ABSTRACT
There is growing evidence that histone lysine demethylases (KDMs) play critical roles in the regulation of embryo development. This study investigated if KDM7A, a lysine demethylase known to act on mono-(me1) and di-(me2) methylation of H3K9 and H3K27, participates in the regulation of early embryo development. Knockdown of KDM7A mRNA reduced blastocyst formation by 69.2% in in vitro fertilized (IVF), 48.4% in parthenogenetically activated (PA), and 48.1% in somatic cell nuclear transfer (SCNT) embryos compared to controls. Global immunofluorescence (IF) signal in KDM7A knockdown compared to control embryos was increased for H3K27me1 on D7, for H3K27me2 on D3 and D5, for H3K9me1 on D5 and D7, and for H3K9me2 on D5 embryos, but decreased for H3K9me1, me2 and me3 on D3. Moreover, KDM7A knockdown altered mRNA expression, including the downregulation of KDM3C on D3, NANOG on D5 and D7, and OCT4 on D7 embryos, and the upregulation of CDX2, KDM4B and KDM6B on D5 embryos. On D3 and D5 embryos, total cell number and mRNA expression of embryo genome activation (EGA) markers (EIF1AX and PPP1R15B) were not affected by KDM7A knockdown. However, the ratio of inner cell mass (ICM)/total number of cells in D7 blastocysts was reduced by 45.5% in KDM7A knockdown compared to control embryos. These findings support a critical role for KDM7A in the regulation of early development and cell lineage specification in porcine embryos, which is likely mediated through the modulation of H3K9me1/me2 and H3K27me1/me2 levels, and changes in the expression of other KDMs and pluripotency genes.
Introduction
Remodeling of the sperm and oocyte chromatin after fertilization involve several genetic and epigenetic events, which orchestrate the establishment of pluripotent cells required for the normal development of the embryo and fetal membranes [Citation1]. The parental genomes undergo extensive chromatin remodeling, including changes in global DNA methylation and post-translational histone modifications [Citation2], which not only alter chromatin structure [Citation3], but also modulate transcriptional activity [Citation4]. These events are dynamically regulated during early development and are essential for the EGA transition and cell differentiation during blastocyst formation [Citation5,Citation6].
Histone methylation is associated with either gene activation or repression, depending on which lysine (K) is modified and the level of methylation, i.e., mono- (me1), di- (me2), or tri- (me3) methylation. Generally, H3K4 methylation is found in promoter regions of actively transcribing genes [Citation7]. In contrast, high levels of H3K9 and H3K27 methylation correlate with transcriptional repression [Citation8], or heterochromatin formation and repression of gene transcription [Citation9], respectively. H3K27me3 and H3K27me2 have been associated with transcriptional inactivation of key developmental genes during stem cell differentiation [Citation7,Citation10], while H3K27me1 is found in intragenic regions and transcription start sites of actively transcribed genes in embryonic stem cells [Citation10,Citation11].
Methylation levels in the lysines 9 and 27 of the histone 3 during embryo development seems to differ between species. While H3K9me2 and H3K9me3 are restricted to maternally-derived chromatin in mouse embryos [Citation12], there is an asymmetric distribution of H3K9me2 in porcine zygotes, but it does not differentiate between male and female pronuclei [Citation13]. The global levels of H3K9me2/3 and H3K27me3 decrease from fertilization until the EGA transition, and then increase by the morula and blastocyst stages in bovine and porcine embryos [Citation14–Citation16]. This suggests that modulation of these repressive epigenetic marks is essential for EGA transition and cell differentiation in those species. However, the mechanisms by which the active and repressive methylation marks are regulated during early embryo development remain poorly understood.
Histone lysine methyltransferases (KMTs) and KDMs enzymes regulate gene expression by modulating histone methylation levels [Citation17]. Among all the histone modifications, the methylation levels of lysines 4, 9 and 27 in histone 3 are the most studied and have been shown to regulate embryo development and EGA transition [Citation18–Citation23], as well as cell differentiation and reprogramming [Citation24–Citation28]. Moreover, functional studies revealed that active removal of epigenetic marks is crucial for embryo development. For example, down-regulation of the KDM6B, which demethylates H3K27me3, compromised blastocyst formation and EGA transition in bovine embryos [Citation29]. Similarly, attenuation of KDM1A, a lysine demethylase that acts on H3K9me2, resulted in the arrest of mouse embryo development at the 2-cell stage [Citation21]. Moreover, depletion of KDM4C, a H3K9me3 demethylase, reduced embryo development and altered the expression of OCT4 and SOX2 genes, which are critical regulators of normal embryo development [Citation21,Citation23].
In a previous study, we found that several KDMs involved in the regulation of H3K4, H3K9 and H3K27 methylation levels were significantly more expressed during the EGA transition in bovine and porcine embryos [Citation30]. Among the KDMs presenting a bell-shape type of expression around the EGA transition was the KDM7A, which is known to have a dual demethylase activity on H3K9 and H3K27. KDM7A was previously shown to erase repressive marks on chromatin during brain development [Citation31,Citation32], and to regulate neural cell fate in mouse embryonic stem cells [Citation33], but no previous studies have investigated its importance for regulation of early embryo development. Therefore, our objective in this study was to evaluate the consequences of KDM7A dysregulation on porcine embryo development and quality, and to explore its potential mechanistic role for normal embryo development, EGA transition and cell lineage specification.
Results
KDM7A attenuation decreased embryo development
In the first experiment, the objective was to assess the importance of KDM7A for embryo development. Two DsiRNAs targeting KDM7A mRNA (si-KDM7A) or control (si-CT) were injected into 1-cell stage embryos produced by PA, IVF and SCNT. The relative knockdown efficiency in KDM7A mRNA levels was 72.1% on day 3, 79.2% on day 5, and 35% on day 7 PA-embryos that were injected with si-KDM7A compared to si-CT (Figure S1). Cleavage rates were similar between si-CT and si-KDM7A groups for PA (65.3 ± 3.2% vs. 58.3 ± 3.1%), IVF (49.0 ± 6.0% vs. 46.3 ± 1.4%) and SCNT (71.6 ± 3.3% vs. 65.3 ± 6.6%) embryos (). However, embryo development to the blastocyst stage was significantly reduced by 48.4% in PA (57.3 ± 3.6% vs. 29.7 ± 3.3%), 69.2% in IVF (30.3 ± 10.2% vs. 9.3 ± 6.3%), and 48.1% in SCNT (26.3 ± 3.4% vs. 13.6 ± 1.3%) embryos injected with si-KDM7A compared to si-CT (). Moreover, KDM7A knockdown hampered embryo quality, as evidenced by the significant decrease in the average number of cells in PA (52.2 ± 4.1 vs. 32.1 ± 4.4), IVF (38.3 ± 5 vs. 19.7 ± 2.3), and SCNT (29.7 ± 3.4 vs. 19.4 ± 6) embryos that developed to the blastocyst stage (). These results indicate that KDM7A is an important regulator of early development in porcine embryos. Given the similar effect of KDM7A knockdown on development of embryos produced by PA, IVF and SCNT, we opted for using only PA embryos in order to streamline subsequent studies aiming at investigating the role of KDM7A on embryo development.
Figure 1. Developmental rates and total number of cells in parthenogenetic (PA), in vitro fertilized (IVF) and somatic cell nuclear transfer (SCNT) embryos injected with si-CT (black bars) and si-KDM7A (white bars). Results are presented as means ± SEM, and P < 0.05 was considered statistically significant. Different letters indicate statistical significance between groups on the same day. Three independent replicates were performed using 30–40 embryos per group in each replicate.
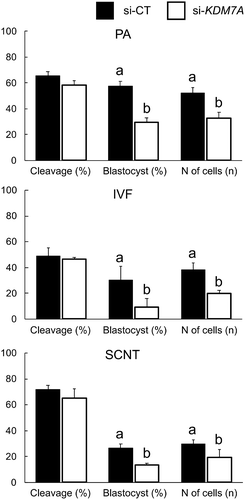
KDM7A attenuation altered H3K27 methylation levels
To evaluate the consequences of the KDM7A knockdown on its targets, the mono-(me1), di-(me2), and tri-(me3) methylation levels in the H3K27 were assessed by immunofluorescence on days 3, 5 and 7 of embryo development (). The relative fluorescence levels of H3K27me1 were increased on day 7 embryos injected with si-KDM7A compared to si-CT (-b). KDM7A knockdown also increased H3K27me2 fluorescence levels on day 3 and day 5 embryos compared to embryos injected with si-CT (-b). We could not quantify H3K27me3 levels because it was not possible to detect a clear immunofluorescence signal on the nuclei of embryos from all the developmental stages in both treatments ().
Figure 2. Immunofluorescence staining for H3K27me1, me2 and me3 on day 3, 5 and 7 PA-derived embryos injected with si-CT or si-KDM7A. (a) Representative pictures of H3K27me1, me2 and me3 staining in si-CT and si-KDM7A embryos. (b) Quantification of the pixel intensity for H3K27me1 and H3K27me2 in si-CT (black bars) and si-KDM7A (white bars). Results are presented as means ± SEM, and P < 0.05 was considered statistically significant. Different letters indicate statistical significance between groups on the same day. Values were corrected to 1 (dashed line) in si-CT groups and results are shown as relative differences between groups. Three independent replicates were performed and 10–15 embryos per group were used for quantification of pixel intensities.
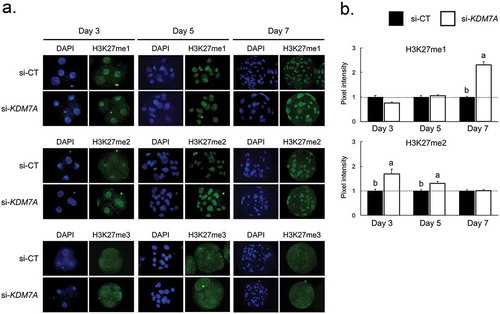
KDM7A attenuation altered H3K9 methylation levels
The mono-(me1), di-(me2), and tri-(me3) methylation levels in the H3K9 were evaluated by immunofluorescence on days 3, 5 and 7 of development in embryos injected with si-KDM7A or si-CT (). The fluorescence levels of H3K9me1, H3K9me2 and H3K9me3 were decreased on day 3 embryos injected with si-KDM7A compared to si-CT (-b). However, H3K9me1 and H3K9me2 fluorescence levels were increased on day 5 embryos and H3K9me1 on day 7 embryos injected with si-KDM7A compared to embryos injected with si-CT (-b). The fluorescence signal for H3K9me2 was weak and not quantifiable on nuclei of day 7 embryos from both si-KDM7A and si-CT groups.
Figure 3. mmunofluorescence staining for H3K9me1, me2 and me3 on day 3, 5 and 7 PA-derived embryos injected with si-CT or si-KDM7A. (a) Representative pictures of H3K9me1, me2 and me3 staining in si-CT and si-KDM7A embryos. (b) Quantification of the pixel intensity for H3K9me1, me2 and me3 in si-CT (black bars) and si-KDM7A (white bars). Results are presented as means ± SEM, and P < 0.05 was considered statistically significant. Different letters indicate statistical significance between groups on the same day. Values were corrected to 1 (dashed line) for si-CT groups and results are shown as relative differences between groups. Three independent replicates were performed and 10–15 embryos per group were used for quantification of pixel intensities.
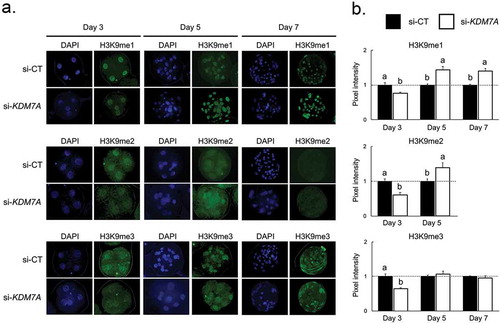
KDM7A attenuation altered mRNA expression levels of lysine demethylases (KDMs)
The relative mRNA abundance of genes encoding the main KDMs of H3K9, H3K27 and H3K4 were evaluated on days 3 and 5 of development in si-CT and si-KDM7A injected embryos. The relative mRNA abundance of two KDMs of H3K9 (KDM3C on day 3 and KDM4B on day 5) was increased in embryos injected with si-KDM7A compared to embryos injected with si-CT (), whereas the mRNA levels of other KDMs of H3K9 (KDM3A, KDM3B, KDM4C) were not significantly altered. The relative mRNA level of KDM6B, a H3K27 demethylase, was increased on day 5 embryos injected with si-KDM7A compared to si-CT, but the mRNA abundance of KDM6A, another H3K27 demethylase, was not altered by treatment in either days (). The mRNA levels of KDMs of H3K4 (KDM5B and KDM5C) were similar on day 3 and day 5 embryos injected with si-KDM7A or si-CT ().
Figure 4. Relative mRNA expression of H3K9 (a), H3K27 (b) and H3K4 (c) demethylases on day 3 and day 5 PA embryos injected with si-CT (black bars) or si-KDM7A (white bars). Results are presented as means ± SEM, and P < 0.05 was considered statistically significant. Different letters indicate statistical significance between groups on the same day. Three independent replicates were performed and pools of 10–15 embryos per group from each replicate were used for mRNA extraction.
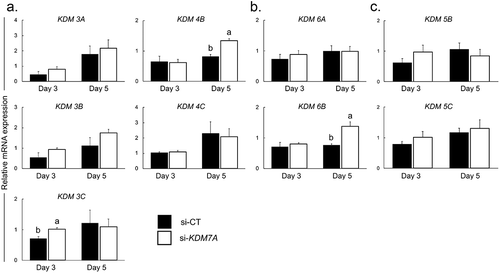
KDM7A attenuation disrupted embryo cell differentiation
Embryos produced by PA were used to determine the developmental stage that is affected by KDM7A knockdown. Analyses of embryos fixed on day 3, 5 and 7 of development revealed that KDM7A knockdown significantly decreased total cell number on day 7, but not on day 3 and day 5 embryos (). It was also observed that mRNA expression of EIF1AX, an important marker of embryo genome activation, was similar at day 3 and day 5 of development in embryos injected with si-KDM7A or si-CT (Figure S2). In addition, the relative mRNA abundance of PPP1R15B, which is an important marker of translation initiation [Citation34], was not altered by KDM7A attenuation on day 3 embryos (Figure S3). To evaluate if KDM7A knockdown affected embryo cell differentiation, the ratio of ICM/total number of cells was evaluated by Sox2/DAPI counterstaining (). The proportion of cells in the ICM (Sox2 stained nuclei) was reduced by 45.5% in embryos injected with si-KDM7A (8.4 ± 1.9%) compared to si-CT (15.5 ± 1.3%) ().
Figure 5. (a) Total number of cells on day 3, 5 and 7 PA embryos from si-CT (black bars) and si-KDM7A (white bars) groups. (b) Representative pictures of Sox2 immunofluorescence staining for differential cell count in blastocysts. (c) Ratio of ICM/total number of cells for si-CT (black bars) and si-KDM7A (white bars) blastocysts. Results are presented as means ± standard error of the mean (S.E.M), and P < 0.05 was considered statistically significant. Three independent replicates were performed and 10–15 embryos per group were used to evaluate total cell number and ratio of ICM/total number of cells.
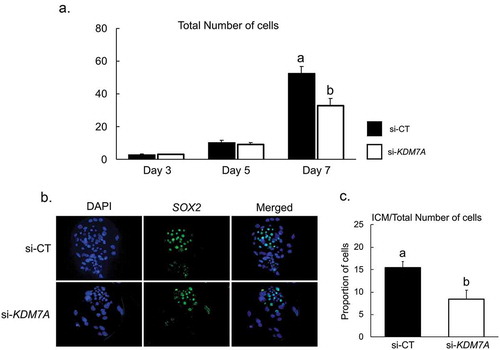
KDM7A attenuation altered expression of pluripotency genes
To determine the effects of KDM7A knockdown on mRNA expression of genes encoding for cell pluripotency and cell fate specification factors, the relative mRNA abundance of NANOG, OCT4, SOX2, LIN28 and CDX2 was quantified on day 5 and day 7 embryos, and SALL4, DPPA3, DPPA5, TBX3 and GATA6 on day 7 embryos. A significant decrease in the relative mRNA abundance of NANOG on day 5 and OCT4 on day 7 was detected in embryos injected with si-KDM7A compare to si-CT (). On the other hand, CDX2 mRNA abundance was increased on day 5 embryos from the si-KDM7A group compared to si-CT (). The relative mRNA expression of SOX2 and LIN28 on day 5 and 7 (), and SALL4, DPPA3, DPPA5, TBX3 and GATA6 on day 7 (Figure S4), was not affected by KDM7A knockdown.
Figure 6. Relative mRNA expression of genes involved on the regulation of cell pluripotency on day 5 and 7 PA embryos injected with si-CT (black bars) or si-KDM7A (white bars). Results are presented as means ± SEM, and P < 0.05 was considered statistically significant. Different letters indicate statistical significance between groups on the same day. * indicates p = 0.0592 value. Three independent replicates were performed and pools of 10–15 embryos per group from each replicate were used for mRNA extraction.
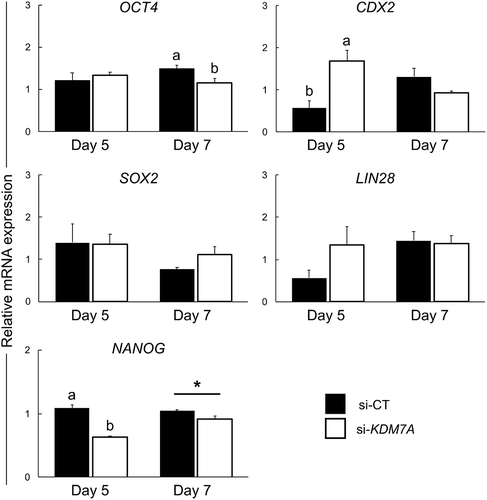
Discussion
Early embryo developmental programming after fertilization is controlled by epigenetic changes in the maternal and paternal genomes. In addition to the well-characterized global DNA demethylation [Citation35], several post-translational modifications in histones are dynamically regulated from fertilization to the blastocyst stage [Citation36]. However, much remains to be done regarding the elucidation of the functional roles controlled by each histone modification in early developing embryos. Several KMTs and KDMs controlling histone methylation levels in early developing embryos have been identified, but more functional studies are needed to fully characterize their roles on chromatin functions, cell differentiation and development. It has been shown some KMTs and KDMs modulate important embryo events including EGA transition [Citation5], and ICM and TE lineages specification at the blastocyst stage [Citation37]. In a previous study, we found that mRNA expression of various KDMs is dynamically regulated during early development of bovine and porcine embryos produced by fertilization and SCNT, suggesting their participation in the regulation of early development in those species [Citation30]. Interestingly, the mRNA expression of several KDMs, including the KDM7A, was transiently increased during the EGA transition in embryos of both species [Citation30]. Those findings suggested that KDM7A, a dual demethylase that acts on both mono- and di-methylation of lysines 9 and 27 of the histone 3, would be involved in the regulation of EGA transition, but the role of this KDM during early embryo development was not yet studied.
Findings from this study first revealed that KDM7A knockdown impaired both embryo development and quality, as determined by the lower blastocyst rates and the lower number of cells in embryos that developed to the blastocyst stage. This indicates that KDM7A participates in the regulation of critical events necessary for the normal development of porcine embryos. Our findings are aligned with those of previous studies that attenuated other KDMs also transiently regulated during the EGA transition, including KDM5B, KDM1A and KDM6B, which act on lysine 4 [Citation38], lysine 9 [Citation21], and lysine 27 [Citation29] of histone 3, respectively. Interesting, our results revealed that embryo development and quality were similarly affected by KDM7A knockdown in SCNT embryos compared to PA and IVF embryos. This suggests that KDM7A role is more likely in the regulation of the embryo developmental programing and may not be required for early cell reprogramming in SCNT embryos.
To confirm KDM7A attenuation efficiency, in addition to quantify it mRNA levels at different developmental stages, the me1, me2, me3 levels of H3K9 and H3K27 were evaluated in PA embryos on days 3, 5 and 7 of development. It was observed that KDM7A knockdown increased H3K27me2 levels on day 3 and day 5 embryos, and H3K27me1 levels on day 7 embryos. Increased levels of H3K9me1 on day 5 and 7 and H3K9me2 on day 5 were also detected in KDM7A knockdown embryos. These results confirmed that KDM7A can modulate both H3K9me1/me2 and H3K27me1/me2 levels in porcine embryos, as previously demonstrated in mouse embryonic stem cells by inhibiting its demethylase activity [Citation31]. A general demethylation of these repressive epigenetic marks was shown to take place after fertilization, which reached lowest levels during the EGA transition in embryos of different species including mice, cattle and swine [Citation16,Citation39–Citation41]. This dynamic regulation is thought to be necessary for the activation of pluripotency-related genes that are inactive during gametogenesis [Citation2,Citation22].
Unexpectedly, we observed that H3K9me1, H3K9me2 and H3K9me3 levels were decreased by KDM7A knockdown on day 3 embryos. Quantification of transcripts for the main KDMs of H3K27, H3K9 and H3K4 revealed that KDM3C mRNA expression, which acts on H3K9me1/2 [Citation42], was upregulated by KDM7A knockdown on day 3 embryos. Moreover, mRNA expression of KDM4C, which demethylates H3K9me2/3 [Citation43], was upregulated by KDM7A knockdown on day 5 embryos. The relative mRNA expression of KDM6B, which demethylates H3K27me3 [Citation29], was also upregulated on day 5 KDM7A knockdown embryos. However, the mRNA expression of KDMs of H3K4 (KDM5B and KDM5C) was not affected by KDM7A knockdown. These observations suggest that upregulation of other KDMs acting on the same repressive marks may promote a compensatory effect in response to KDM7A dysregulation. However, further studies are needed to explain the interplay among KDMs acting on the same histone modification.
To explore the mechanism by which KDM7A affected porcine embryo development, cell lineage specification and expression of pluripotency regulators were compared in si-CT and si-KDM7A embryos. Total cell number was similar on days 3 and 5 of development in embryos from si-KDM7A and si-CT treatments. This suggests that KDM7A is not essential for regulation of events linked to the EGA transition. Likewise, the mRNA expression of EIF1AX, an important marker of EGA transition in porcine embryos [Citation44], was not altered by KDM7A knockdown on day 3 and day 5 embryos. On the other hand, total cell number on day 7 blastocysts and ICM/total cell ratio were significantly decreased by KDM7A knockdown. This indicates that KDM7A is involved in the first cell lineages specification during morula to blastocyst transition, which is critical for subsequent phases of development. In mice, histone post-translational modifications seem to be the main epigenetic component regulating transcription during trophectoderm (TE) and ICM specification. Indeed, there is evidence that the methylation levels of H3K9me3 and H3K27me3 affect the regulation of extraembryonic cell lineages formation [Citation37], a process that was shown to be independent of DNA methylation [Citation45]. In this context, the H3K9me3 methyltransferase SETDB1 was shown to repress the expression of genes from the TE-specific lineage [Citation46,Citation47]. Moreover, H3K9me3 is also important for repressing ICM-specific genes in the TE lineage [Citation48]. Different levels of H3K27me3 were also observed between ICM and TE cells in pig blastocysts [Citation49,Citation50], which suggests a role in the first cell lineages specification. Findings from this study provide evidence that KDM7A participates in this process by regulating methylation levels on both H3K9 and H3K27.
In order to evaluate if KDM7A is involved in the regulation of transcription factors controlling cell lineage specification, the relative mRNA abundance of genes involved in the process [Citation37] was compared between si-KDM7A and si-CT embryos on days 5 and 7 of development. In mice, transcription factors such as NANOG, OCT4 and SOX2 are strictly expressed in the ICM while CDX2 is only expressed by TE cells [Citation51,Citation52]. In pig embryos, CDX2 is detected only in the TE cells, but OCT4 can be detected in both ICM and TE cells at the blastocyst stage [Citation53], while SOX2 [Citation54] and NANOG [Citation55] are only expressed by ICM cells. It has been shown that OCT4 expression is required for NANOG activation in bovine embryos [Citation56], and overexpression of NANOG promoted the activation of pluripotency genes in porcine fibroblasts [Citation57]. We observed that KDM7A knockdown increased the CDX2 mRNA abundance on day 5 embryos, while NANOG and OCT4 mRNA levels were decreased on day 5 and day 7 embryos, respectively. This suggests that the altered cell phenotype, i.e., decreased ICM/total number of cells ratio, observed in KDM7A knockdown embryos may be a consequence of the abnormal regulation of those genes. Whether KDM7A activity directly regulates the expression of NANOG, OCT4 and CDX2 by modulating H3K9 and/or H3K27 methylation patterns in their regulatory sequences or indirectly by modulating other transcriptional regulators of those genes remains to be determined.
In conclusion, findings from this study revealed that KDM7A participates in the regulation of normal development of porcine embryos. Attenuation of KDM7A mRNA altered H3K9 and H3K27 methylation levels, decreased embryo development and total cell number at the blastocyst stage and altered ICM/total number of cells ratio in blastocysts. This study also provides solid evidence that KDM7A regulates events controlling first cell lineage specification after the EGA transition, by directly or indirectly regulating the expression of genes controlling cell pluripotency.
Material and methods
Oocyte collection and in vitro maturation (IVM)
Ovaries of prepubertal gilts were collected at a local slaughterhouse (Olymel S.E.C./L.P., Saint-Esprit, QC, Canada) and transported to the laboratory at 32°C in saline solution containing penicillin (100 UI/ml – Sigma P4333; Sigma-Aldrich, Oakville, ON, Canada) and streptomycin (10 mg/ml – Sigma P4333). Cumulus-oocyte complexes (COCs) were aspirated from 3 to 6 mm follicles using a 10 mL syringe and 20-gauge needle and only those having a minimum of three cumulus cells layers and a homogeneously granulated cytoplasm were selected for IVM.
IVM was conducted in 90 μl drops of medium under mineral oil in 5% CO2 incubator at 38.5°C and high humidity. For the first 22 h of the maturation medium consisted of TCM 199 (Life technologies – 12,340–030), supplemented with 20% of porcine follicular fluid, 1mM dibutyryl cyclic adenosine monophosphate (dbcAMP – Sigma D0260), 0.1 mg/mL cysteine (Sigma C6852), 10 ng/mL epidermal growth factor (EGF; Life technologies PHG0314), 0.91 mM sodium pyruvate (Sigma P4567), 3.05 mM D-glucose (Sigma G6152), 0.5 µg/mL LH (Sioux Biochemical Inc. 70,025), 0.5 µg/mL FSH (Sioux Biochemical Inc. 70,015), and 20 µg/mL gentamicin (Sigma G1272). To complete IVM, COCs were transferred to the same IVM medium, but without LH, FSH and dbcAMP, for additional 20 to 22 h under the same conditions.
In vitro fertilization (IVF)
After IVM, cumulus cells were removed by vortexing in TCM 199 HEPES-buffered medium (Life Technologies, 12,340–030) supplemented with 0.1% hyaluronidase (Sigma H4272). Denuded oocytes were washed three times in pre-stabilized modified Tris-Buffered Medium (mTBM) [Citation58], containing 2 mM caffeine (Sigma C0750) and 0.1% BSA (Sigma A6003), and then co-culture in groups of 70–80 oocytes/well with 2 × 105 sperm/ml, in four-well plates with 500 µl medium for 5 h under the same atmospheric conditions used for IVM.
Somatic cell nuclear transfer (SCNT) and parthenogenetic activation (PA)
Porcine fibroblast cells were cultured in vitro in Dulbecco’s Modified Eagle Medium/Nutrient Mixture F-12 Ham (DMEM-F12 – Sigma D8900), supplemented with 10% fetal bovine serum (FBS; Life Technologies 12,484–028) and 1% antibiotics (10,000 U/mL penicillin and 10,000 µg/mL streptomycin) at 38°C in 5% CO2 and 95% air. For SCNT, matured oocytes with an extruded polar body were cultured in TCM 199 supplemented with 0.4 µg/mL demecolcine (Sigma D1925) and 0.05 M sucrose (Sigma S1888) for 60 min. This treatment resulted in a small protrusion in the ooplasmic membrane that contained the metaphase chromosomes. Oocytes were transferred to TCM-199 HEPES-buffered medium supplemented with 2 mg/mL bovine serum albumin (BSA, fatty acid free – Sigma A6003), 20 µg/mL gentamicin, and 7.5 µg/mL cytochalasin B (Sigma C6762) for 5–10 min, and then enucleated by removing the protruded chromatin and the first polar body. A nuclear donor cell was transferred into the perivitelline space of each enucleated oocyte, and then electrofused using a single DC pulse of 32V for 70 µsec. Electrofusion was performed in a 0.28 M mannitol (Sigma M9647) solution supplemented with 50 µM CaCl2 (Sigma C7902), 100 µM MgSO4 (Sigma M7774), and 0.1% BSA. Oocytes were then transferred to TCM-199 medium supplemented with 3 mg/mL BSA for 1 h to allow cell fusion.
For parthenogenetic activation, matured porcine oocytes were exposed to 15 µM ionomycin (Sigma I0634) for 5 min followed by 4 h culture in Ca2+ free porcine zygote medium (PZM-3) [Citation59] supplemented with 10 mM strontium chloride (Sigma 439,665), 7.5 µg/mL of cytochalasin B and 10 µg/mL of cycloheximide (Sigma C7698) [Citation60]. SCNT oocytes were activated immediately after cell fusion using the same activation protocol.
Embryo culture
Embryos produced by PA, IVF or SCNT were cultured in PZM-3 medium in a humidified atmosphere of 5% CO2 and 95% air at 38.5°C. At day 5 of development, the medium was supplemented with 10% FBS. Cleavage rates were evaluated at 48 h and blastocyst rates at day 7 of embryo development.
KDM7A knockdown
For knockdown experiments, Dicer-substrate short interfering RNAs (DsiRNAs) were designed (Custom DsiRNA Design Tool) and synthetized by Integrated DNA Technologies (Windsor, ON, CA). Specificity was confirmed by using the Basic Local Alignment Search Tool (BLAST; National Center for Biotechnology Information, Bethesda, MD, USA). Oocytes were microinjected using a FemtoJet 4i system (Eppendorf Canada, Mississauga, Ontario), with 10 pl of 25 μM diluted sense and antisense DsiRNAs that targeted 2 different sequences in the KDM7A mRNA, or control nonsense scrambled sequences (Table S1). Microinjection was performed in TCM199 HEPES-buffered medium supplemented with 2 mg/ml BSA (fatty acid free) and 20 µg/ml gentamicin, using an inverted Nikon microscope (Nikon, Tokyo, Japan) equipped with a micromanipulator system (Narishige International, Long Island, NY, USA). The knockdown efficiency was assessed by quantifying the relative mRNA abundance of KDM7A by quantitative PCR on days 3, 5 and 7 after DsiRNAs microinjection. DsiRNAs were microinjected before activation, after fertilization and after activation for PA, IVF and SCNT embryos, respectively.
RNA extraction and reverse transcription quantitative PCR (RT-qPCR)
Total RNA was extracted from pools of 10–15 embryos on days 3, 5 and 7 of development using the PicoPure RNA Isolation Kit (Life Technologies KIT0204) according to the manufacturer’s instructions. RNA was treated with DNase I (Qiagen 79,254) and reverse transcribed using the SuperScript VILO cDNA Synthesis Kit (Life Technologies 11,754–050). RT-qPCR reactions were performed in a CFX 384 real-time PCR system (BioRad, Hercules, CA, USA) using the advanced qPCR Mastermix (Wisent Bioproducts 800–431). Primers were designed based on porcine (Table S2) sequences available in GenBank and synthesized by IDT. Samples were run in duplicates and the standard curve method was used to determine the abundance of mRNA for each gene. Relative mRNA expression was normalized to the mean abundance of the internal control gene H2A. All reactions had efficiency between 90 and 110%, r2 ≥ 0.98 and slope values from −3.6 to −3.1. Dissociation curve analyses were performed to validate the specificity of the amplification products.
Immunofluorescence staining
Embryos from the different experimental groups were collected on days 3, 5 and 7 of development, rinsed in PBS containing 0.1% polyvinyl alcohol (PVA; Sigma P8136) and fixed during 15–20 min in 4% paraformaldehyde. Fixed embryos were rinsed in PBS containing 0.1% PVA and stored at 4ºC in PBS supplemented with 0.3% BSA and 0.1% Triton X-100 (Sigma T8787) for no more than 1 week. For immunofluorescence staining, fixed embryos were incubated for 2 h at room temperature in blocking solution (3% BSA and 0.2% Tween-20 (Sigma P1379) in PBS), and then maintained overnight in the presence of one of the following primary antibodies: anti-histone H3K27 mono-methyl (Upstate; 07–448 diluted 1:1000), anti-histone H3K27 di-methyl (Upstate; 07–452 diluted 1:1000), anti-histone H3K27 tri-methyl (Upstate; 07–449 diluted 1:1000), anti-histone H3K9 mono-methyl (ab-176,880, diluted 1:1000), anti-histone H3K9 di-methyl (ab-176,882, diluted 1:1500), anti-histone H3K9 tri-methyl (ab-176,916, diluted 1:1000), or Sox-2 antibody (sc-365,823, diluted 1:200). Samples were then washed three times for 20 minutes each in blocking solution and incubated in the presence of 1:2000 diluted Alexa Fluor 488 (Abcam, ab-150,077) anti-rabbit secondary antibody. Samples were washed three times (20 min each) in blocking solution, and the last wash was supplemented with 10 µg/ml 4,6-diamidino-2-phenylindole (DAPI – Invitrogen D1306) for DNA staining, and then mounted on a slide using a drop of mowiol (Sigma 32,449–0). Slides were stored in a dark box at 4ºC and examined within 48 h after preparation. The slides were examined by epifluorescence using a Nikon eclipse 80i microscope (Nikon, Tokyo, Japan) with ×200 magnification. Images were individually recorded using a Retiga 2000R monochrome digital camera (Qimaging, BC, Canada). The exposure gains and rates were consistent between samples. Fluorescence intensities were quantified using the ImageJ software. Control samples from each developmental stage were processed as described above but the primary antibody was omitted. Differential cell count on embryos that developed to the blastocyst stage on day 7 of culture was performed by dividing the number of Sox-2 positive nuclei by the total number of nuclei.
Statistical analysis
Data of cleavage, embryo development, number of cells, pixel intensity quantification and gene expression were analyzed by analysis of variance (ANOVA) followed by the LSMeans Student t-test using the JMP software (SAS Institute Inc., Cary, NC). Data were tested for normal distribution using the Shapiro–Wilk test and normalized when necessary. Results are presented as means ± standard error of the mean (SEM), and p < 0.05 was considered statistically significant.
Supplemental Material
Download MS Word (7.9 MB)Supplemental Material
Download MS Word (23.3 KB)Acknowledgments
The authors are thankful to Olymel S.E.C./L.P. abattoirs for donation of porcine ovaries, and CIPQ Inc. for the donation of porcine semen.
Disclosure statement
No potential conflict of interest was reported by the authors.
Supplementary Material
The supplementary material for this article can be accessed here.
Additional information
Funding
References
- Rivera RM, Ross JW. Epigenetics in fertilization and preimplantation embryo development. Prog Biophys Mol Biol. 2013 Dec;113(3):423–432. PubMed PMID: 23454467.
- Marcho C, Cui W, Mager J. Epigenetic dynamics during preimplantation development. Reproduction. 2015 Sep;150(3):R109–20. PubMed PMID: 26031750; PubMed Central PMCID: PMCPMC4529766.
- Cabot B, Cabot RA. Chromatin remodeling in mammalian embryos. Reproduction. 2018 Mar;155(3):R147–R158. PubMed PMID: 29339454.
- Li L, Lu X, Dean J. The maternal to zygotic transition in mammals. Mol Aspects Med. 2013 Oct;34(5):919–938. PubMed PMID: 23352575; PubMed Central PMCID: PMCPMC3669654.
- Eckersley-Maslin MA, Alda-Catalinas C, Reik W. Dynamics of the epigenetic landscape during the maternal-to-zygotic transition. Nat Rev Mol Cell Biol. 2018 Apr 23. PubMed PMID: 29686419. DOI:10.1038/s41580-018-0008-z.
- Ostrup O, Andersen IS, Collas P. Chromatin-linked determinants of zygotic genome activation. Cell Mol Life Sci. 2013 Apr;70(8):1425–1437. PubMed PMID: 22965566.
- Bernstein BE, Mikkelsen TS, Xie X, et al. A bivalent chromatin structure marks key developmental genes in embryonic stem cells. Cell. 2006 Apr 21;125(2):315–326. PubMed PMID: 16630819.
- Boyer LA, Plath K, Zeitlinger J, et al. Polycomb complexes repress developmental regulators in murine embryonic stem cells. Nature. 2006 May 18;441(7091):349–353. PubMed PMID: 16625203.
- Bannister AJ, Zegerman P, Partridge JF, et al. Selective recognition of methylated lysine 9 on histone H3 by the HP1 chromo domain. Nature. 2001 Mar 1;410(6824):120–124. PubMed PMID: 11242054.
- Barski A, Cuddapah S, Cui K, et al. High-resolution profiling of histone methylations in the human genome. Cell. 2007 May 18;129(4):823–837. PubMed PMID: 17512414.
- Ferrari KJ, Scelfo A, Jammula S, et al. Polycomb-dependent H3K27me1 and H3K27me2 regulate active transcription and enhancer fidelity. Mol Cell. 2014 Jan 9;53(1):49–62. PubMed PMID: 24289921.
- Lepikhov K, Walter J. Differential dynamics of histone H3 methylation at positions K4 and K9 in the mouse zygote. BMC Dev Biol. 2004;4:1.
- Sega MF, Lee K, Machaty Z, et al. Pronuclear stage porcine embryos do not possess a strict asymmetric distribution of lysine 9 dimethylation of histone H3 based solely on parental origin. Mol Reprod Dev. 2007;74(1):2–7.
- Cao Z, Li Y, Chen Z, et al. Genome-wide dynamic profiling of histone methylation during nuclear transfer-mediated porcine somatic cell reprogramming. PLoS One. 2015;10(12):e0144897. PubMed PMID: 26683029; PubMed Central PMCID: PMCPMC4687693.
- Gao Y, Hyttel P, Hall VJ. Regulation of H3K27me3 and H3K4me3 during early porcine embryonic development. Mol Reprod Dev. 2010 Jun;77(6):540–549. PubMed PMID: 20422712.
- Ross PJ, Ragina NP, Rodriguez RM, et al. Polycomb gene expression and histone H3 lysine 27 trimethylation changes during bovine preimplantation development. Reproduction. 2008 Dec;136(6):777–785. PubMed PMID: 18784248.
- Hyun K, Jeon J, Park K, et al. Writing, erasing and reading histone lysine methylations. Exp Mol Med. 2017 Apr 28;49(4):e324. PubMed PMID: 28450737.
- Glanzner WG, Wachter A, Coutinho AR, et al. Altered expression of BRG1 and histone demethylases, and aberrant H3K4 methylation in less developmentally competent embryos at the time of embryonic genome activation. Mol Reprod Dev. 2017 Jan;84(1):19–29. PubMed PMID: 27879032.
- Liu X, Wang C, Liu W, et al. Distinct features of H3K4me3 and H3K27me3 chromatin domains in pre-implantation embryos. Nature. 2016 Sep 22;537(7621):558–562. PubMed PMID: 27626379.
- Dahl JA, Jung I, Aanes H, et al. Broad histone H3K4me3 domains in mouse oocytes modulate maternal-to-zygotic transition. Nature. 2016 Sep 22;537(7621):548–552. PubMed PMID: 27626377.
- Ancelin K, Syx L, Borensztein M, et al. Maternal LSD1/KDM1A is an essential regulator of chromatin and transcription landscapes during zygotic genome activation. Elife. 2016 Feb 2:5. PubMed PMID: 26836306; PubMed Central PMCID: PMCPMC4829419. DOI:10.7554/eLife.08851.
- Bogliotti YS, Ross PJ. Mechanisms of histone H3 lysine 27 trimethylation remodeling during early mammalian development. Epigenetics. 2012 Sep;7(9):976–981. PubMed PMID: 22895114; PubMed Central PMCID: PMCPMC3515017.
- Wang J, Zhang M, Zhang Y, et al. The histone demethylase JMJD2C is stage-specifically expressed in preimplantation mouse embryos and is required for embryonic development. Biol Reprod. 2010 Jan;82(1):105–111. PubMed PMID: 19696013.
- Zhang Z, Zhai Y, Ma X, et al. Down-regulation of H3K4me3 by MM-102 facilitates epigenetic reprogramming of porcine somatic cell nuclear transfer embryos. Cell Physiol Biochem. 2018;45(4):1529–1540. PubMed PMID: 29466785.
- Liu X, Wang Y, Gao Y, et al. H3K9 demethylase KDM4E is an epigenetic regulator for bovine embryonic development and a defective factor for nuclear reprogramming. Development. 2018 Feb 16;145(4):1-12. 10.1242/dev.158261. PubMed PMID: 29453221.
- Hormanseder E, Simeone A, Allen GE, et al. H3K4 methylation-dependent memory of somatic cell identity inhibits reprogramming and development of nuclear transfer embryos. Cell Stem Cell. 2017 Jul 6;21(1):135–143 e6. PubMed PMID: 28366589; PubMed Central PMCID: PMCPMC5505866.
- Xie B, Zhang H, Wei R, et al. Histone H3 lysine 27 trimethylation acts as an epigenetic barrier in porcine nuclear reprogramming. Reproduction. 2016 Jan;151(1):9–16. PubMed PMID: 26515777.
- Matoba S, Liu Y, Lu F, et al. Embryonic development following somatic cell nuclear transfer impeded by persisting histone methylation. Cell. 2014 Nov 6;159(4):884–895. PubMed PMID: 25417163; PubMed Central PMCID: PMCPMC4243038.
- Chung N, Bogliotti YS, Ding W, et al. Active H3K27me3 demethylation by KDM6B is required for normal development of bovine preimplantation embryos. Epigenetics. 2017;12(12):1048–1056. PubMed PMID: 29160132; PubMed Central PMCID: PMCPMC5810760.
- Glanzner WG, Rissi VB, de Macedo MP, et al. Histone 3 lysine 4, 9 and 27 demethylases expression profile in fertilized and cloned bovine and porcine embryos. Biol Reprod. 2018 Mar 8. PubMed PMID: 29528362. DOI:10.1093/biolre/ioy054.
- Tsukada Y, Ishitani T, Nakayama KI. KDM7 is a dual demethylase for histone H3 Lys 9 and Lys 27 and functions in brain development. Genes Dev. 2010 Mar 1;24(5):432–437. PubMed PMID: 20194436; PubMed Central PMCID: PMCPMC2827838.
- Huang C, Chen J, Zhang T, et al. The dual histone demethylase KDM7A promotes neural induction in early chick embryos. Dev Dyn. 2010;239(12):3350–3357.
- Huang C, Xiang Y, Wang Y, et al. Dual-specificity histone demethylase KIAA1718 (KDM7A) regulates neural differentiation through FGF4. Cell Res. 2010 Feb;20(2):154–165. PubMed PMID: 20084082.
- Huang Y, Kim JK, Do DV, et al. Stella modulates transcriptional and endogenous retrovirus programs during maternal-to-zygotic transition. Elife. 2017 Mar 21;6. PubMed PMID: 28323615; PubMed Central PMCID: PMCPMC5404928. DOI:10.7554/eLife.22345.
- Dean W, Santos F, Stojkovic M, et al. Conservation of methylation reprogramming in mammalian development: aberrant reprogramming in cloned embryos. Proc Natl Acad Sci U S A. 2001 Nov 20;98(24):13734–13738. PubMed PMID: 11717434; PubMed Central PMCID: PMCPMC61110.
- Morgan HD, Santos F, Green K, et al. Epigenetic reprogramming in mammals. Hum Mol Genet. 2005 Apr 15;14(1):R47–58. PubMed PMID: 15809273.
- Paul S, Knott JG. Epigenetic control of cell fate in mouse blastocysts: the role of covalent histone modifications and chromatin remodeling. Mol Reprod Dev. 2014 Feb;81(2):171–182. PubMed PMID: 23893501; PubMed Central PMCID: PMCPMC4276566.
- Huang J, Zhang H, Wang X, et al. Impairment of preimplantation porcine embryo development by histone demethylase KDM5B knockdown through disturbance of bivalent H3K4me3-H3K27me3 modifications. Biol Reprod. 2015 Mar;92(3):72. PubMed PMID: 25609834; PubMed Central PMCID: PMCPMC4367965.
- van der Heijden GW, Dieker JW, Derijck AA, et al. Asymmetry in histone H3 variants and lysine methylation between paternal and maternal chromatin of the early mouse zygote. Mech Dev. 2005 Sep;122(9):1008–1022. PubMed PMID: 15922569.
- Liu H, Kim JM, Aoki F. Regulation of histone H3 lysine 9 methylation in oocytes and early pre-implantation embryos. Development. 2004 May;131(10):2269–2280. PubMed PMID: 15102709.
- Erhardt S, Su IH, Schneider R, et al. Consequences of the depletion of zygotic and embryonic enhancer of zeste 2 during preimplantation mouse development. Development. 2003 Sep;130(18):4235–4248. PubMed PMID: 12900441.
- Shen H, Xu W, Lan F. Histone lysine demethylases in mammalian embryonic development. Exp Mol Med. 2017 Apr 28;49(4):e325. PubMed PMID: 28450736.
- Loh YH, Zhang W, Chen X, et al. Jmjd1a and Jmjd2c histone H3 Lys 9 demethylases regulate self-renewal in embryonic stem cells. Genes Dev. 2007 Oct 15;21(20):2545–2557. PubMed PMID: 17938240; PubMed Central PMCID: PMCPMC2000320.
- Magnani L, Johnson CM, Cabot RA. Expression of eukaryotic elongation initiation factor 1A differentially marks zygotic genome activation in biparental and parthenogenetic porcine embryos and correlates with in vitro developmental potential. Reprod Fertil Dev. 2008;20(7):818–825. PubMed PMID: 18842184.
- Sakaue M, Ohta H, Kumaki Y, et al. DNA methylation is dispensable for the growth and survival of the extraembryonic lineages. Curr Biol. 2010 Aug 24;20(16):1452–1457. PubMed PMID: 20637626.
- Yuan P, Han J, Guo G, et al. Eset partners with Oct4 to restrict extraembryonic trophoblast lineage potential in embryonic stem cells. Genes Dev. 2009 Nov 1;23(21):2507–2520. PubMed PMID: 19884257; PubMed Central PMCID: PMCPMC2779752.
- Yeap LS, Hayashi K, Surani MA. ERG-associated protein with SET domain (ESET)-Oct4 interaction regulates pluripotency and represses the trophectoderm lineage. Epigenetics Chromatin. 2009 Oct 7;2(1):12. PubMed PMID: 19811652; PubMed Central PMCID: PMCPMC2763847.
- Alder O, Lavial F, Helness A, et al. Ring1B and Suv39h1 delineate distinct chromatin states at bivalent genes during early mouse lineage commitment. Development. 2010 Aug 1;137(15):2483–2492. PubMed PMID: 20573702; PubMed Central PMCID: PMCPMC2927698.
- Marinho LSR, Rissi VB, Lindquist AG, et al. Acetylation and methylation profiles of H3K27 in porcine embryos cultured in vitro. Zygote. 2017;25(05):575–582.
- Park KE, Magnani L, Cabot RA. Differential remodeling of mono- and trimethylated H3K27 during porcine embryo development. Mol Reprod Dev. 2009 Nov;76(11):1033–1042. PubMed PMID: 19536841.
- Strumpf D, Mao CA, Yamanaka Y, et al. Cdx2 is required for correct cell fate specification and differentiation of trophectoderm in the mouse blastocyst. Development. 2005 May;132(9):2093–2102. PubMed PMID: 15788452.
- Mitsui K, Tokuzawa Y, Itoh H, et al. The homeoprotein Nanog is required for maintenance of pluripotency in mouse epiblast and ES cells. Cell. 2003 May 30;113(5):631–642. PubMed PMID: 12787504.
- Kuijk EW, Du Puy L, Van Tol HT, et al. Differences in early lineage segregation between mammals. Dev Dyn. 2008 Apr;237(4):918–927. PubMed PMID: 18330925.
- Liu S, Bou G, Sun R, et al. Sox2 is the faithful marker for pluripotency in pig: evidence from embryonic studies. Dev Dyn. 2015 Apr;244(4):619–627. PubMed PMID: 25619399.
- Hall VJ, Christensen J, Gao Y, et al. Porcine pluripotency cell signaling develops from the inner cell mass to the epiblast during early development. Dev Dyn. 2009 Aug;238(8):2014–2024. PubMed PMID: 19618464.
- Simmet K, Zakhartchenko V, Philippou-Massier J, et al. OCT4/POU5F1 is required for NANOG expression in bovine blastocysts. Proc Natl Acad Sci U S A. 2018 Mar 13;115(11):2770–2775. PubMed PMID: 29483258; PubMed Central PMCID: PMCPMC5856541.
- Zhang L, Luo Y-B, Bou G, et al. Overexpression nanog activates pluripotent genes in porcine fetal fibroblasts and nuclear transfer embryos. Anat Rec. 2011;294(11):1809–1817.
- Abeydeera LR, Day BN. Fertilization and subsequent development in vitro of pig oocytes inseminated in a modified tris-buffered medium with frozen-thawed ejaculated spermatozoa. Biol Reprod. 1997 Oct;57(4):729–734. PubMed PMID: 9314573.
- Yoshioka K, Suzuki C, Tanaka A, et al. Birth of piglets derived from porcine zygotes cultured in a chemically defined medium. Biol Reprod. 2002 Jan;66(1):112–119. PubMed PMID: 11751272.
- Che L, Lalonde A, Bordignon V. Chemical activation of parthenogenetic and nuclear transfer porcine oocytes using ionomycin and strontium chloride. Theriogenology. 2007 Apr 15;67(7):1297–1304. PubMed PMID: 17350088.