ABSTRACT
Insufficient activity of the bone-forming osteoblasts leads to low bone mass and predisposes to fragility fractures. The functional capacity of human mesenchymal stem cells (hMSCs), the precursors of osteoblasts, may be compromised in elderly individuals, in relation with the epigenetic changes associated with aging. However, the role of hMSCs in the pathogenesis of osteoporosis is still unclear. Therefore, we aimed to characterize the genome-wide methylation and gene expression signatures and the differentiation capacity of hMSCs from patients with hip fractures. We obtained hMSCs from the femoral heads of women undergoing hip replacement due to hip fractures and controls with hip osteoarthritis. DNA methylation was explored with the Infinium 450K bead array. Transcriptome analysis was done by RNA sequencing. The genomic analyses revealed that most differentially methylated loci were situated in genomic regions with enhancer activity, distant from gene bodies and promoters. These regions were associated with differentially expressed genes enriched in pathways related to hMSC growth and osteoblast differentiation. hMSCs from patients with fractures showed enhanced proliferation and upregulation of the osteogenic drivers RUNX2/OSX. Also, they showed some signs of accelerated methylation aging. When cultured in osteogenic medium, hMSCs from patients with fractures showed an impaired differentiation capacity, with reduced alkaline phosphatase activity and poor accumulation of a mineralized matrix. Our results point to 2 areas of potential interest for discovering new therapeutic targets for low bone mass disorders and bone regeneration: the mechanisms stimulating MSCs proliferation after fracture and those impairing their terminal differentiation.
Introduction
Osteoporotic hip fractures have a particularly negative impact on patient quality of life and life expectancy. The pathogenetic mechanisms of osteoporosis are not completely understood, but they imply an imbalance in bone remodeling, with a predominance of bone resorption over bone formation. Osteoblasts are the cells responsible for bone formation. Human mesenchymal stem cells (hMSCs) are pluripotent cells capable of differentiating into osteoblasts, chondrocytes, adipocytes, and myoblasts. Therefore, hMSCs are the subject of considerable interest from a double perspective. On the one hand, hMSC malfunction may be involved in the pathogenesis of osteoporosis.Citation1,2 Thus, elucidating the mechanisms involved in hMSCs proliferation and differentiation could point to new drug targets to improve osteoblast function in osteoporotic patients. On the other hand, the infusion of hMSCs could theoretically improve bone formation systemically or locally. A few studies tried to characterize hMSCs from patients with osteoporosis, but the results are controversial.Citation3-5 Therefore, much more basic research is needed to characterize hMSCs in osteoporosis before using, them for the treatment of skeletal disorders.
Epigenetic mechanisms play an important role in skeletal biology. In particular, DNA methylation has been shown to modulate the differentiation of cells toward the osteoblastic lineage.Citation6,7 Thus, it is tempting to speculate that changes in DNA methylation influence the differentiation capability of hMSCs in osteoporosis. However, there are only scarce data about the methylation signature of skeletal hMSCs. Therefore, we aimed to perform a genome-wide methylome analysis of hMSCs from patients with hip fractures and explore the functional consequences at the transcriptome level and the ability to proliferate and differentiate in vitro.
Results
Isolation and characterization of hMSCs
We had similar success rate in establishing hMSC cultures with samples obtained from patients with fractures (27 out of 41, 66%) or with osteoarthritis (OA; 22 out of 32, 69%). The hMSC phenotype was confirmed by flow cytometry using a combination of markers (CD45−, CD34−, CD90+, CD73+, CD105+) that define the phenotype of bone marrow hMSCs (Fig. S1).Citation2
DNA methylation profiling
When the interrogated CpGs were grouped according to their genomic position, gene bodies were more methylated than CpG islands or gene promoters (Figs. S2 and S3). The average methylation level was similar in both study groups. Nevertheless, among the 477,708 sites explored, we found 9,038 differentially methylated CpG sites. Of them, 4,417 were more methylated and 4,621 were less methylated in samples obtained from osteoporotic patients with hip fracture (FRX). Among the differentially methylated CpG sites, 1,586 sites were located in CpG islands, 1,105 in shores, 353 in shelves, and 5,994 sites in open sea.
The analysis at the region level revealed 217 out of 30,877 gene promoters with differential methylation, which correspond to 71 protein coding genes, of a total of 21,191 different genes, including 111 more methylated and 106 less methylated in FRX. Regarding gene bodies, 62 were found more methylated and 67 less methylated in FRX. Among CpG islands, 16 were more methylated and 24 less methylated in FRX ( and Fig. S4).
Table 1. Distribution of differentially methylated CpGs between MSCs from fracture patients (FRX) and patients with osteoarthritis in various genomic regions.
Differentially methylated sites were enriched in enhancer regions (which included 2,425 of the 9,038 differentially methylated CpGs). The distances to the transcription start sites (TSSs) are shown in Fig. S5. The region level analysis revealed 1,684 differentially methylated gene enhancer regions; 870 regions (associated with 722 protein-coding genes) were hypermethylated and 814 (678 genes) were hypomethylated in FRX in comparison with OA (Table S1 and ). Most FRX and OA samples tended to be grouped in common clusters, but there was some degree of overlapping, as shown in . Genes with differentially methylated enhancers were overrepresented among pathways related to stem cell development and bone-related pathways, like the Wnt receptor signaling pathway (P = 4.5×10−8; binomial test), regulation of osteoblast differentiation (P = 9.1×10−5), regulation of hMSCs proliferation (P = 7.6×10−6), and bone mineralization (P = 7.6×10−4) pathways (Fig. S6).
Figure 1. Methylation analysis of hMSCs. (A) Volcano plot of DNA methylation differences in enhancer regions of hMSCs obtained from fractures and OA. In green, sites with a FDR<0.05 and absolute β differences larger than 0.1 (B) Heat-map showing β values of enhancer regions. In red more methylated and in green less methylated. Samples are named with a laboratory identifier code (JAR).
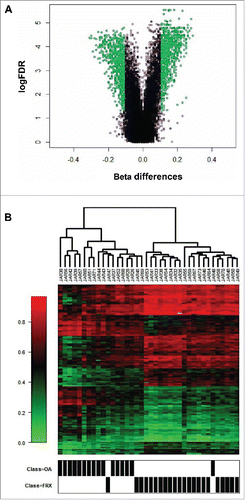
Gene expression analysis
Overall, 11,390 genes were expressed in both FRX and OA samples, whereas 496 genes were expressed only in FRX and 1,695 in OA. As expected, the gene expression signature was typical of hMSCs (Table S2). Overall, 99 protein-coding genes were upregulated in FRX (FDR<0.10 and fold-change>2), whereas 239 were downregulated (Fig. S7 and Table S3). The top 50 up- or down-regulated genes are shown in .
Table 2. Top 50 upregulated genes in fractures. Gene symbol and complete gene name are shown. Each gene with their corresponding fold change value and corrected P-value (FDR).
Table 3. Top 50 downregulated genes in fractures. Gene symbol and complete gene name are shown. Each gene with their corresponding fold change value and corrected P-value (FDR).
Among genes with differential expression, those upregulated in FRX were enriched in pathways related to hMSCs differentiation and bone formation, whereas those downregulated in FRX were enriched in pathways related to the immune response, among others (Table S4).
Correlation between differential DNA methylation and differential gene expression
Genes with differentially methylated enhancers were overrepresented among those showing differential expression (19.8% vs. 9.2% in those without differential expression; P = 1.2×10−10). The direction of the association was variable, but there was a trend for an inverse correlation between enhancer methylation and expression [odds ratio (OR): 0.3; 95% confidence interval: 0.12–0.99; P = 0.05]. This is schematically depicted in , showing that 18 genes upregulated in FRX had differentially methylated enhancers: 8 hypermethylated and 10 hypomethylated. On the other hand, 55 genes downregulated in FRX had differentially methylated enhancers: 39 hypermethylated and 16 hypomethylated. Examples of the individual values of expression and methylation of some genes are shown in Fig. S8. The comparative Gene Ontology Enrichment analysis revealed that genes with hypomethylated enhancers and upregulated expression in fractures were overrepresented in pathways related to hMSCs proliferation, osteoblast differentiation, and bone mineralization, as well as some neuron-related pathways ( and Fig. S9).
Figure 2. Relationship between methylation and gene expression signatures. (A) Venn diagram summarizing the association between differential DNA methylation and differential gene expression (comparisons of hMSCs from fractures over hMSCs from controls). (B) Pathways enrichment analysis of genes with hypomethylated enhancers that were upregulated in fractures.
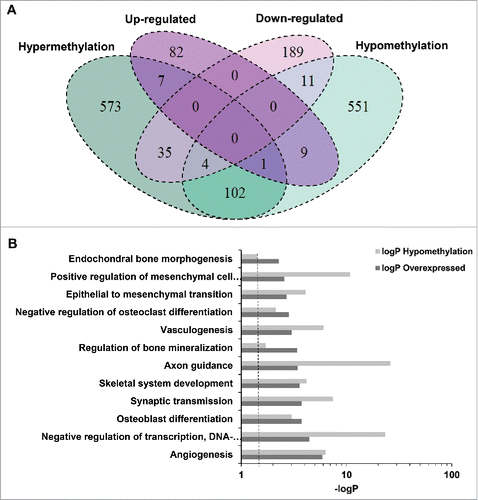
Validation of expression and methylation differences across groups
We confirmed, by qPCR, the expression pattern of 10 genes among those that showed differential expression in the RNAseq analysis (SPARC, LOXL2, FOXP2, LAMC1, SLC5A3, OPG, ID2, LASP1, IGFBP4, and UNC5B), including 8 samples previously analyzed by RNAseq and 19 new samples. There were strong correlations in the individual results obtained with both techniques (Figs. S10 and S11). Similarly, to validate the results obtained with the 450K array, we analyzed, by pyrosequencing, 4 genes showing differential methylation according to array data analysis (Fig. S12).
DNA methylation age
We studied the DNA methylation aging in our samples by using software based on Illumina DNA Infinium 450K data that analyzes a set of genes showing age-related changes in methylation. There was a significant correlation between the epigenetic age and the chronological age (r = 0.64, P = 1.36 × 10−5). The slopes of the regression lines were similar in both patient groups (, left panel), but the lines were vertically displaced. Therefore, when the regression was computed with both groups combined, the deviations of the epigenetic age from the chronological age were higher in the FRX group and negative in the OA group, thus suggesting accelerated epigenetic aging in the former (, right panel).
Proliferative capability of hMSCs
Interestingly, the proportion of actively dividing, Ki-67 positive cells was significantly higher among hMSCs grown from patients with FRX (). These results agreed with those obtained from a MTT assay, which confirmed a higher proliferation rate in hMSCs cultures established from patients with fractures ().
Figure 4. Proliferation capacity and expression of selected genes by hMSCs from patients with fractures (FRX) and osteoarthritis (OA). (A) Proliferation assessed by Ki67 staining. (B) Proliferation by a MTT assay. (C) Expression of osteogenic markers by hMSCs from FRX and OA. (D) Expression of adipogenic markers by hMSCs.
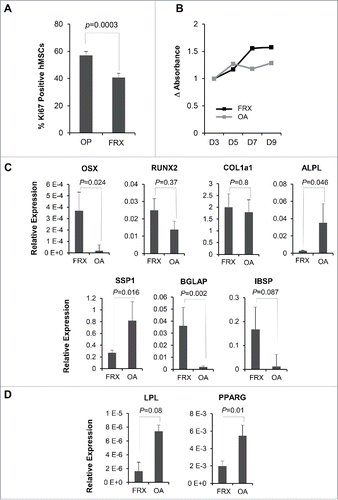
Transcriptional signature and bone differentiation capacity of hMSCs
Analysis of the gene expression levels of a set of osteogenic and adipogenic markers showed significant differences in the expression levels of key osteogenic genes (), such as OSX, ALPL, SPP1, and BGLAP, between patients with osteoporotic fractures and OA. These data agreed and validated the previously shown results from the RNAseq analysis. Interestingly, OSX, a key transcription factor expressed in the first stages of osteoblast development, was significantly upregulated in hMSCs from patients with FRX, whereas collagen expression was similar in both groups. Markers, such as ALPL and SPP1, normally expressed later on during the osteogenic differentiation process, were downregulated in hMSCs isolated from patients with osteoporotic fractures. On the other hand, adipogenic markers, such as PPARG and LPL, were expressed less abundantly in patients with fractures than in those with OA ().
In line with their hMSC phenotype, the cells were able to differentiate normally into adipocytes and osteoblasts (). However, there were marked differences between groups and the capacity to form a mineralized matrix was markedly diminished in hMSCs from patients with FRX (P = 0.00015; ). The alkaline phosphatase activity in hMSC cultures from FRX was also much lower than in cultures of OA origin (), and there was a correlation between alkaline phosphatase activity and matrix mineralization (Spearman's rho 0.84, P < 0.001). On the other hand, there was a tendency for enhanced OSX and RUNX2 expression in FRX cells ().
Figure 5. Differentiation capacity of hMSCs. (A) Osteogenic and adipogenic differentiation of hMSCs, as revealed by Alizarin red staining and Oil red staining, respectively. (B) Osteogenic differentiation of hMSCs from patients with fractures (FRX) and with osteoarthritis (OA), semiquantitative analysis. (C) Alkaline phosphatase activity in hMSC maintained in osteogenic medium. (D) Expression of the osteoblastic differentiation drivers OSX and RUNX2 in hMSCs from patients with FRX and OA maintained in osteogenic medium.
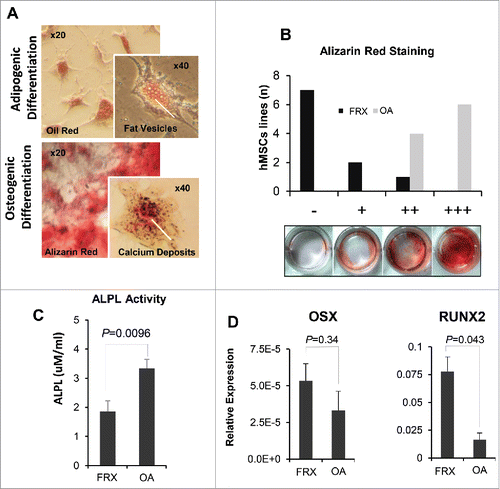
Discussion
We have previously shown that modifications in DNA methylation play a central role in the differentiation of cells along the osteoblastic lineage and that genes related to skeletal development are differentially methylated in bone samples of patients with osteoporotic fractures.Citation8 In the present study, we explored the epigenome of hMSCs in patients with fractures. To our knowledge, this is the first study combining epigenome-wide and transcriptome-wide analyses of hMSCs in osteoporosis. We established that the methylation signatures of hMSCs from patients with fractures and OA show significant differences at the enhancer regions of several genes related to cell proliferation and differentiation.
Our study has some limitations. The method used does not allow distinguishing methylated and hydroxymethylated cytosines. Due to practical reasons, we isolated hMSCs from the bone marrow of OA patients rather than normal bone marrow as comparison controls. Nevertheless, it has been suggested that MSCs from OA patients have similar proliferation and osteogenic differentiation capacity to MSCs from healthy donors.Citation9 Also, we removed the subchondral region, trying to avoid the potential influence of the changes taking place in the subchondral bone.Citation10 Aging is associated with methylation differences in some genesCitation11 and patients with fractures were somewhat older than those with OA. Nevertheless, we adjusted the results including age as a covariate to avoid any age-related bias. Also, due to the low percentage of hMSCs in bone marrow (less than 1/10,000 to 1/100,000) hMSCs needed to be expanded in vitro before the analysis. However, to avoid changes in the epigenome signature that could bias the results, in vitro expansion was kept to a minimum and only cells at first passage were used for the analyses.
The classical view of DNA methylation tended to associate increased methylation with decreased gene expression and vice versa. However, our results confirm that the relationship between methylation and expression is indeed variable. Although increased methylation was associated with a decreased expression of many genes, the opposite was also true. In fact, that seems to be the case in other cell types.Citation12,13 Additionally, as previously reported in other conditions, including the osteoarthritic cartilage,Citation14,15 most differentially methylated regions do not appear to be at the gene bodies or at promoter regions, but at genomic regions with enhancer activity distant from the promoter. Thus, changes in methylation at regulatory regions may influence the expression of genes situated several hundred kilobases away. Also, as shown in our genomic region analysis, most regions showing differential methylation between groups appear to be outside CpG islands.
Rather unexpectedly, hMSCs from patients with hip fractures tended to display upregulation of genes linked to cell proliferation pathways, in parallel with a decreased methylation of their enhancer regions. Those genes were overrepresented in important pathways, such as those regulating cell proliferation, osteoblast differentiation, or vasculogenesis. This translated into an increased proliferation of these cells, in comparison with cells from patients with OA, in line with the concept that fractures activate and mobilize hMSCs and are consistent with previous reports showing a mobilization of MSCs into the circulation in response to fracture.Citation16 The mechanisms involved remain to be elucidated but may include the release of activating cytokines and activation of the Wnt pathway, as suggested by our enrichment analysis. Wnt ligands promote the proliferation of osteoblasts precursors.Citation17,18 In line with this, Baht et al. recently reported that haematopoietic cells of young mice are able to stimulate the osteoblastic responses in aged animals by a mechanism that involves β-catenin, a critical signaling mediator of the canonical Wnt pathway.Citation19 Neural-mediated mechanisms may also be included, as suggested by the enhanced bone formation shown by patients with head trauma CitationCitation20 and the differential methylation and expression of some genes in nervous tissue-related pathways also found in the present study.
MSCs from fracture patients showed upregulation of genes driving osteogenic differentiation, such as RUNX2 and OSX. The expression of some genes, such as BGLAP and IBSP, usually considered as characteristic of the osteoblastic phenotype and targets of RUNX2, was also increased in hMSCs from fracture patients in basal conditions. However, those hMSCs showed a reduced ability to form a mineralized matrix when cultured in osteogenic medium. This could be related to a decreased activity of alkaline phosphatase, which plays a critical role in mineralization, or to the persistent upregulation of RUNX2. RUNX2 is a master driver of osteoblast precursor differentiation.Citation21 Specific epigenetic marks contribute to the regulation of RUNX2 expression.Citation22 RUNX2 controls bone development and osteoblast differentiation by regulating the expression of a significant number of bone-related target genes, including collagen, osteocalcin, osteopontin, bone sialoprotein, and osteoprotegerin. Thus, it plays a critical role in the early stages of differentiation of hMSCs into pre-osteoblasts. However, after this initial step, RUNX2 expression must be turned down to allow terminal differentiation of osteoblasts.Citation23
Recent experiments in rodent models of osteoporosis have shown that the MSC transplantation may have a positive effect on bone mass locally and even systemically.Citation24,25 Additionally, molecules helping to targeted delivery MSCs into bone are being developed.Citation26 This raises the possibility of using MSCs for bone regeneration, fracture consolidation, or treating widespread bone loss. Since allogenic transplants of MSCs pose difficulties related to histocompatibility, in clinical practice autologous transplants would be preferable. Remarkably, our results are encouraging because they show that, despite some accelerated epigenetic aging, hMSCs from patients with fragility fractures are activated and maintain a good proliferation capacity, rendering them potentially useful for autologous transplants. Indeed, these cells were able to express genes encoding several proteins present in bone matrix in quantities similar to those expressed by cells from OA patients, which have been reported to behave similarly to MSCs from healthy subjects.Citation9 However, their ability for terminal differentiation and the formation of a mineralized matrix appears to be compromised, at least in vitro. Hence, it would be highly desirable to reach a better understanding of the mechanisms impairing that ability to circumvent them with the final objective of optimizing bone matrix formation in vivo.
In summary, the epigenome-wide signature of hMSCs from fracture patients shows differentially methylated regions in comparison with hMSCs derived from OA patients. Most differences are in genomic regions with enhancer activity, distant from gene bodies and apart from CpG islands. They are associated with upregulation of several genes involved in MSC proliferation and differentiation, including upregulation of genes such as RUNX2/OSX driving the differentiation toward an osteoblastic phenotype. However, their ability to form a mineralized matrix appears to be impaired. These results point to 2 areas of potential interest for discovering new therapeutic targets for low bone mass disorders and bone regeneration: the mechanisms stimulating MSCs after fracture and the mechanisms impairing the terminal differentiation of MSCs. Additionally, these results emphasize the concept that modifications of DNA methylation may have a cis-influence on rather distant genes.
Materials and methods
Human mesenchymal stem cells (hMSCs) isolation and culture
Bone marrow hMSCs were obtained from the femoral heads of patients undergoing replacement surgery due to osteoporotic hip fractures (FRX; age 62 to 88 years) or hip osteoarthritis (OA; age: 72 to 92 years). They included female patients with osteoporotic hip fractures or hip osteoarthritis. Patients with high-impact fractures, secondary osteoporosis, or secondary OA were excluded. All patients gave informed written consent. The study protocol was approved by the institutional review board (Comité de Ética en Investigación Clínica de Cantabria). Cylinders of trabecular bone were extracted with a trephine, after removing the subchondral and subfracture edges, they were washed with 50 mL of phosphate-buffered saline (PBS). Cells were subjected to a density Ficoll gradient. Then, 2 × 10Citation6 per cmCitation2 were cultured on polystyrene culture flasks in Mesencult™ MSC Basal media completed with 10% of Mesenchymal Stem Cell Stimulatory supplements (Stem Cell Technologies®, Vancouver, Canada). Representative samples were characterized by staining for surface markers in a FACSCanto II flow cytometer (Becton Dickinson, New Jersey, USA) after labeling with antibodies against CD45, CD34, CD73, CD90, and CD105.
Genome-wide methylation analysis
Since only MSCs at first passages were used for the experiments, there was a limited number of cells to perform all experiments with some of the hMSC lines. Thus, only 22 FRX and 17 OA samples of all the samples initially harvested were used for the analyses of DNA methylation (patients age: 62 to 95 years). DNA was extracted with phenol:chloroform:isoamyl alcohol and bisulfite-converted before genome-wide analysis of methylation with the Infinium Human Methylation450 BeadChip array (Illumina®, San Diego, CA, USA) in the Spanish “Centro Nacional de Genotipado” (CEGEN-ISCIII).
Raw data files were pre-processed using R/Bioconductor package RnBeads, CitationCitation27 and methylation was described as β value, which ranges between 0 (no methylation) to 1 (full methylation). Analyses were conducted at the single CpG site level and at various region levels, including age as a covariate. Regions with FDR < 0.05 and an absolute difference in methylation higher than 10% (Δβ > 0.10) were considered as differentially methylated. Annotation data for the genomic enhancers study were retrieved from the H1 embryonic stem cell line from the UCSC Table Browser.
The methylation level of selected CpGs was replicated by pyrosequencing (PyromarkQ24 Advanced System®). Primers used for PCR amplification and sequencing were designed with the PyroMark assay designer (Table S5). The statistical significance of the differences between FRX and OA patients was tested by Mann-Whitney tests, with a significance threshold of 0.05.
Transcriptome analysis
RNA was isolated from hMSCs (n = 10 FRX and n = 10 OA) using Trizol® (Thermofisher Scientific, Waltham, MA, USA). After quality check and quantitation, the samples were prepared using the NEBNext Ultra Directional RNA Library Protocol. Samples were sequenced on an Illumina Hi-seq 2,000 sequencer (NTX-Dx, Gent, Belgium). Sample reads were mapped to the human reference genome build GRCh37. Expression analysis was done with cufflinks v2.1.1 on Gencode Annotation v15. FPKM values were calculated for each annotated gene and transcript. RNAseq analysis was performed at the transcript level with Altanalyze software CitationCitation28 and at the gene level with the Bioconductor package EdgeR.Citation29 Genes with FDR < 0.10 and fold-changes > 2 were regarded as differentially expressed.
Enrichment analyses of the genes with differentially-methylated enhancers were obtained from the output of GREAT software.Citation30 The overrepresentation of genes with differential expression in different cell pathways (Wikipathways) was obtained from the output of WEBGESTALT software,Citation31 which incorporates information from different public resources. The Gene Ontology enrichment analyses from the common terms between differentially methylated enhancers and differential expression were done with ArrayTrack software.Citation32
RNAseq data and expression of selected genes were confirmed by using real-time quantitative PCR (qPCR), after reverse-transcription of RNA into cDNA, using the housekeeping genes GAPDH and RPL13A for normalization. Assay details are given in Table S5.
DNA methylation age
The epigenetic age was calculated from the methylation level of a set of 353 CpGs, which has been shown to change with aging in a wide variety of tissues.Citation33 The relationship of the epigenetic age and the chronological age was explored by linear regression analysis.
Proliferation analysis
The proliferation status of hMSCs was assessed by immunocytochemistry using an anti-Ki-67 antibody. Ki-67 is a nuclear protein associated with cell proliferation. The results were confirmed by a cell proliferation colorimetric assay based on the reduction of the tetrazolium dye MTT.
Osteogenic and adipogenic differentiation
hMSCs were cultured in osteogenic induction medium (low glucose Dulbecco Modified Eagle Medium (DMEM) with 10% FBS, 50 µM ascorbic acid, 10 mM β-glycerophosphate, 100 nM dexamethasone) or adipogenic induction medium (DMEM, supplemented with 1.0 M dexamethasone, 0.2 mM indomethacin, 0.5 mM 3-isobutyl-1-methylxanthine, 10% FBS). hMSCs were plated in a 24-well plate and incubated for up to 3 weeks, before staining or RNA extraction. The formation of mineralized matrix was assessed by Alizarin Red staining. The staining was evaluated semiquantitatively by 2 independent observers who were blind of the culture origin. Other wells were used to determine alkaline phosphatase activity in cell lysates by a colorimetric method based on the ability of ALPL to hydrolyze p-nitrophenylphosphate.Citation34 Oil Red was used to visualize the presence of lipid droplets.
Disclosure of potential conflicts of interest
WVC and MB are employees of NTX, the company that performed RNA sequencing. Other authors do not have conflicts of interest relevant to this paper.
Author Contributions
Study design: JAR, FMPC; Clinical data Collection: CGI, MIPN, MAA. Experimental data collection: FMPC, AR, CS, WVC, MB, AFF. Data analysis and interpretation: WVC, MB, AR, FMPC, MFF, JAR. Drafting Manuscript: AR, FMPC, JAR; Revising Manuscript Content: AR, FMPC, JAR. Approving final version of manuscript: All authors. JAR takes responsibility for the integrity of the data analysis.
Supplementary_materials.zip
Download Zip (1.2 MB)Acknowledgment
We thank the skillful technical assistance of Jana Arozamena.
Funding
This study was supported by a grant from Instituto de Salud Carlos III (PI12/615), through a program potentially co-funded by FEDER Funds from the European Union. Carolina Sañudo was partially supported by IDIVAL. The methylation analysis service was performed at CEGEN-PRB2-ISCIII; it is supported by grant PT13/0001, ISCIII-SGEFI / FEDER
References
- Liu H, Xia X, Li B. Mesenchymal stem cell aging: Mechanisms and influences on skeletal and non-skeletal tissues. Exp Biol Med (Maywood) 2015; 240:1099-106; PMID:26088863; http://dx.doi.org/10.1177/1535370215591828
- Donoso O, Pino AM, Seitz G, Osses N, Rodríguez JP. Osteoporosis-associated alteration in the signalling status of BMP-2 in human MSCs under adipogenic conditions. J Cell Biochem 2015; 116:1267-77; PMID:25640452; http://dx.doi.org/10.1002/jcb.25082
- Haasters F, Docheva D, Gassner C, Popov C, Böcker W, Mutschler W, Schieker M, Prall WC. Mesenchymal stem cells from osteoporotic patients reveal reduced migration and invasion upon stimulation with BMP-2 or BMP-7. Biochem Biophys Res Commun 2014; 452:118-23; PMID:25152406; http://dx.doi.org/10.1016/j.bbrc.2014.08.055
- Liu W, Qi M, Konermann A, Zhang L, Jin F, Jin Y. The p53/miR-17/Smurf1 pathway mediates skeletal deformities in an age-related model via inhibiting the function of mesenchymal stem cells. Aging (Albany NY) 2015; 7:205-18; PMID:25855145; http://dx.doi.org/10.18632/aging.100728
- Prall WC, Haasters F, Heggebö J, Polzer H, Schwarz C, Gassner C, Grote S, Anz D, Jäger M, Mutschler W, et al. Mesenchymal stem cells from osteoporotic patients feature impaired signal transduction but sustained osteoinduction in response to BMP-2 stimulation. Biochem Biophys Res Commun 2013; 440:617-22; PMID:24099772; http://dx.doi.org/10.1016/j.bbrc.2013.09.114
- Delgado-Calle J, Sañudo C, Bolado A, Fernández AF, Arozamena J, Pascual-Carra MA, Rodriguez-Rey JC, Fraga MF, Bonewald L, Riancho JA. DNA methylation contributes to the regulation of sclerostin expression in human osteocytes. J Bone Miner Res 2012; 27:926-37; PMID:22162201; http://dx.doi.org/10.1002/jbmr.1491
- Perez-Campo F, Riancho JA. Epigenetic Mechanisms Regulating Mesenchymal Stem Cell Differentiation. Curr Genomics 2015; 16:368-83; PMID:27019612; http://dx.doi.org/10.2174/1389202916666150817202559
- Delgado-Calle J, Fernández AF, Sainz J, Zarrabeitia MT, Sañudo C, García-Renedo R, Pérez-Núñez MI, García-Ibarbia C, Fraga MF, Riancho JA. Genome-wide profiling of bone reveals differentially methylated regions in osteoporosis and osteoarthritis. Arthritis Rheum 2013; 65:197-205; PMID:23124911; http://dx.doi.org/10.1002/art.37753
- Stiehler M, Rauh J, Bünger C, Jacobi A, Vater C, Schildberg T, Liebers C, Günther K-P, Bretschneider H. In vitro characterization of bone marrow stromal cells from osteoarthritic donors. Stem Cell Res 2016; 16:782-9; PMID:27155399; http://dx.doi.org/10.1016/j.scr.2016.03.013
- Li G, Yin J, Gao J, Cheng TS, Pavlos NJ, Zhang C, Zheng MH. Subchondral bone in osteoarthritis: insight into risk factors and microstructural changes. Arthritis Res Ther 2013; 15:223; PMID:24321104; http://dx.doi.org/10.1186/ar4405
- Roforth MM, Farr JN, Fujita K, McCready LK, Atkinson EJ, Therneau TM, Cunningham JM, Drake MT, Monroe DG, Khosla S. Global transcriptional profiling using RNA sequencing and DNA methylation patterns in highly enriched mesenchymal cells from young versus elderly women. Bone 2015; 76:49-57; PMID:25827254; http://dx.doi.org/10.1016/j.bone.2015.03.017
- Shakhbazov K, Powell JE, Hemani G, Henders AK, Martin NG, Visscher PM, Montgomery GW, McRae AF. Shared genetic control of expression and methylation in peripheral blood. BMC Genomics 2016; 17:278; PMID:27048375; http://dx.doi.org/10.1186/s12864-016-2498-4
- van Eijk KR, de Jong S, Boks MPM, Langeveld T, Colas F, Veldink JH, de Kovel CGF, Janson E, Strengman E, Langfelder P, et al. Genetic analysis of DNA methylation and gene expression levels in whole blood of healthy human subjects. BMC Genomics 2012; 13:636; PMID:23157493; http://dx.doi.org/10.1186/1471-2164-13-636
- Zhang Y, Fukui N, Yahata M, Katsuragawa Y, Tashiro T, Ikegawa S, Michael Lee MT. Genome-wide DNA methylation profile implicates potential cartilage regeneration at the late stage of knee osteoarthritis. Osteoarthritis Cartilage 2016; 24:835-43; PMID:26746145; http://dx.doi.org/10.1016/j.joca.2015.12.013
- Jeffries MA, Donica M, Baker L, Stevenson M, Annan AC, Humphrey MB, James JA, Sawalha AH. Genome-wide DNA methylation study identifies significant epigenomic changes in osteoarthritic subchondral bone and similarity to overlying cartilage. Arthritis Rheumatol 2016; 68(6):1403-14 ; PMID:26713865; http://dx.doi.org/10.1002/art.39555
- Alm JJ, Koivu HMA, Heino TJ, Hentunen TA, Laitinen S, Aro HT. Circulating plastic adherent mesenchymal stem cells in aged hip fracture patients. J Orthop Res 2010; 28:1634-42; PMID:20540091; http://dx.doi.org/10.1002/jor.21167
- Qiang Y-W, Barlogie B, Rudikoff S, Shaughnessy JD. Dkk1-induced inhibition of Wnt signaling in osteoblast differentiation is an underlying mechanism of bone loss in multiple myeloma. Bone 2008; 42:669-80; PMID:18294945; http://dx.doi.org/10.1016/j.bone.2007.12.006
- Maupin KA, Droscha CJ, Williams BO. A Comprehensive Overview of Skeletal Phenotypes Associated with Alterations in Wnt/β-catenin Signaling in Humans and Mice. Bone Res 2013; 1:27-71; PMID:26273492; http://dx.doi.org/10.4248/BR201301004
- Baht GS, Silkstone D, Vi L, Nadesan P, Amani Y, Whetstone H, Wei Q, Alman BA. Exposure to a youthful circulaton rejuvenates bone repair through modulation of β-catenin. Nat Commun 2015; 6:7131; PMID:25988592; http://dx.doi.org/10.1038/ncomms8131
- Yang T-Y, Wang T-C, Tsai Y-H, Huang K-C. The effects of an injury to the brain on bone healing and callus formation in young adults with fractures of the femoral shaft. J Bone Joint Surg Br 2012; 94:227-30; PMID:22323691; http://dx.doi.org/10.1302/0301-620X.94B2.28193
- Lian JB, Gordon JA, Stein GS. Redefining the activity of a bone-specific transcription factor: novel insights for understanding bone formation. J Bone Miner Res 2013; 28:2060-3; PMID:23966343; http://dx.doi.org/10.1002/jbmr.2076
- Rojas A, Aguilar R, Henriquez B, Lian JB, Stein JL, Stein GS, van Wijnen AJ, van Zundert B, Allende ML, Montecino M. Epigenetic Control of the Bone-master Runx2 Gene during Osteoblast-lineage Commitment by the Histone Demethylase JARID1B/KDM5B. J Biol Chem 2015; 290:28329-42; PMID:26453309; http://dx.doi.org/10.1074/jbc.M115.657825
- Bruderer M, Richards RG, Alini M, Stoddart MJ. Role and regulation of RUNX2 in osteogenesis. Eur Cell Mater 2014; 28:269-86; PMID:25340806; http://dx.doi.org/10.22203/eCM.v028a19
- Antebi B, Pelled G, Gazit D. Stem cell therapy for osteoporosis. Curr Osteoporos Rep 2014; 12:41-7; PMID:24407712; http://dx.doi.org/10.1007/s11914-013-0184-x
- Kiernan J, Hu S, Grynpas MD, Davies JE, Stanford WL. Systemic Mesenchymal Stromal Cell Transplantation Prevents Functional Bone Loss in a Mouse Model of Age-Related Osteoporosis. Stem Cells Transl Med 2016; 5:683-93; PMID:26987353; http://dx.doi.org/10.5966/sctm.2015-0231
- Yao W, Lane NE. Targeted delivery of mesenchymal stem cells to the bone. Bone 2015; 70:62-5; PMID:25173607; http://dx.doi.org/10.1016/j.bone.2014.07.026
- Assenov Y, Müller F, Lutsik P, Walter J, Lengauer T, Bock C. Comprehensive analysis of DNA methylation data with RnBeads. Nat Methods 2014; 11:1138-40; PMID:25262207; http://dx.doi.org/10.1038/nmeth.3115
- Emig D, Salomonis N, Baumbach J, Lengauer T, Conklin BR, Albrecht M. AltAnalyze and DomainGraph: analyzing and visualizing exon expression data. Nucleic Acids Res 2010; 38:W755-62; PMID:20513647; http://dx.doi.org/10.1093/nar/gkq405
- Robinson MD, McCarthy DJ, Smyth GK. edgeR: a Bioconductor package for differential expression analysis of digital gene expression data. Bioinformatics 2010; 26:139-40; PMID:19910308; http://dx.doi.org/10.1093/bioinformatics/btp616
- McLean CY, Bristor D, Hiller M, Clarke SL, Schaar BT, Lowe CB, Wenger AM, Bejerano G. GREAT improves functional interpretation of cis-regulatory regions. Nat Biotechnol 2010; 28:495-501; PMID:20436461; http://dx.doi.org/10.1038/nbt.1630
- Wang J, Duncan D, Shi Z, Zhang B. WEB-based GEne SeT AnaLysis Toolkit (WebGestalt): update 2013. Nucleic Acids Res 2013; 41:W77-83; PMID:23703215; http://dx.doi.org/10.1093/nar/gkt439
- Xu J, Kelly R, Fang H, Tong W. ArrayTrack: a free FDA bioinformatics tool to support emerging biomedical research–an update. Hum Genomics 2010; 4:428-34; PMID:20846933
- Horvath S. DNA methylation age of human tissues and cell types. Genome Biol 2013; 14:R115; PMID:24138928; http://dx.doi.org/10.1186/gb-2013-14-10-r115
- Delgado-Calle J, Sañudo C, Sánchez-Verde L, García-Renedo RJ, Arozamena J, Riancho JA. Epigenetic regulation of alkaline phosphatase in human cells of the osteoblastic lineage. Bone 2011; 49:830-8; PMID:21700004; http://dx.doi.org/10.1016/j.bone.2011.06.006