4K8ac, H4K12ac, and H4K16ac (EMD Millipore, Billerica, MA, USA). The specificities of the antibodies were established by Western blotting and by the Antibody Validation Database (http://compbio.med.harvard.edu/antibodies/). The cells were then incubated with anti-rabbit antibodies conjugated with fluorochrome 647 (Life Technologies). D54MG samples were intracellularly stained in identical manner as described for the PBMCs, without the initial surface staining. All the samples were fixed in 1% paraformaldehyde and run on the Accuri C6 Flow Cytometer (BD Biosciences) using 4-color analyses. Rabbit anti-GST was used to define background staining.
RNA extraction and gene expression studies
Total RNA was isolated from purified primary monocytes. Primary monocytes were separated from lymphocytes in PBMCs by adhesion on gelatin-coated plastic, which achieved a cell purity of 90–95%. RNA was prepared using TRI Reagent® (Sigma-Aldrich, St. Louis, MO, USA) and the RNeasy® mini kit (Qiagen, Hilden, Germany). RNA was reverse transcribed into cDNA using the Advantage RT-for-PCR kit (Clontech, Mountain View, CA, USA). Real-time quantitative reverse transcription polymerase chain reactions (qRT-PCR) were performed using the Taqman Gene Expression Assay (Life Technologies) and primers (Life Technologies) for GCN5, PCAF, CBP, P300, ATF2, TIP60, HAT1, HBO1, HDAC3, HDAC11, and SIRT1.
RNA-Seq
RNASeq studies from a published different set of 8 controls and 9 SLE patients were examined to define HAT and HDAC gene expression.Citation5
Immunoprecipitation and western blotting
D54MG cells were washed, lysed in radioimmunoprecipitation assay (RIPA) buffer, sonicated and centrifuged. The supernatant was pre-cleared with Protein A agarose beads (Life Technologies). For each antibody, 1.2 mg of protein was incubated overnight with 2 μg of anti-IRF1 mouse monoclonal IgG1 antibody and then 30 μl of Protein A agarose beads for 1 h at 4°C. The following antibodies (Santa Cruz biotechnologies, Santa Cruz, CA, USA) were used for western blotting: anti-GCN5, anti-PCAF, anti-CBP, anti-P300, anti-ATF2, anti-TIP60, anti-HAT1, anti-HBO1, anti-HDAC3, anti-HDAC11, and anti-SIRT1. Mouse IgG was used as a negative antibody for the immunoprecipitation. Whole cell lysates were used for positive controls.
Chromatin immunoprecipitation (ChIP)
ChIP experiments were carried out as previously described,Citation6,7 with the modification that chromatin fixation was carried out at 37°C for 30 min, as recommended for p300 ChIP experiments.Citation8 p300 antibody from Santa Cruz Biotechnology (C-20, Santa Cruz, CA) and GST antibody from Invitrogen (Camarillo, CA, USA) were used for the ChIP assays.
Statistical analysis
Student's t-test was used in the analyses to determine statistical significance. P-values ≤0.05 were depicted schematically by * in the figures. Error bars were calculated by standard error.
Results
Increased acetylation in H4 lysine residues in SLE primary monocytes
We recruited SLE patients from the Hopkins Lupus Cohort. details the demographic and disease characteristics of our cohort of SLE patients (n = 26). All of the SLE patients were female and the average SELENA-SLEDAI disease activity index was 2.48 and the average ESR was 33 mm/hr. Control subjects (n = 24) were also all female and of comparable ages.
Table 1. Characteristics of SLE patients cohort*
Histone acetylation patterns are shaped by a dynamic balance of HATs and HDACs, which typically target a subset of lysines for modification.Citation9 There are 4 lysine residues on histone H4 that can be acetylated: K5, K8, K12, and K16. Each of these lysine residues are acetylated and de-acetylated by a known set of HATs and HDACs enzymes.Citation10,11 We examined the acetylation pattern of the individual H4 lysine residues in SLE patients as an indicator of which HATs might be dysregulated in SLE. We looked for hyperacetylation of specific H4 lysine residues in SLE cells using flow cytometry to measure global levels of H4 lysine acetylation. CD14+ monocytes were identified by surface staining and physical parameters, as shown in . Intracellular flow cytometry measured the total acetylation of H4, as well as the acetylation of specific H4 lysine residues K5, K8, K12, and K16, as shown in a representative diagram in . shows the averaged median fluorescence intensity collected from 26 SLE patients and 24 controls. Total H4 acetylation (H4ac) was significantly increased in SLE monocytes compared to controls. Significantly increased acetylation at H4K5, H4K8, H4K12, and H4K16 was also seen in SLE monocytes compared to controls.
Figure 1. Intracellular flow cytometry. (A) Monocytes in the peripheral blood mononuclear cells from the SLE patients and controls were identified by physical parameters and (B) CD14+ surface staining. (C) Representative flow results depicting the measurement of the acetylation of individual lysine residues, as well as global H4 acetylation, in an SLE patient.
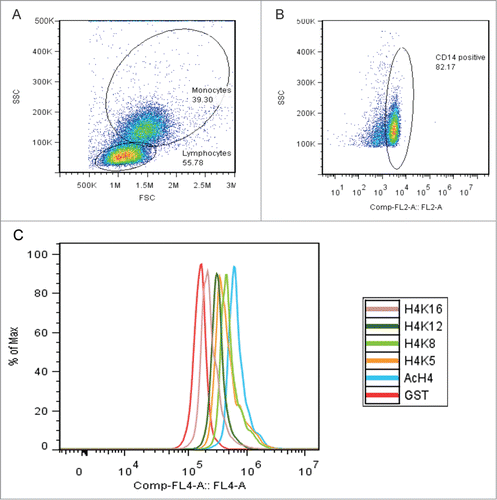
Figure 2. H4 specific lysine acetylation. (A) Flow cytometry on 26 SLE patients and 24 control subjects. Anti-GST is a rabbit IgG control. Total H4 acetylation (H4Ac) is significantly* (P < 0.04) increased in the SLE monocytes as compared to controls. Acetylation at all individual H4 lysine residues (H4K5, H4K8, H4K12 and H4K16) is also significantly* (all P values ≤ 0.05) increased in SLE monocytes over controls. Values are the mean ± SD. (B) Flow cytometry results from vector-control D54MG cells and IRF1 overexpressing D54MG cells. Anti-GST is a rabbit IgG control. Total H4ac in the IRF1 overexpressing cells is significantly* (P = 0.02) increased compared to the control cells. Acetylation at lysine residues H4K5, H4K8, and H4K12 is also significantly* increased (P values ≤ 0.05) in the IRF1 overexpressing cells as compared to vector-controls. Values are the mean ± SD.
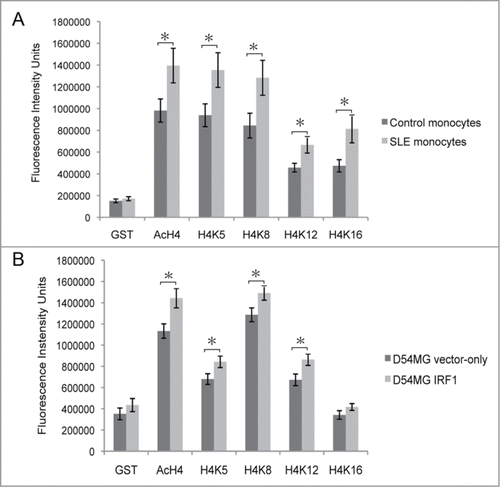
For comparison, we measured acetylation at H4 lysine residues in CD3+ T cells of both SLE patients and controls. We found significantly (P < 0.05) increased H4K8ac and H4K16ac in SLE T cells compared to control T cells. This pattern of increased acetylation across multiple lysine residues suggested that there was broad dysregulation of the acetylation and deacetylation enzymes involved in histone acetylation.
H4 hyperacetylation is favored in patients with SLE
The increase in H4 lysine acetylation could be due to global changes in HAT/HDAC balance or due to increased targeting of HAT enzymes. We investigated potential global HAT and HDAC dysregulation by defining their expression in the monocytes of SLE patients as compared to controls. We first examined our published RNA-Seq data from isolated monocytes of 9 SLE patients and 8 healthy controls.Citation5 We searched for HATs and HDACs with significant differential gene expression between SLE and controls by interrogating the RNA-seq data. There was significantly decreased expression of histone deacetylases HDAC3 and HDAC11 in SLE patients (P = 0.0029 and 0.0092, respectively). In contrast, there was increased expression of PCAF (P = 0.07), which functions to acetylate K5, K8, and K16.
qRT-PCR analysis of HAT/HDAC gene expression was performed to validate the data from the RNA-Seq studies using new samples. qRT-PCR studies were performed using mRNA from purified monocytes of 26 SLE patients and 15 healthy controls (). PCAF had significantly (P = 0.002) higher expression in SLE monocytes than in controls. The expression levels of HDAC11 were significantly (P = 0.04) decreased in SLE as compared to controls, while HDAC3 expression was also lower in SLE monocytes than in controls, though not significantly (P = 0.27). p300 expression was lower in SLE than in control, while the expression levels of other HATs (CBP, ATF2, GCN5, HAT1, TIP60, MOF, and HBO1) were not significantly different between the SLE and control monocytes. The altered balance of HATs and HDACs could contribute to the increased H4 acetylation seen.
Table 2. qRT-PCR analysis of HAT/HDAC* gene expression in SLE and control monocytes‡
IRF1 overexpression in D54MG cells leads to increase in H4 acetylation
We previously found that there was increased H4 acetylation specifically at genes predicted to be regulated by IRF1, and expression of these IRF-regulated genes was increased in SLE monocytes.Citation1 We hypothesized that IRF1 might participate in increased H4 acetylation in SLE. We tested our hypothesis by establishing an in vitro IRF1 overexpression system in D54MG cells. D54MG cells are transformed human glioma cells and they were used in our system because of their response to inflammatory stimuli similar to that seen in monocytes and their ability to tolerate IRF1 overexpression.
Flow cytometry studies compared the acetylation levels of individual H4 lysine residues (K5, K8, K12, and K16) in D54MG cells transfected with IRF1 with those in D54MG cells transfected with the vector-control. shows the gating of D54MG cells transfected with IRF1. A representative figure showing intracellular flow measurement of total H4 acetylation and the acetylation of H4K5, H4K8, H4K12, and H4K16 is shown in . IRF1 gene expression was increased more than 9-fold (P = 0.03) in the IRF1 overexpression cells compared to vector-control cells (). There was a significant increase in total H4 acetylation in the IRF1 overexpressing cells as compared to vector-control cells as measured by flow cytometry (). Additionally, H4K5ac, H4K8ac, and H4K12ac were also significantly increased in the cells with IRF1 overexpression compared to vector-controls. No increase in acetylation was seen at H4K16 as a result of IRF1 overexpression. These data support a pivotal role for IRF1 in the hyperacetylation phenotype seen in SLE. While it did not perfectly replicate the pattern in SLE, these results demonstrate that IRF1 can drive H4 acetylation.
Figure 3. Intracellular flow cytometry. (A) Viable D54MG cells were identified by physical parameters. (B) Representative flow results depicting the measurement of the acetylation of individual lysine residues, as well as global H4 acetylation, in D54MG cells. GST represents an off target control antibody.
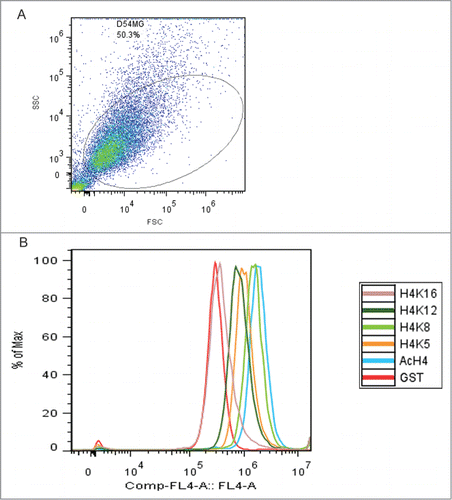
Figure 4. IRF1 gene expression levels in D54MG overexpression and knock-down cells, as normalized to their respective vector-control cells and expressed as fold-change differences. (A) IRF1 gene expression is increased fold9- (P = 0.03) in the IRF1 overexpressing cells over the control cells. (B) IRF1 gene expression is decreased by 40% (P = 0.004) in the IRF1 knockdown cells as compared to the control cells.
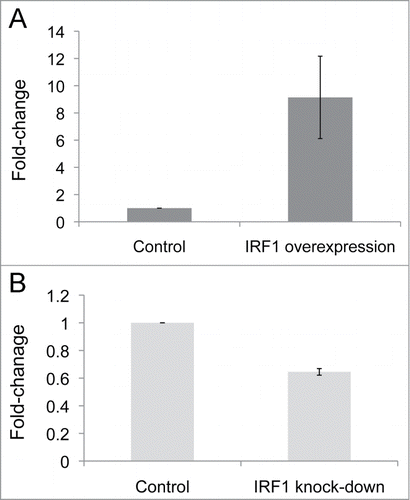
We also investigated the HAT/HDAC gene expression levels in the in vitro IRF1 overexpression system by qRT-PCR (). We did not see marked changes in HAT and HDAC gene expression. Therefore, the role of IRF1 does not revolve around substantial changes to the HAT/HDAC balance.
Figure 5. HAT/HDAC gene expression levels in vector-control D54MG cells versus IRF1 overexpressing D54MG cells. The qRT-PCR results (n = 5 pairs) from IRF1 overexpressing cells were normalized to the vector-control cells and expressed as fold-change differences. An imbalance of HATs and HDACs gene expression that would promote the H4 hyperacetylation observed in the IRF1 over-expressing D54MG cells was not found.
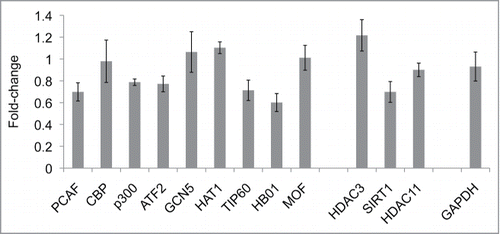
IRF1 interactions with HATs and HDACs in D54MG cells
The overexpression of IRF1 led to H4 hyperacetylation, signifying that IRF1 directly or indirectly regulated histone acetylation. We proceeded to investigate potential IRF1 interactions with selected HATs and HDACs in order to define the mechanism of H4 hyperacetylation due to IRF1 overexpression. An IRF1 immunoprecipitation survey of the HAT enzymes was performed. IRF1 interactions with HDACs were also examined. The co-immunoprecipitation studies were done in D54MG under both unstimulated and stimulated conditions. The cells were stimulated with interferon α (IFN-α), an important cytokine in SLE pathophysiology.
We found protein-protein interactions between IRF1 and several HATs: PCAF, ATF2, p300, CBP, and GCN5 (). IRF1 interacted with PCAF and ATF2 regardless of stimulation, whereas IRF1 bound to CBP, p300, and GCN5 conditionally under IFN-α stimulation. The conditional binding with IRF1 occurred quickly, within 1 h of IFN-α stimulation and disassociated by 4 h after stimulation. Similarly, IRF1 associated with HDAC3 within 1 h of IFN-α stimulation and uncoupled by 4 h post stimulation ().
Figure 6. IRF1 interactions with HATs and HDACs. (A) An IRF1 co-immunoprecipitation survey of HATs active on H4 lysine groups is shown. IRF1 interactions were examined under unstimulated conditions, as well as a time course of IFN-a stimulation over 24 h. IRF1 binds with PCAF and ATF2 in both unstimulated and stimulated states. IRF1 interactions induced by IFN-a stimulation are seen with CBP, p300, GCN5. Mouse IgG was used as a negative control for the immunoprecipitation. The positive control for each individual HAT is shown in the lysate column. (B) An IRF1 co-immunoprecipitation survey with selected HDACs is shown. IRF1 association with HDAC3 is seen at 1 h after IFN-α stimulation and disassociates by 4 h. (C) The IRF1 immunoprecipitation for the HAT study is shown to demonstrate that IRF1 recovery was comparable across all time points.
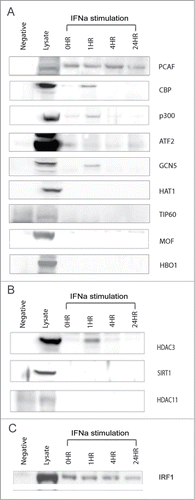
IRF1 recruitment of p300 to target genes
We hypothesized that IRF1 recruits HATs to the regulatory regions of genes, serving a targeting function. Chromatin immunoprecipitation (ChIP) assays were performed with p300 in IRF1 overexpressing cells and IRF1 knockdown cells to test this hypothesis. Target genes (INHBA, TDP2, TAP1, TNFRSF14, IFIH1, and SERPINE1) that were previously shown to have both increased IRF1 binding and mRNA expression in monocytes from SLE patients were selected for study.Citation1 Anti-p300 antibodies, as well as anti-GST antibody as negative control, were used for immunoprecipitation. PCR was performed to evaluate for differential target gene p300 recruitment.
We found that in D54MG cells with IRF1 knockdown (), there was significantly (P ≤ 0.05) decreased p300 binding in the several target genes: TAP1, TNFRSF14, IFIH1, and SERPINE1 (). illustrates results from complementary experiments in D54MG cells stably transfected by an IRF1 overexpression vector, normalized to its control empty vector. Significantly (P ≤ 0.05) increased p300 binding was seen in several target genes (TDP2, TAP1, and SERPINE1) in the cells with increased IRF1 expression. Control ChIP assays were done for both conditions using anti-GST antibody, which is a non-binding antibody. shows representative results from the control experiments in IRF1 overexpressing cells, normalized to control.
Figure 7. The effect of IRF1 on the recruitment of p300 to target genes. (A) D54MG cells were stably transfected with an IRF1 siRNA to knock-down IRF1 expression or a control empty vector. The cells were chromatin immunoprecipitated with antibodies to p300 or GST (a non-binding control antibody). The effect of IRF1 expression on the recruitment of p300 to target genes was examined. The target genes (INHBA, TDP2, TAP1, TNFRSF14, IFIH1, and SERPINE1) were selected for both increased IRF1 binding and mRNA expression in SLE patients.Citation1 GAPDH is a control gene. The qRT-PCR results (n = 6 pairs) from the IRF1 knockdown cells were normalized to the vector-control cells and expressed as fold-change differences. Significantly (* indicates P ≤ 0.05) decreased p300 binding in several target genes (TAP1, TNFRSF14, IFIH1, and SERPINE1) was seen in the cells with decreased IRF1 expression. (B) D54MG cells were stably transfected with an IRF1 overexpression vector or a control empty vector. Anti-p300 ChIP assays were performed as above. The qRT-PCR results (n = 4 pairs) from the IRF1 overexpression cells were normalized to the vector-control cells and expressed as fold-change differences. Significantly (* indicates P ≤ 0.05) increased p300 binding in several target genes (TDP2, TAP1, and SERPINE1) was seen in the cells with increased IRF1 expression. (C) A representative anti-GST ChIP is shown as a control using a non-binding antibody.
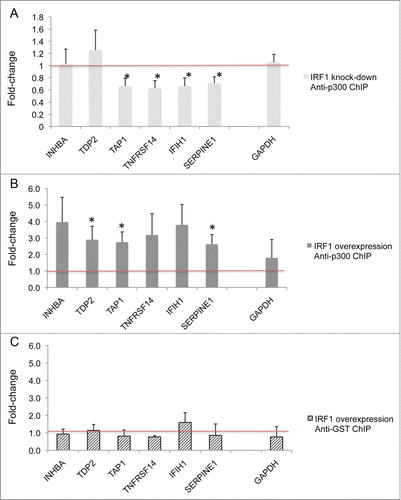
Discussion
This is the first analysis of global histone acetylation in SLE evaluating multiple H4 lysines. We found evidence that multiple lysines were hyperacetylated, which was consistent with multiple HATs or a broadly functioning HAT driving acetylation in SLE. Specifically, increased H4K5ac has been implicated in expression bookmarking,Citation12 where a previously expressed gene is more competent for subsequent expression. H4K8 is acetylated by a distinct group of HATsCitation13 and it is typically read by the SWI/SNF complex not the CBP/p300 complex.Citation14 Its role is primarily in transcriptionally active genes, possibly contributing to elongation.Citation15 H4K12ac is localized at promotersCitation16 and may play a role in the developmental regulation of gene expression.Citation17 H4K16 is globally reduced in cancerCitation18 and it thought to play a role in higher order chromatin conformation.Citation19 All 4 modifications are associated with transcriptional activity.
This study explored the role of IRF1 in the hyperacetylation of H4 in SLE patients. We showed that IRF1 overexpression was associated with globally increased H4 acetylation. This process is favored by an imbalance in the HAT:HDAC dynamic, promoting H4 acetylation.
Histone acetylation genomic patterns are established through the balance of active HATs and HDACs, both globally and at specific regulatory sequences. The activated IRF1 protein has short half-life of 30 min,Citation20 consequently its associations with chromatin modifier enzymes are transient. Our co-immunoprecipitation studies illustrate these dynamic interactions of IRF1 with CBP, p300, and GCN5, which were seen at 1 h after IRF1 activation and reversed by 4 h. This suggests that while the activated IRF1 protein has a short half-life, the interactions are dynamic and IRF1 is continually activated in the presence of IFN-α. IRF1 binding with PCAF and ATF2 appeared to be constitutive. Studies have previously reported that IRF1 is part of a protein complex that includes PCAF, GCN5 and CBP/p300.Citation21,22 PCAF directly binds IRF1 and enhances IRF1-dependent promoter activity, leading to increase in IFN-a-inducible promoter activity. PCAF interacts directly with p300/CBP. Thus, IRF1 can recruit the PCAF and CBP/p300 complex to acetylate H4.Citation21 ATF2 also interacted with IRF1 and ATF2 phosphorylation has been shown to precede H4 acetylation.Citation23 The association between IRF1 and HDAC3 may target genes for down-regulation but HDAC3 is also known to be involved in macrophage polarizationCitation24 and HDACs can deacetylate transcription factors such as NFκBCitation25 and STATs,Citation26 providing for a potential alternative role for this interaction.
We demonstrated that IRF1 interacted with multiple HATs that can broadly increase acetylation at all H4 lysine residues. In vitro overexpression IRF1 was able to produce a pattern of hyperacetylation at H4 lysine residues similar to that in seen in SLE. Furthermore, IRF1 overexpression was associated with increased targeting of p300 to known IRF1 targets. IRF1 knockdown, in contrast, was associated with diminished p300 recruitment to known IRF1 targets. The genes impacted by the IRF1 overexpression and knockdown were not concordant and this may be due to the time point that we selected or to stabilization by other transcription factors. Our hypothesis is that some portion of the dysregulated gene expression seen in SLE is not due to specific ongoing stimulation, but is a result of globally aberrant chromatin modifications that facilitate and perpetuate chronic inflammation. This has extremely important implications for the chronicity of the disease process. These studies link an initial stimulus that drives a type I IFN response with activation of IRF1, recruitment of HATs to specific IRF1-inducible target genes and a durably altered set point for expression. These data provide an important mechanistic insight into the dysregulated epigenome in SLE and may direct novel therapeutics.
Disclosure of Potential Conflicts of Interest
No potential conflicts of interest were disclosed.
Acknowledgments
We are grateful to patients, volunteers, nurses and physicians for their contribution and support.
Funding
This study was supported in part by a generous donation from the Irvin Siegel Lupus Research Fund, the Wallace Chair of Pediatrics, and NIH grants R01 ES017627 and AR43727, The Alliance for Lupus Research and the Postdoctoral Fellowship from the Arthritis Foundation. This project was supported by funding from the Arthritis Foundation (YTL), NIH AR43727 (MP), NIH R01ES017627 and The Alliance for Lupus Research (KES).
References
- Zhang Z, Song L, Maurer K, Petri MA, Sullivan KE. Global H4 acetylation analysis by ChIP-chip in systemic lupus erythematosus monocytes. Genes Immun 2010; 11:124-33; PMID:19710693; http://dx.doi.org/10.1038/gene.2009.66
- Ogasawara K, Hida S, Azimi N, Tagaya Y, Sato T, Yokochi-Fukuda T, Waldmann TA, Taniguchi T, Taki S. Requirement for IRF-1 in the microenvironment supporting development of natural killer cells. Nature 1998; 391:700-3; PMID:9490414; http://dx.doi.org/10.1038/35636
- Kano S, Sato K, Morishita Y, Vollstedt S, Kim S, Bishop K, Honda K, Kubo M, Taniguchi T. The contribution of transcription factor IRF1 to the interferon-gamma-interleukin 12 signaling axis and TH1 versus TH-17 differentiation of CD4+ T cells. Nat Immunol 2008; 9:34-41; PMID:18059273; http://dx.doi.org/10.1038/ni1538
- Fangtham M, Petri M. 2013 update: Hopkins lupus cohort. Curr Rheumatol Rep 2013; 15:360; PMID:23888367; http://dx.doi.org/10.1007/s11926-013-0360-0
- Shi L, Zhang Z, Yu AM, Wang W, Wei Z, Akhter E, Maurer K, Costa Reis P, Song L, Petri M, et al. The SLE Transcriptome Exhibits Evidence of Chronic Endotoxin Exposure and Has Widespread Dysregulation of Non-Coding and Coding RNAs. PloS one 2014; 9:e93846; PMID:24796678; http://dx.doi.org/10.1371/journal.pone.0093846
- Garrett S, Dietzmann-Maurer K, Song L, Sullivan KE. Polarization of primary human monocytes by IFN-gamma induces chromatin changes and recruits RNA Pol II to the TNF-α promoter. J Immunol 2008; 180:5257-66; PMID:18390706; http://dx.doi.org/10.4049/jimmunol.180.8.5257
- Garrett S, Fitzgerald MC, Sullivan KE. LPS and poly I:C induce chromatin modifications at a novel upstream region of the IL-23 p19 promoter. Inflammation 2008; 31:235-46; PMID:18587636; http://dx.doi.org/10.1007/s10753-008-9070-6
- Gasper WC, Marinov GK, Pauli-Behn F, Scott MT, Newberry K, DeSalvo G, Ou S, Myers RM, Vielmetter J, Wold BJ. Fully automated high-throughput chromatin immunoprecipitation for ChIP-seq: identifying ChIP-quality p300 monoclonal antibodies. Sci Rep 2014; 4:5152; PMID:24919486; http://dx.doi.org/10.1038/srep05152
- Peserico A, Simone C. Physical and functional HAT/HDAC interplay regulates protein acetylation balance. J Biomed Biotechnol 2011; 2011:371832; PMID:21151613; http://dx.doi.org/10.1155/2011/371832
- Bhaumik SR, Smith E, Shilatifard A. Covalent modifications of histones during development and disease pathogenesis. Nat Struct Mol Biol 2007; 14:1008-16; PMID:17984963; http://dx.doi.org/10.1038/nsmb1337
- McManus KJ, Hendzel MJ. Quantitative analysis of CBP- and P300-induced histone acetylations in vivo using native chromatin. Mol Cell Biol 2003; 23:7611-27; PMID:14560007; http://dx.doi.org/10.1128/MCB.23.21.7611-7627.2003
- Zhao R, Nakamura T, Fu Y, Lazar Z, Spector DL. Gene bookmarking accelerates the kinetics of post-mitotic transcriptional re-activation. Nat Cell Biol 2011; 13:1295-304; PMID:21983563; http://dx.doi.org/10.1038/ncb2341
- Larschan E, Winston F. The S. cerevisiae SAGA complex functions in vivo as a coactivator for transcriptional activation by Gal4. Genes Dev 2001; 15:1946-56; PMID:11485989; http://dx.doi.org/10.1101/gad.911501
- Agalioti T, Chen G, Thanos D. Deciphering the transcriptional histone acetylation code for a human gene. Cell 2002; 111:381-92; PMID:12419248; http://dx.doi.org/10.1016/S0092-8674(02)01077-2
- Cho H, Orphanides G, Sun X, Yang XJ, Ogryzko V, Lees E, Nakatani Y, Reinberg D. A human RNA polymerase II complex containing factors that modify chromatin structure. Mol Cell Biol 1998; 18:5355-63; PMID:9710619
- Wang Z, Zang C, Rosenfeld JA, Schones DE, Barski A, Cuddapah S, Cui K, Roh TY, Peng W, Zhang MQ, et al. Combinatorial patterns of histone acetylations and methylations in the human genome. Nat Genet 2008; 40:897-903; PMID:18552846; http://dx.doi.org/10.1038/ng.154
- Paradowska AS, Miller D, Spiess AN, Vieweg M, Cerna M, Dvorakova-Hortova K, Bartkuhn M, Schuppe HC, Weidner W, Steger K. Genome wide identification of promoter binding sites for H4K12ac in human sperm and its relevance for early embryonic development. Epigenetics 2012; 7:1057-70; PMID:22894908; http://dx.doi.org/10.4161/epi.21556
- Fraga MF, Ballestar E, Villar-Garea A, Boix-Chornet M, Espada J, Schotta G, Bonaldi T, Haydon C, Ropero S, Petrie K, et al. Loss of acetylation at Lys16 and trimethylation at Lys20 of histone H4 is a common hallmark of human cancer. Nat Genet 2005; 37:391-400; PMID:15765097; http://dx.doi.org/10.1038/ng1531
- Shogren-Knaak M, Ishii H, Sun JM, Pazin MJ, Davie JR, Peterson CL. Histone H4-K16 acetylation controls chromatin structure and protein interactions. Science 2006; 311:844-7; PMID:16469925; http://dx.doi.org/10.1126/science.1124000
- Watanabe N, Sakakibara J, Hovanessian AG, Taniguchi T, Fujita T. Activation of IFN-β element by IRF-1 requires a posttranslational event in addition to IRF-1 synthesis. Nucleic Acids Res 1991; 19:4421-8; PMID:1886766; http://dx.doi.org/10.1093/nar/19.16.4421
- Masumi A, Wang IM, Lefebvre B, Yang XJ, Nakatani Y, Ozato K. The histone acetylase PCAF is a phorbol-ester-inducible coactivator of the IRF family that confers enhanced interferon responsiveness. Mol Cell Biol 1999; 19:1810-20; PMID:10022868
- Merika M, Williams AJ, Chen G, Collins T, Thanos D. Recruitment of CBP/p300 by the IFN β enhanceosome is required for synergistic activation of transcription. Mol Cell 1998; 1:277-87; PMID:9659924; http://dx.doi.org/10.1016/S1097-2765(00)80028-3
- Bruhat A, Cherasse Y, Maurin AC, Breitwieser W, Parry L, Deval C, Jones N, Jousse C, Fafournoux P. ATF2 is required for amino acid-regulated transcription by orchestrating specific histone acetylation. Nucleic Acids Res 2007; 35:1312-21; PMID:17267404; http://dx.doi.org/10.1093/nar/gkm038
- Mullican SE, Gaddis CA, Alenghat T, Nair MG, Giacomin PR, Everett LJ, Feng D, Steger DJ, Schug J, Artis D, et al. Histone deacetylase 3 is an epigenomic brake in macrophage alternative activation. Genes Dev 2011; 25:2480-8; PMID:22156208; http://dx.doi.org/10.1101/gad.175950.111
- Yu Z, Zhang W, Kone BC. Histone deacetylases augment cytokine induction of the iNOS gene. J Am Soc Nephrol 2002; 13:2009-17; PMID:12138131; http://dx.doi.org/10.1097/01.ASN.0000024253.59665.F1
- Kramer OH, Knauer SK, Greiner G, Jandt E, Reichardt S, Guhrs KH, Stauber RH, Bohmer FD, Heinzel T. A phosphorylation-acetylation switch regulates STAT1 signaling. Genes Dev 2009; 23:223-35; PMID:19171783; http://dx.doi.org/10.1101/gad.479209