ABSTRACT
Epigenetic modifications, such as DNA methylation, can be regulated by nutrition and dietary factors. There has been a large increase in the use of sustainable plant-based protein sources in fish feed due to limitations of fishmeal resources, which are needed to sustain a rapidly growing aquaculture industry. With this major transition from marine ingredients to plant-based diets, fish are abruptly introduced to changes in dietary composition and exposed to a variety of phytochemicals, some of which known to cause epigenetic changes in mammals. However, the effect of plant ingredients on the epigenome of fish is barely understood. In the present study, the nutriepigenomic effects of the addition of pea, soy, and wheat gluten protein concentrate to aquafeeds were investigated using zebrafish as a model. A genome-wide analysis of DNA methylation patterns was performed by reduced representation bisulphite sequencing to examine global epigenetic alterations in the mid intestine after a 42-day feeding trial. We found that inclusion of 30% of wheat gluten, pea and soy protein concentrate in the diet induced epigenetic changes in the mid intestine of zebrafish. A large number of genes and intergenic regions were differentially methylated with plant-based diets. The genes concerned were related to immunity, NF‐κB system, ubiquitin-proteasome pathway, MAPK pathway, and the antioxidant defence system. Epigenetic regulation of several biological processes, including neurogenesis, cell adhesion, response to stress and immunity was also observed. Ultimately, the observed epigenetic changes may enable zebrafish to rapidly regulate inflammation and maintain intestinal homoeostasis when fed plant protein–based diets.
Introduction
Epigenetic modifications, such as DNA methylation, can modulate gene expression without changing the actual genome sequence [Citation1]. Nutritional and dietary factors are believed to affect DNA methylation by altering the substrates and cofactors necessary for DNA methylation, and by changing the activity of the enzymes regulating the one-carbon cycle or DNA demethylation activity [Citation2,Citation3]. In recent years, there has been a marked increase in the use of plant ingredients in aquafeeds, exposing fish to dietary changes and to a variety of phytochemicals and phytoestrogens, which are known to cause epigenetic changes in mammals. In spite of its potential impact on fish health and growth, the effect of plant ingredients on the epigenome of fish is barely understood.
Limitations in fishmeal resources to supply the feed requirements of the rapidly growing aquaculture industry have steered the exploration of novel sustainable plant-based protein sources for fish feed [Citation4,Citation5]. As a result, the dependency of the aquaculture industry on fishmeal is gradually decreasing; during the period from 1990 to 2013 the Norwegian salmon industry reported a large decrease in the use of marine resources from 90% to 30% [Citation6], and this is expected to further decrease [Citation7]. With this complete dietary transition from marine ingredients to plant-based diets, fish are introduced to changes in nutritional content and exposed to a variety of phytochemicals. Essential constituents in plant feed ingredients, such as structural components, storages of nutrients and energy, allergens and plant defence metabolites can act as antinutrients (ANF) and interfere with nutrient digestibility, absorption, and utilization, and may adversely affect animal health and performance [Citation8,Citation9]. Phytoestrogens are plant analogues of animal oestrogens and they can potentially affect all processes regulated through oestrogenic receptors, as well as mediate genomic and epigenomic processes in mammals [Citation10,Citation11].
High protein concentrates produced from soy, wheat, or other grains and oilseeds are the most promising and widely used alternative protein sources in aquafeeds [Citation4]. Soy protein concentrate (SPC) is among the most utilized single alternative protein sources due to its high protein content, comparably favourable amino acid profile and low fibre content [Citation4,Citation12]. Furthermore, SPC does not contain the ANF saponin and is low in trypsin inhibitors, storage globulins with antigenic properties and oligosaccharides in comparison to soybean meal (SBM) [Citation12,Citation13]. Some studies indicated that SPC does not cause intestinal enteritis similar to that induced by SBM in salmonids [Citation14,Citation15], but that it is able to cause transcriptomic changes in the intestine of Atlantic salmon at a 45% inclusion level [Citation14]. Several in vitro and in vivo mammalian studies have reported that soy diets or the soy isoflavones (genistin, daidzin, and glycitin) could induce epigenetic modulation [Citation11,Citation16,Citation17].
Several studies carried out to evaluate the possibility of using pea protein concentrate (PPC) in aquafeeds have mainly reported favourable results [Citation18–Citation20]. Nevertheless, a study in Atlantic salmon revealed that a diet containing 35% PPC induced inflammation in the distal intestine, with symptoms similar to SBM-induced enteritis [Citation15]. Processing of PPC by dehulling and air classification can lower certain ANFs, like tannins but it can still contain protease inhibitors, phytate, α-galactosidases, and saponin [Citation13]. Supplementation of saponin to PPC caused enteritis and transcriptomic changes related to inflammation in the intestine of Atlantic salmon [Citation21].
Wheat gluten (WG) has also shown promising results as an alternative protein source for aquafeeds for several farmed fish species due to its inherent properties [Citation22]. It has a comparatively higher content of crude protein (even higher than the fishmeal), sulphur amino acids and glutamate than other commonly used plant protein sources [Citation22,Citation23]. Even though WG intolerance causes coeliac disease in humans, it did not cause enteritis in Atlantic salmon [Citation23].
Research on the dietary incorporation of plant proteins on the growth, nutrient utilization, and intestinal pathology has been performed in Atlantic salmon and other farmed fish species [Citation5,Citation19,Citation24] but many questions remain. The molecular effects of plant ingredients are not well understood and virtually nothing is known about the effect of plant ingredients on the epigenome of fish. Therefore, we investigated the nutriepigenomic effects of including plant-based proteins from pea, soy, and wheat into aquafeeds using zebrafish as a model. A genome-wide analysis of DNA methylation patterns was performed by reduced representation bisulphite sequencing (RRBS) to examine global epigenetic alterations in the mid intestine.
Results
Genome-wide intestinal methylation changes associated with plant protein diets in zebrafish
We assessed the epigenetic changes (DNA methylation) in the mid intestine of zebrafish in response to plant protein diets that included 30% pea protein concentrate (pea diet), soy protein concentrate (soy diet) and wheat gluten (wheat diet) and control diet containing fishmeal as the only protein source. RRBS generated 25–40 million raw reads per library, of which 43–51% were uniquely mapped to the bisulphite converted zebrafish genome GRCz11 (Supplementary Figure 1). We were able to analyse an average of 204.4 million cytosines per sample, including 40.6 million in CpG context. We observed an average of 78%, 0.8%, and 0.5% methylated cytosines, respectively, for CpG, CHG, and CHH contexts (Supplementary Table 1).
Differential methylation analysis of CpGs in the intestine of the pea, soy, and wheat diet groups in comparison to the control group revealed 1739, 3607, and 3568 significantly differentially methylated cytosines (DMCs) (>25%, q < 0.01) (), with similar proportions of hyper- and hypo-methylated cytosines. The highest frequency of methylation differences was observed between 25% and 34% and then between 35% and 44% for all three plant diets fed groups (Supplementary Figure 2).
Table 1. General statistics on significantly differentially methylated cytosines in CpG context with 10% coverage in the intestinal genome of zebrafish fed with plant protein–based diets.
Genomic feature analysis revealed that the number of DMCs in intergenic regions were higher than the number of DMCs in genic regions comprising promoter (−1 kb to +100 bp from the transcription start site, TSS), exons, introns, and transcription termination sites (TTS, −100 bp to +1 kb) ()) for all three plant diet groups. We could also find several differentially methylated repeats in the plant diet groups and they were present both in the genic and intergenic regions. The DNA repeat elements which composed of tandem repeats and transposons had the highest number of DMCs with equivalent proportion of hyper- and hypo-methylation (Figure 1(b)). In particular, long terminal repeat elements (LTR), which include retrotransposons, and short interspersed nuclear elements (SINE) showed considerably higher hypermethylation in all three groups fed with plant diets.
Figure 1. RRBS analysis of the mid intestine of zebrafish fed with diets containing 30% of PPC (pea), SPC (soy), or WG (wheat) in comparison to control fish fed with a fishmeal diet. (a) Genomic feature analysis of differentially methylated cytosines in plant-based diets. (b) Differentially methylated cytosines in different repeat elements in plant-based diets. TTS: Transcription termination site, LINE: Long interspersed nuclear elements, SINE: Short interspersed nuclear elements, DNA: DNA repeat elements and LTR: Long terminal repeat elements. For each diet, the number of total genomic features and the percentage of DMCs per genomic region (in brackets) are indicated above the respective bars.
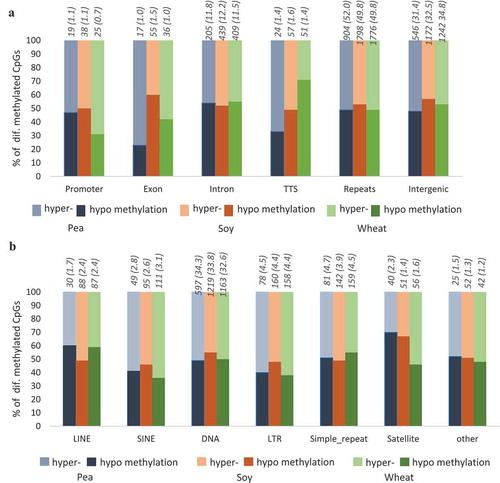
The total number of genes associated with differential methylation in both genic and intergenic regions of the intestinal genome of plant protein diet-fed fish are illustrated in . The soy diet group had the highest number of -such genes (2032), followed by wheat (1849) and pea (1020) diet groups. A total of 239 genes were common to all three plant diet groups.
Biological processes needed to maintain intestinal epithelial integrity and homoeostasis are enriched in fish fed with plant proteins
To understand the overall effect of the large number of DMCs in the intestine of fish fed with plant protein diets, we carried out a separate GO enrichment analysis for the genes associated with differential methylation in genic and intergenic regions. The summarized GO terms generated from enriched nonredundant biological GO terms from the three plant diet groups are presented in ,) for genic and intergenic regions, respectively. The complete list of non-redundant GO terms for each diet group is available in Supplementary Tables 2a and 2b for genic and intergenic regions, respectively.
Figure 3. Non-redundant-enriched GO biological processes determined for zebrafish fed with pea, soy, and wheat diets. Data are summarized as scatter plots using REVIGO tool. GO terms are marked with circles and plotted according to semantic similarities to other GO terms. The colour of the circles ranging from blue to red indicates the order of increase in log10 p-value. Circle sizes are proportional to the respective frequencies of the GO terms (circles of more general terms are larger). (a) Summarized GO terms for genes associated with the differential methylation in genic regions in fish fed with plant protein–based diets. (b) Summarized GO terms for genes associated with the differential methylation in intergenic regions in fish fed with plant protein–based diets. Small black circles indicate GO terms common to the three plant diet groups.
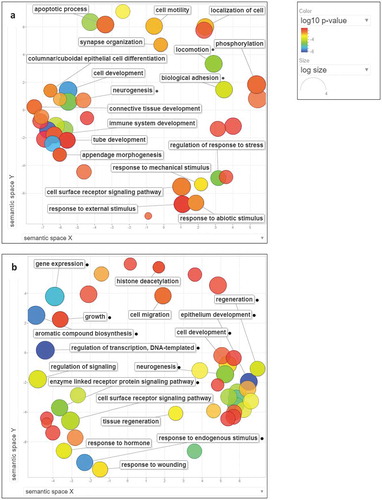
Neurogenesis (GO:0022008), biological adhesion (GO:0022610), and cell development (GO:0048468) processes were enriched in all three plant diet groups for the genes associated with differential methylation in genic regions. Pea and soy diet-fed groups indicated enrichment of biological processes related to locomotion (GO:0040011), cell motility (GO:0048870), and localization of cell (GO:0051674). Among the six GO terms common to soy and wheat diet-fed fish were regulation of response to stress (GO:0080134), synapse organization (GO:0050808), and appendage morphogenesis (GO:0035107). The pea diet group had exclusively enriched GO terms, including apoptotic process (GO:0006915), immune system development (GO:0002520), phosphorylation (GO:0016310), and response to external stimulus (GO:0009605). Among the GO terms enriched only in the soy diet group were cell surface receptor signalling pathway (GO:0007166), connective tissue development (GO:0061448), and tube development (GO:0035295). Wheat diet-fed fish showed uniquely enriched GO terms including columnar/cuboidal epithelial cell differentiation (GO:0002065), response to abiotic stimulus (GO:0009628), and response to mechanical stimulus (GO:0009612).
Most of the GO terms enriched for genes associated with differential methylation in intergenic regions were common to all three plant diet groups (), Supplementary Table 2b). Some of the common enriched processes were neurogenesis (GO:0022008), cell development (GO:0048468), epithelium development (GO:0060429), enzyme-linked receptor protein signalling pathway (GO:0007167), gene expression (GO:0010467), and regulation of transcription, DNA-templated (GO:0006355). Wheat diet-fed fish had a unique enrichment of biological process histone deacetylation (GO:0016575).
Feeding plant protein diets was associated with differential methylation in promoter regions of various genes
Differential methylation in promoter regions of several genes was observed in the three plant diet groups (). Hypermethylation of the promoter of semaphorin 3H (sema3h), grouped in biological process, negative chemotaxis (GO:0050919), was observed for all three plant diet groups. Pea and soy diet groups showed hypermethylation in the promoter of death-associated protein 3 (dap3), a serine/threonine kinase important for regulating several processes including apoptosis. The soy diet group also showed three hypermethylated cytosines in the promoter of rap2c, a member of the RAS oncogene family, which is important for the establishment of the endothelial intestinal barrier. The wheat diet group had hypermethylation in the promoters of some genes related to immunological process such as protein kinase C, beta a (prkcba) and Nedd4 family interacting protein 1, like (ndfip1l). Hypomethylation of promoter regions was observed for several genes involved in the immune-related processes, including tetraspanin 7b (tspan7b) in soy diet-fed fish.
Table 2. Selected genes associated with differential methylation in promoter regions in the intestinal genome of zebrafish fed with plant protein–based diets.
Several genes important for regulation of intestinal inflammation and homoeostasis linked to DMCs
A number of selected genes with more than two DMCs are shown in . Supplementary Table 3a indicates the percentage of methylation for each of the DMCs and Supplementary Table 3b presents the extended gene list. Among the genes shared by all three plant diet groups was TANK-binding kinase (tbk1), a serine/threonine kinase important in regulating inflammatory responses to foreign agents, which had more than 8 hypomethylated cytosines in the genic regions (pea-08, soy-18 and wheat-22). Furthermore, proteasome subunit alpha 1 (psma1), which plays a vital role in the immune process, showed notable hypomethylation. Mitogen-activated protein kinase kinase 4b (map2k4b), an activator in JNK pathway, showed hypermethylation in the pea and wheat gluten diet groups, but hypomethylation in the soy diet group. Common to the pea and soy diet groups was hyp-omethylation of peroxiredoxin 1 (prdx1), which is an important antioxidant enzyme. We also observed a number of unique genes for each plant protein–based diet group, among those genes with several DMCs in genic and/or intergenic regions ().
Table 3. Selected genes associated with several DMCs in the intestinal genome of zebrafish fed with plant protein–based diets.
Several immune genes, mainly interleukins and interferons, were methylated in genic and/or intergenic regions in the three plant protein–based diet groups (). We found considerable differences in the occurrence of DMCs in these genes between the groups. For instance, interleukin 1 receptor accessory protein-like 2 (il1rapl2) was differentially methylated in the pea and wheat groups, and interferon regulatory factor 4a (irf4a) differentially methylated in pea and soy groups. In contrast, differential methylation was observed in interleukin 10 receptor, beta (il10rb), major histocompatibility complex class I LAA (mhc1laa), chemokine (C-C motif) ligand 39, duplicate 3 (ccl39.3) and RELT, TNF receptor (relt) in fish of all diet groups.
Table 4. Immune genes affected by differential methylation in the intestinal genome of zebrafish fed with plant protein–based diets.
Histological observation of mild inflammation in the mid intestine induced by plant protein–based diets
The histological analysis of the mid intestine of zebrafish including controls ()) and fish fed with pea ()), soy ()) and wheat gluten ()) protein concentrates revealed mild inflammation-related symptoms in all three plant diet groups. Widening of the lamina propria could be observed in all plant diet-fed groups, whereas infiltration of intraepithelial immune cells was most prominent in intestine sections of fish from the soy diet group. Infiltration of immune cells further into the lamina propria was noted for the same group. In contrast, pea and wheat diet-fed fish showed increased infiltration levels of eosinophilic granulocytes.
Figure 4. Representative histological images from the mid intestines of zebrafish fed with a control diet containing fishmeal as the only protein source (a), and diets containing 30% plant-based proteins: PPC (b), SPC (c), and WG (d). Plant-based diets induced mild inflammation-related symptoms such as widened lamina propria (lp) and increased infiltration of immune cells (ic) in all plant diet groups, and increased infiltration of eosinophilic granulocytes (eg) in fish fed with PPC and WG diets. ec: enterocytes and gc: goblet cells.
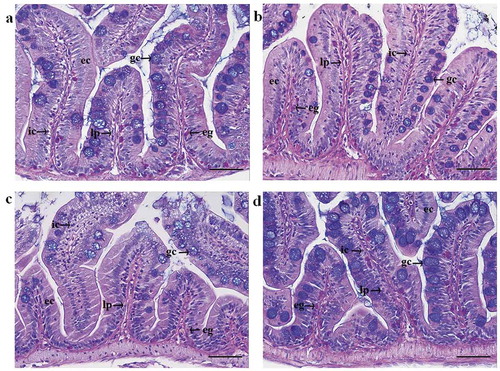
Systematic representation of epigenetic regulation of the intestinal genome by plant protein–based diets in zebrafish
The inclusion of 30% of pea or soy protein concentrate or wheat gluten in the diet induced epigenetic changes in the mid intestine of zebrafish (). The nuclear factor‐κB (NF‐κB) is important for the regulation of genes involved in inflammation, apoptosis, and cell proliferation, amongst others. Activation of NF‐κB occurs through degradation of IκB proteins by the IκB-kinase (IKK) complex via the ubiquitin-proteasome pathway. All three plant-based diet groups showed hypomethylation of IKK tbk1, indicating a possible epigenetic regulation of NF‐κB activity. Furthermore, the plant diet groups displayed differential methylation of psma1, an essential component of proteasome. The soy- and wheat-diet groups showed changes in methylation of genes involved in the ubiquitination system, namely fbxo28 and keap1b. Moreover, a member of the MAPK kinase family, map2k4b, was differentially methylated in fish fed with plant-based diets, potentially regulating the cellular stress response. Genes coding for the antioxidant enzymes prdx1 (pea and soy) and txnrd3 (wheat) were differentially methylated, suggesting a probable control of the antioxidant defence by plant diets. In addition, GO analysis revealed a possible epigenetic regulation of neurogenesis, cell adhesion, and response to stress and immunity in fish fed with plant diets.
Figure 5. Systematic representation of the epigenetic regulation of intestinal genome by inclusion of 30% pea or soy protein concentrates or wheat gluten in the diet of zebrafish. Induced epigenetic changes in the mid intestine affected the nuclear factor‐κB (NF‐κB), IκB proteins, IκB-kinase (IKK), and the MAPK kinase family, which are involved in the regulation of different cellular processes.
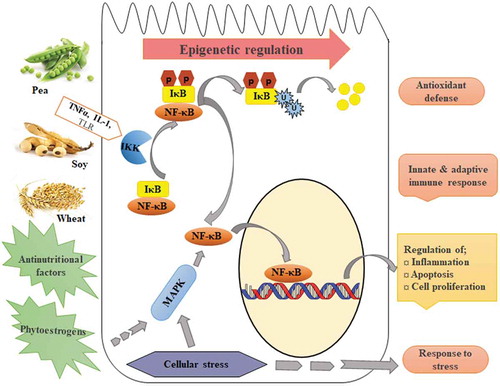
Discussion
This pioneer study revealed that partial inclusion of plant-based protein into the diet induced genome-wide epigenetic changes in the mid intestine of zebrafish. Diet-related epigenetic changes have been described in relation to feeding of high fat, low protein or energy-restricted diets or phytochemicals, mostly in cell culture and mammalian models [Citation2,Citation10,Citation25]. So far, only one other study has reported a diet-related epigenetic change in fish, i.e., Skjærven et al. [Citation26] showed that the parental 1-C nutrient status was linked to genome-wide DNA methylation changes in their offspring.
A large number of cytosines were differentially methylated in the intestinal genome of zebrafish fed with plant protein ingredients compared to the control diet. They were found both in genic and intergenic regions, the latter being predominant. Genome feature analysis also assigned intergenic cytosines to genes with the nearest TSS. DNA methylation in different genomic regions can potentially affect gene expression leading to cellular and phenotypical changes in the organism [Citation27]. It is generally believed that methylation in promoter regions is inversely correlated with the expression rate of genes [Citation28], but it can vary with specific promoter sequences and gene functions [Citation29]. On the other hand, methylation in gene bodies is often positively correlated with gene expression levels, and it is thought that this methylation blocks the transcription of repetitive DNA elements. Gene body methylation is also important in the regulation of alternative promoters and splicing [Citation30,Citation31]. Similar to other animal genomes, approximately 50% of the zebrafish genome consists of intergenic regions, which have important regulatory roles [Citation32]. Methylation of intergenic regions can silence transposable and viral elements to prevent possible gene disruption and DNA mutation caused by their expression [Citation27]. Active transposable elements can result in mutations, chromosome breakage, illegitimate recombination, and genome rearrangements [Citation33]. Epigenetic mechanisms can contribute to maintain the genome architecture integrity by silencing these transposable elements. Moreover, transposable elements are increasingly recognized a part of the regulatory toolkit of the genome, with important roles in directing gene expression. It is reported that environment and stress can activate transposable elements either directly or indirectly by the inhibition of gene-silencing mechanisms [Citation33]. In mice, dietary supplementation of a methyl donor changed the coat colour of the offspring as a result of the alteration in a transposable element-induced epiallele of the agouti gene [Citation34]. Comparative methylome analysis of mouse and zebrafish liver and brain revealed higher CpG methylation in zebrafish for both tissues, possibly due to the fact that a higher percentage of the zebrafish genome is occupied by transposable elements (52% compared to 42% in mouse) [Citation35]. The genome-wide methylation differences within transposable elements (e.g., LINE, SINE, and LTR) observed in our study could be attributed to several factors, including exposure to phytochemicals and phytoestrogens present in the plant diets compared to the control diet. High differential methylation in intergenic regions has also been reported in zebrafish exposed to other chemical substances and environmental conditions [Citation36–Citation38]. The DNA methylation pattern of a tissue depends on the cellular phenotype, which is influenced by environmental perturbations [Citation39]. Our qualitative histological analysis revealed increased infiltration of intraepithelial immune cells and eosinophilic granulocytes in the intestine of fish fed with plant protein–based diets compared to the control fishmeal diet. Hence, the genome-wide DNA methylation differences observed in fish fed with plant ingredients may be, at least partly, cause or consequence of changes in cell composition.
Gene ontology enrichment analysis of genes associated with differentially methylated cytosines indicated that several biological processes were enriched in fish fed with plant protein–based diets. Among them were neurogenesis (in all three diet groups) and synapse organization (soy and wheat diet groups), which are of particularly interest regarding the enteric nervous system (ENS). The ENS is well conserved in all vertebrates, although teleost has a morphologically rather simple ENS devoid of well-organized ganglia [Citation40]. It is involved in the regulation of several gastrointestinal functions including motility, mucosal secretion and absorption, blood flow, epithelial barrier and epithelial proliferation, and differentiation [Citation41]. Dietary nutrients including glucose, lipids, and amino acids (glutamate, glycine, and γ-aminobutyric acid) are known to have direct and indirect influence on the ENS, eventually affecting ENS-controlled gut functions [Citation42]. Glutamate is an excitatory neurotransmitter for the ENS and plays a role in neuronal differentiation, synaptic maintenance, and plasticity. Generally, plant proteins and specially wheat gluten contain higher levels of glutamate compared to fishmeal [Citation18,Citation22]. In addition, pea contains acetylcholine, which is another neurotransmitter [Citation43]. Therefore, it could be possible that the higher levels of glutamate and other neurotransmitters in plant-derived protein concentrates can influence the methylation status of genes involved in neurogenesis and synapse organization, ultimately having a potential impact on the ENS and related functions.
Another interesting process enriched in the three fish groups fed with plant-based diets is biological adhesion/cell adhesion. Cadherins are calcium-dependent cell adhesion molecules, which form a large and diverse group and are involved in the formation of adherens junctions together with catenin. The cadherins–catenin complex is important for the perception of the microenvironment and the mediation of major intracellular signalling pathways important for tissue/epithelial homoeostasis and morphology [Citation44,Citation45]. It was suggested that during the development of SBM-induced enteritis, saponins may increase gut permeability, resulting in a weakened intestinal epithelial barrier and increased exposure of the local immune system to various antigens [Citation46–Citation48]. However, high dietary inclusion of plant proteins that are generally low in saponins such as PPC (35% inclusion) and bean protein concentrate (45% inclusion) resulted also in gut inflammation resembling SBM-induced enteritis [Citation14,Citation15]. These findings indicate that the overall loss of intestinal integrity by different ANF/antigens present in plant diets may trigger gut inflammation [Citation49]. This hypothesis is supported by our findings in the present study that showing differential methylation in several members of the cadherin family (cdh1, cdh2, cdh7, cdh10a, cdh11, cdh15) and catenin (ctnna2), indicating a likely epigenetic regulation of the intestinal integrity in fish fed with plant protein–based diets. Epigenetic adaptations to maintain intestine integrity as well as to regulate the immune response (as detailed in the following sections) may help the fish to keep the intestinal inflammation at a mild level, preventing it from developing into severe enteritis, as evidenced by our histological analysis of the mid intestine of plant protein–based diets fed zebrafish. Moreover, we observed the enrichment of additional processes that are required to maintain gut homoeostasis, including stress responses (soy and wheat diet groups), responses to external stimuli (pea diet group), responses to abiotic stimuli and mechanical stimuli and responses to oxidative stress (wheat diet group). Interestingly, the pea diet group alone showed an enrichment of some biological processes, such as apoptosis and immune system development. Nevertheless, the intestinal genome of fish from all three plant diet groups had a number of immune or immune-related genes with several DMCs in the genic and/or intergenic regions. Among them were a number of cytokines [Citation49], and/or their receptors and related proteins. Those include family members of the β-Trefoil cytokines (il1rapl2), Jellyroll cytokines (tnfa & relt), type I helical cytokines (il6st & il15) and type II α-helical cytokines (ifnlr1, irf2bpl, irf4a & ifnphi3). This indicated the possible impact of plant diets on the epigenetic regulation of genes of the innate as well as the adaptive immune system in the mid intestine of adult zebrafish. On the other hand, epigenetic changes in those genes may also have been a consequence of the changes in cell-type composition, as evidenced by the histological analysis of the mid intestine of fish from the three plant diet groups. The immunological response of fish to PPC [Citation15] and SPC [Citation14] -containing diets has been previously described based on histological, physiological, and transcriptomic analyses in the respective studies. Zebrafish larvae fed diets containing 30% of soy protein isolate reported increased expression of proinflammatory cytokines [Citation50]. On the other hand, diets with 35% wheat gluten did not show any significant immunopathological changes in the gut of Atlantic salmon [Citation23].
We identified several genes that are associated with differential methylation in promoter regions. One example is sema3h, which showed hypermethylation of its promoter in all plant diet groups. Sema3h belongs to class 3 semaphorins, which play a vital role in the zebrafish development [Citation51]. It has also been reported in mammals that class 3 semaphorins interact with neuropilins in dendric cells and initiate an immune response to antigen exposure [Citation52]. Therefore, the differential methylation observed in sema3h, neuropilin 1 (4 DMCs in pea diet group) and neuropilin 2 (1, 2 and 17 DMCs in pea, soy, and wheat diet groups, respectively) in the zebrafish intestine may be linked to the presence of antigens in plant-based diets.
Some genes in the intestinal genome of fish fed with plant-based diets are associated with a large number of DMCs, and those genes could represent potential epigenetic markers. Among them were components of the mitogen‐activated protein kinase (MAPK) pathway and several members of the ubiquitin system as well as the ubiquitin-proteasome system. Moreover, some genes were associated with antioxidant defence and metabolic processes. Some of those genes were common to all three plant diet groups, while others were specific for particular diets. It is plausible that their epigenetic regulation plays an important role in regulating intestinal inflammation and maintaining homoeostasis. One such gene is Tank-binding kinase 1 (tbk1), which was associated with more than 8 hypomethylated cytosines in its gene body, mostly in the introns, in all zebrafish fed with plant-based diets. It is involved in the regulation of the nuclear factor‐κB (NF‐κB), which is a transcription factor important for the regulation of genes involved in inflammation and immunity [Citation53,Citation54]. Activation of NF‐κB occurs through degradation of inhibitory κB (IκB) proteins by the IκB-kinase (IKK) complex via the ubiquitin-proteasome system [Citation53]. Tbk1 is a IκB-related kinase that activates NF‐κB after forming a complex with the IκB proteins Tank and Traf2 [Citation54]. Studies also revealed that a high level of Tbk1 triggered an MAPK phosphatase‐1-mediated negative feedback pathway to control inflammation [Citation55]. The epigenetic regulation of tkb1 may be involved in controlling the inflammatory response triggered by plant protein–based diets and in maintaining the intestinal homoeostasis in zebrafish. Additionally, MAPK kinase 4b (map2k4b), a member of the MAPK kinase family, showed differential methylation in the three groups receiving plant protein–based diets. Response to external stimuli in eukaryotic cells is mainly triggered by activating MAPK signalling pathways, namely the extracellular signal-regulated kinase (ERK or MAPK), p38 kinases, and c-Jun NH2-terminal kinases (JNK) [Citation56]. Map2k4b directly phosphorylates and activates JNK in response to cellular stress and proinflammatory cytokines [Citation56]. Therefore, the epigenetic modifications observed in the JNK activator map2k4b may affect cellular responses such as apoptosis and inflammation in the intestine, as well as the local stress response.
Similarly, we also detected differential methylation in the proteasome subunit alpha type-1 (psma1) in all three plant-based diet groups. Psmal is a part of the eukaryotic proteasome, a well-organized protease complex, which interacts with the ubiquitin system and controls nearly all basic cellular processes including cell cycle, signal transduction, cell death, immune response, and metabolism [Citation57,Citation58]. The Ubiquitin-proteasome system also controls the inflammatory response by activating NF-κB [Citation53] and regulating leukocyte proliferation [Citation59]. Therefore, potential regulation of psma1 through differential methylation induced by plant diets could be important for modulation of the inflammatory response and other cellular processes that depend on the ubiquitin-proteasome system.
Fish fed with soy protein concentrate- or wheat gluten-containing diets displayed different methylation patterns of genes in the ubiquitination complex: fbxo28 and keap1b, respectively. The F-box protein is part of a major type of E3 ubiquitin ligases, SCF (Skp–Cullin–F-box) complex [Citation60]. It is responsible for recognizing different substrates for ubiquitination and is involved in the regulation of protein stability and a wide range of cellular processes [Citation60]. Interestingly, fish from the soy diet group showed 21 hypermethylated cytosines in the gene body of fbxo28. In contrast, Keap1, a different adaptor for the cullin-based E3 ubiquitin ligase complex [Citation61] was differentially methylated in the wheat gluten-fed group. Keap1 is an oxidative stress sensor and important for the rapid degradation of the transcript factor Nrf2 (NF-E2-related factor 2) through the ubiquitin-proteasome system [Citation61]. Nrf2 activates the antioxidant response by inducing transcription of a variety of genes that are able to combat the harmful effects of oxidative stress. When the stressful stimuli are over, Nrf2 is maintained at basal levels by Cul3-Keap1-E3 ligase [Citation62]. Wheat gluten contains a very high level of glutamate, which is an important precursor for glutathione, a vital molecule for the antioxidant defence [Citation63]. Possibly, epigenetic modulation of keap1b could be associated with potential intracellular antioxidant activity triggered by the high glutamate levels in wheat gluten. Furthermore, the wheat gluten diet was also associated with the epigenetic regulation of thioredoxin reductase (txnrd3), which is an important component of the antioxidant defence thioredoxin complex involved in the control of oxidative stress and apoptosis [Citation64]. Thioredoxin reductase maintains thioredoxin in the reduced state in an NADPH-dependent reaction, and thioredoxin reductase 3 can use glutathione as a substrate [Citation65]. Txnrd3 is also a target of the Wnt pathway, which is important for regulating the continuous renewal of the intestinal epithelium [Citation65]. We observed the epigenetic regulation of txnrd3 only in the wheat diet group, which could be associated with the high glutamate content in this feed. The antioxidative enzyme peroxiredoxin 1 (Prdx1) protects cells from oxidative damage by reducing peroxides. It also functions as a molecular chaperone under stressful conditions [Citation66,Citation67]. The intestinal genomes of both pea- and soy-fed fish showed the same 16 hypomethylated cytosines in the intergenic regions close with prdx1. These modifications could be linked to the high antioxidant activity of isoflavones (e.g., genistein and daidzein) present in plant ingredients, especially in soy [Citation68].
There is increasing evidence that fish respond to nutritional programing, but the molecular basis of this cellular memory is not fully understood. For instance, short-term feeding of rainbow trout (Oncorhynchus mykiss) fry with a plant-based diet improved their feed intake by 30% and growth rate by 42% when the same diet was provided to adult fish [Citation69]. It is plausible that the diet-associated DNA methylation changes observed in the intestine of zebrafish have the properties of epigenetic memory [Citation70], which would enable their adaptation to plant-based feeds containing phytochemicals and different amino acid profiles.
Conclusions
Using an RRBS approach, we were able to show that partial inclusion of plant-based proteins into the diet-induced genome-wide changes in DNA methylation in the mid intestine of zebrafish. A large number of genes were differentially methylated by exposure to pea or soy protein concentrate- and wheat gluten-containing diets, both in genic and intergenic regions, with the latter being the most predominant. All fish displayed epigenetic changes in genes related to neurogenesis, which is especially interesting with respect to the enteric nervous system. Methylation changes in genes related to biological adhesion/cell adhesion could indicate an adaptation to maintain epithelial integrity. In addition, the fish showed a large number of DMCs in several genes associated with the regulation of inflammation and intestinal homoeostasis. Among those were genes related to NF‐κB, the ubiquitin-proteasome, MAPK pathways, and the antioxidant defence system. Some differentially methylated genes varied among diet groups, indicating that specific plant ingredients can have unique effects on the intestine methylome. These epigenetic adaptations could help the zebrafish to rapidly regulate inflammation and maintain intestinal homoeostasis when fed with plant protein–based diets. The knowledge generated from this pioneer study can be of benefit for the aquaculture industry in the search of alternative feed ingredients.
Material and methods
Feeding trials
All custom-made diets were produced by extrusion at the Nofima Feed Technology Centre, Fyllingsdalen, Norway as detailed by Johny et al. [Citation68]. The control fishmeal diet included 79.4% fish meal as the protein source along with 4% of fish oil, 12% of wheat as the binding source, and 4.6% of other additives containing monosodiumphosphate – 24% P, vitamin, and mineral mix. Plant protein–based diets were prepared by replacing 30% fishmeal by PPC, SPC, or WG as protein source. Feed analysis indicated that all diets had comparatively similar protein (50–59%) and lipid (9–11%) contents [Citation68].
Feeding trials were conducted for 46 days at Nord University, Norway, using four-month-old zebrafish (AB strain) with a mean weight of 0.214 ± 0.027 g at the start of the experiment. Each feeding group was allocated to four randomly distributed 3.5 L tanks containing 16 fish (1:1 sex ratio) in a flow-through system with 20% water exchange per hour (ZebTEC Stand-Alone Toxicology Rack; Techniplast, London, UK). They were maintained under the standard husbandry conditions, including a stable temperature of 28 ± 0.5°C, pH 7.5, water conductivity of 1500 µS/cm, and a photoperiod of 12 h light:12 h dark. Fish were fed twice a day with a total feed amount equal to 2.5% (w/w) of their body weights, and their feeding behaviour and health were regularly controlled. Feeding was stopped 24 h prior to sampling.
During sampling, the fish in each tank were separated by gender and weighed. Fish were euthanized with a lethal dose of 200 mg/L tricaine methanesulfonate (MS222) (Sigma-Aldrich, St. Louis, MO, USA), buffered with an equal amount of sodium bicarbonate. The mid intestine was carefully dissected under a light microscope from three fish per tank and preserved in neutral buffered 10% (v/v) formalin at 4°C for histological analysis. The remaining fish were immediately frozen in liquid nitrogen and stored at −80°C. The mid intestine was dissected out from the frozen specimens for RRBS analysis. We randomly selected five female fish including the four tanks per treatment. We used only female fish for the RRBS analysis to avoid sex effects that might interfere with the interpretation of epigenetic changes induced by plant-based diets.
Reduced representation bisulphite sequencing
For RRBS analysis, genomic DNA was extracted using the E.Z.N.A insect DNA kit (Omega Bio-tek, Norcross, GA, USA) following the protocol from the manufacturer. Quantity and quality of the DNA were analysed using a qubit fluorometer (Invitrogen, Thermo Fisher Scientific, Waltham, MA, USA) and Agilent 2200 TapeStation (Agilent Technologies, Santa Clara, CA, USA), respectively.
Library preparation for RRBS was performed with the NuGen ovation RRBS methyl-seq system 1–16 (Tecan Genomics, Inc, Redwood City, CA, USA), following the manufacturer’s instructions. Genomic DNA was digested with MspI at 37°C for 1 h, followed by adapter ligation and final repair. For the bisulphite conversion of adapter-ligated libraries and subsequent cleaning, the EpiTect fast bisulphite conversion kit (Qiagen, Hilden, Germany) was used following the manufacturer’s protocol. The resulting bisulphite converted libraries were amplified with 12 PCR cycles and later purified, utilizing reagents and recommendations of the NuGen RRBS kit. Quality and quantity of the RRBS libraries were assessed using the TapeStation. Two sequencing runs were performed for two pools containing 10 libraries, each with equal representation from the fishmeal control and three plant protein–based diets, on an Illumina NextSeq platform (San Diego, CA, USA) at FBA, Nord University, using a single-end 75 bp high-throughput sequencing kit with 4% of Phix control DNA (Illumina) as an internal control, following the instructions for RRBS sequencing. The sequence data were deposited in the NCBI Sequence Read Archive (SRA) with the reference number (PRJNA577226).
RRBS data analysis
Raw sequencing data were processed for quality and diversity adapter trimming using Trim Galore (Babraham Bioinformatics) without the – rrbs option, according to NuGEN’s data analysis protocol. The diversity adapters attached to the 3ʹ end of the reads were removed with a specific script provided by NuGen. This preserves the first base of the MspI fragment, which contains a CpG methylation measurement. Quality trimmed reads were aligned to the zebrafish genome (GRCz11, NCBI) using Bismark [Citation71] allowing less than three mismatches per 100 bp reads, directional option, and with all the other default parameters. Methylation information was extracted using the – cytosine_report option generating a genome-wide methylation report for all cytosines in the genome.
Differentially methylated cytosines in CpG context between the genomes of zebrafish fed with plant protein–based diets in comparison to the marine control diet were analysed using the methylKit package [Citation72]. Methylation information was read from the CpG report generated from the Bismark aligner by specifying the – bismarkCytosineReport in methRead function of methylKit. Samples were filtered based on coverage discarding all reads below 10% coverage, and above the 99.9th percentile of coverage with the aim of avoiding PCR bias. DMCs were extracted by logistic regression with the statistical criteria of q value ≤0.01 and methylation differences ≥25%.
Functional annotation and gene ontology analysis of DMCs
DMC annotation was performed using the HOMER package [Citation73]. It should be noted that overlapping annotations were prioritized based on the order by HOMER: transcription start sites (TSS, including promoter regions −1 kb to +100 bp from TSS) > transcription termination sites (TTS, from – 100 bp to +1 kb) > exon (coding) > 5ʹ UTR exon > 3ʹ UTR exon > CpG islands > repeats > introns > intergenic regions. In the case of methylated cytosines in intergenic regions, HOMER identifies and assigns them to the gene with the nearest TSS.
Gene ontology enrichment analysis (GO) was carried out separately for genes associated with differential methylation in (i) promoter, exon, intron, and TTS, and (ii) intergenic regions. Initially, enriched GO fat terms were extracted with an EASE Score (a modified Fisher exact p-value) of 0.01 using DAVID online tool [Citation74,Citation75]. They were then summarized by removing redundant GO terms and visualized in semantic similarity-based scatterplots using REVIGO online tool [Citation76].
Histology
Standard histological techniques were used for dehydration in ethanol, equilibration in xylene and preparation of paraffin-embedded intestines from six female fish including at least one fish from four replicate tanks per dietary treatment. Five-micrometre thick longitudinal sections were stained with Alcian Blue-Periodic Acid Schiff’s reagent (AB-PAS, pH 1.0, Sigma-Aldrich, Missouri, USA) using a robot slide stainer (Microm HMS 760×, MICROM International GmbH, Walldorf, Germany) as described by Bancroft and Gamble [Citation77]. Photomicrographs were captured with an Olympus BX61/Camera Color View IIIu (Olympus Europa GmbH, Hamburg, Germany) and processed using the imaging system Cell P (Soft Imaging System GmbH, Münster, Germany).
Authors’ Contributions
AD: Designed the study, conducted the feeding trials and sampling, performed the laboratory work, the bioinformatics analysis and interpretation of the results, and wrote the manuscript.
XC: Contributed significantly to the laboratory work.
DD: Performed the histological analysis.
PS: Contributed significantly to conducting the feeding trials and sampling.
CKF: Conceived and designed the study, provided the reagents, and revised the manuscript.
JF: Conceived and designed the study, provided the reagents, contributed significantly to the bioinformatics analysis and interpretation of the results, and revised the manuscript.
All authors read and approved the manuscript.
Ethics Statement
All experiments involving zebrafish were conducted in agreement with the guidelines provided by the Norwegian Animal Research Authority (FOTS ID 12581, 27 July 2017) and approved by the Nord University (Norway) ethics committee.
Supplemental Material
Download MS Word (61.9 KB)Supplemental Material
Download MS Word (32.5 KB)Acknowledgments
A. S. Bogevik at Nofima (Bergen, Norway) is acknowledged for preparing the zebrafish feed. Tomasz Podgorniak at Norwegian University of Life Sciences (Oslo, Norway) and Ioannis Konstantinidis at the Nord University (Bodø, Norway) are thanked for their recommendations during the bioinformatics analysis.
Disclosure Statement
The authors reported no potential conflicts of interest.
Supplemental Material
Supplemental data for this article can be accessed here.
Additional information
Funding
References
- Bird A. Perceptions of epigenetics. Nature. 2007;447(7143):396–398.
- Kadayifci FZ, Zheng S, Pan Y-X. Molecular mechanisms underlying the link between diet and DNA methylation. Int J Mol Sci. 2018;19(12):4055.
- Zhang N. Epigenetic modulation of DNA methylation by nutrition and its mechanisms in animals. Anim Nutr. 2015 Sept 1;1(3):144–151.
- Hardy RW. Utilization of plant proteins in fish diets: effects of global demand and supplies of fishmeal. Aquac Res. 2010;41:770–776.
- Gatlin DM, Barrows FT, Brown P, et al. Expanding the utilization of sustainable plant products in aquafeeds: a review. Aquac Res. 2007;38:551–579.
- Ytrestøyl T, Aas TS, Åsgård T. Utilisation of feed resources in production of Atlantic salmon (Salmo salar) in Norway. Aquaculture. 2015;448:365–374.
- FAO. The state of world fisheries and aquaculture 2016: contributing to food security and nutrition for all. Rome; 2016. FAO.
- Krogdahl Å, Penn M, Thorsen J, et al. Important antinutrients in plant feedstuffs for aquaculture: an update on recent findings regarding responses in salmonids. Aquac Res. 2010;41(3):333–344.
- Francis G, Makkar HPS, Becker K. Antinutritional factors present in plant-derived alternate fish feed ingredients and their effects in fish. Aquaculture. 2001;199:197–227.
- Shankar S, Kumar D, Srivastava RK. Epigenetic modifications by dietary phytochemicals: implications for personalized nutrition. Pharmacol Ther. 2013;138(1):1–17.
- Rietjens IMCM, Sotoca AM, Vervoort J, et al. Mechanisms underlying the dualistic mode of action of major soy isoflavones in relation to cell proliferation and cancer risks. Mol Nutr Food Res. 2013;57(1):100–113.
- Zhou Z, Ringø E, Olsen RE, et al. Dietary effects of soybean products on gut microbiota and immunity of aquatic animals: a review. Aquac Nutr. 2018;24(1):644–665.
- Drew MD, Borgeson TL, Thiessen DL. A review of processing of feed ingredients to enhance diet digestibility in finfish. Anim Feed Sci Technol. 2007 Oct 22;138(2):118–136.
- Król E, Douglas A, Tocher DR, et al. Differential responses of the gut transcriptome to plant protein diets in farmed Atlantic salmon. BMC Genomics. 2016;17:156.
- Penn MH, Bendiksen EÅ, Campbell P, et al. High level of dietary pea protein concentrate induces enteropathy in Atlantic salmon (Salmo salar L.). Aquaculture. 2011;310:267–273.
- Howard TD, Ho S-M, Zhang L, et al. Epigenetic changes with dietary soy in cynomolgus monkeys. PLoS One. 2011;6(10):e26791.
- Day JK, Bauer AM, desBordes C, et al. Genistein alters methylation patterns in mice. J Nutr. 2002;132(8):2419S–2423S.
- Øverland M, Sørensen M, Storebakken T, et al. Pea protein concentrate substituting fish meal or soybean meal in diets for Atlantic salmon (Salmo salar)—Effect on growth performance, nutrient digestibility, carcass composition, gut health, and physical feed quality. Aquaculture. 2009 Mar 20;288(3):305–311.
- Burel C, Boujard T, Tulli F, et al. Digestibility of extruded peas, extruded lupin, and rapeseed meal in rainbow trout (Oncorhynchus mykiss) and turbot (Psetta maxima). Aquaculture. 2000;188(3–4):285–298.
- Zhang Y, Øverland M, Sørensen M, et al. Optimal inclusion of lupin and pea protein concentrates in extruded diets for rainbow trout (Oncorhynchus mykiss). Aquaculture. 2012;344–349:100–113.
- Kortner TM, Skugor S, Penn MH, et al. Dietary soyasaponin supplementation to pea protein concentrate reveals nutrigenomic interactions underlying enteropathy in Atlantic salmon (Salmo salar). BMC Vet Res. 2012 July 02;8(1):101.
- Apper-Bossard E, Feneuil A, Wagner A, et al. Use of vital wheat gluten in aquaculture feeds. Aquat Biosyst. 2013;9:21.
- Storebakken T, Shearer KD, Baeverfjord G, et al. Digestibility of macronutrients, energy and amino acids, absorption of elements and absence of intestinal enteritis in Atlantic salmon, Salmo salar, fed diets with wheat gluten. Aquaculture. 2000;184(1–2):115–132.
- Carter CG, Hauler RC. Fish meal replacement by plant meals in extruded feeds for Atlantic salmon, Salmo salar L. Aquaculture. 2000;185(3–4):299–311.
- McKay JA, Mathers JC. Diet induced epigenetic changes and their implications for health. Acta Physiol. 2011;202(2):103–118.
- Skjærven KH, Jakt LM, Fernandes JMO, et al. Parental micronutrient deficiency distorts liver DNA methylation and expression of lipid genes associated with a fatty-liver-like phenotype in offspring. Sci Rep. 2018 Feb 14;8(1):3055.
- Moore LD, Le T, Fan G. DNA methylation and its basic function. Neuropsychopharmacology. 2013;38(1):23–38.
- Jones PA. Functions of DNA methylation: islands, start sites, gene bodies and beyond. Nat Rev Genet. 2012 May 29 online;13:484.
- Weber M, Hellmann I, Stadler MB, et al. Distribution, silencing potential and evolutionary impact of promoter DNA methylation in the human genome. Nat Genet. 2007 Mar 04 online;39:457.
- Maunakea AK, Nagarajan RP, Bilenky M, et al. Conserved role of intragenic DNA methylation in regulating alternative promoters. Nature. 2010 July 08 online;466:253.
- Jjingo D, Conley AB, Yi SV, et al. On the presence and role of human gene-body DNA methylation. Oncotarget. 2012;3(4):462–474.
- Francis WR, Wörheide G. Similar ratios of introns to intergenic sequence across animal genomes. Genome Biol Evol. 2017;9(6):1582–1598.
- Slotkin RK, Martienssen R. Transposable elements and the epigenetic regulation of the genome. Nat Rev Genet. 2007 Apr 01;8(4):272–285.
- Cooney CA, Dave AA, Wolff GL. Maternal methyl supplements in mice affect epigenetic variation and DNA methylation of offspring. J Nutr. 2002;132(8):2393S–2400S.
- Zhang C, Hoshida Y, Sadler KC. Comparative epigenomic profiling of the DNA methylome in mouse and zebrafish uncovers high interspecies divergence. Front Genet. 2016;7:110.
- Kamstra JH, Sales LB, Aleström P, et al. Differential DNA methylation at conserved non-genic elements and evidence for transgenerational inheritance following developmental exposure to mono(2-ethylhexyl) phthalate and 5-azacytidine in zebrafish. Epigenetics Chromatin. 2017;10:20.
- Falisse E, Ducos B, Stockwell PA, et al. DNA methylation and gene expression alterations in zebrafish early-life stages exposed to the antibacterial agent triclosan. Environ Pollut. 2018 Dec 01;243:1867–1877.
- Lappalainen T, Greally JM. Associating cellular epigenetic models with human phenotypes. Nat Rev Genet. 2017 July;18(7):441–451.
- Olsson C. Autonomic innervation of the fish gut. Acta Histochem. 2009 May 01;111(3):185–195.
- Furness JB. The enteric nervous system and neurogastroenterology. Nat Rev Gastroenterol Hepatol. 2012 May 01;9(5):286–294.
- Neunlist M, Schemann M. Nutrient-induced changes in the phenotype and function of the enteric nervous system. J Physiol. 2014;592(14):2959–2965.
- Briguglio M, Dell’Osso B, Panzica G, et al. Dietary neurotransmitters: a narrative review on current knowledge. Nutrients. 2018;10(5):591.
- Maître J-L, Heisenberg C-P. Three functions of cadherins in cell adhesion. Curr Biol. 2013;23(14):R626–R633.
- Klezovitch O, Vasioukhin V. Cadherin signaling: keeping cells in touch. F1000Res. 2015;4:550.
- Knudsen D, Jutfelt F, Sundh H, et al. Dietary soya saponins increase gut permeability and play a key role in the onset of soyabean-induced enteritis in Atlantic salmon (Salmo salar L.). Br J Nutr. 2008;100(1):120–129.
- Martin SAM, Dehler CE, Król E. Transcriptomic responses in the fish intestine. Dev Comp Immunol. 2016 Nov 01;64:103–117.
- Zou J, Secombes CJ. The function of fish cytokines. Biology (Basel). 2016;5(2):23.
- Fuentes-Appelgren P, Opazo R, Barros L, et al. Effect of the dietary inclusion of soybean components on the innate immune system in zebrafish [Article]. Zebrafish. 2014;11(1):41–49.
- Stevens CB, Halloran MC. Developmental expression of sema3G, a novel zebrafish semaphorin. Gene Expr Patterns. 2005 June 1;5(5):647–653.
- Roy S, Bag AK, Singh RK, et al. Multifaceted role of neuropilins in the immune system: potential targets for immunotherapy. Front Immunol. 2017 Oct 10;8:1228.
- Karin M, Delhase M. The IκB kinase (IKK) and NF-κB: key elements of proinflammatory signalling. Semin Immunol. 2000 Feb 1;12(1):85–98.
- Pomerantz JL, Baltimore D. NF-kappaB activation by a signaling complex containing TRAF2, TANK and TBK1, a novel IKK-related kinase. Embo J. 1999;18(23):6694–6704.
- Kim E, Yoon JY, Lee J, et al. TANK-binding kinase 1 and Janus kinase 2 play important roles in the regulation of mitogen-activated protein kinase phosphatase-1 expression after toll-like receptor 4 activation. J Cell Physiol. 2018;233(11):8790–8801.
- Cuenda A. Mitogen-activated protein kinase kinase 4 (MKK4). Int J Biochem Cell Biol. 2000 June 01;32(6):581–587.
- Hershko A, Ciechanover A. The ubiquitin system. Annu Rev Biochem. 1998;67(1):425–479.
- Ben-Neriah Y. Regulatory functions of ubiquitination in the immune system. Nat Immunol. 2002 Jan 01 online;3:20.
- Cron KR, Zhu K, Kushwaha DS, et al. Proteasome inhibitors block DNA repair and radiosensitize non-small cell lung cancer. PLoS One. 2013;8(9):e73710.
- Ho MS, Tsai P-I, Chien C-T. F-box proteins: the key to protein degradation. J Biomed Sci. 2006 March 01;13(2):181–191.
- Kobayashi A, Kang M-I, Okawa H, et al. Oxidative stress sensor Keap1 functions as an adaptor for Cul3-based E3 ligase to regulate proteasomal degradation of Nrf2. Mol Cell Biol. 2004;24(16):7130–7139.
- Villeneuve NF, Lau A, Zhang DD. Regulation of the Nrf2-Keap1 antioxidant response by the ubiquitin proteasome system: an insight into cullin-ring ubiquitin ligases. Antioxid Redox Signal. 2010;13(11):1699–1712.
- Newsholme P, Lima MMR, Procopio J, et al. Glutamine and glutamate as vital metabolites. Braz J Med Biol Res. 2003;36:153–163.
- Arnér ESJ, Holmgren A. Physiological functions of thioredoxin and thioredoxin reductase. Eur J Biochem. 2000;267(20):6102–6109.
- Kipp AP, Müller MF, Göken EM, et al. The selenoproteins GPx2, TrxR2 and TrxR3 are regulated by Wnt signalling in the intestinal epithelium. Biochim Biophys Acta Gen Subj. 2012 Oct 01;1820(10):1588–1596.
- Ding C, Fan X, Peroxiredoxin WG. 1 - an antioxidant enzyme in cancer. J Cell Mol Med. 2017;21(1):193–202.
- Mullen L, Hanschmann E-M, Lillig CH, et al. Cysteine oxidation targets peroxiredoxins 1 and 2 for exosomal release through a novel mechanism of redox-dependent secretion. Mol Med. 2015 January 01;21(1):98–108.
- Johny A, Fæste CK, Bogevik AS, et al. Development and validation of a liquid chromatography high-resolution mass spectrometry method for the simultaneous determination of mycotoxins and phytoestrogens in plant-based fish feed and exposed fish. Toxins (Basel). 2019;11(4):222.
- Geurden I, Borchert P, Balasubramanian MN, et al. The positive impact of the early-feeding of a plant-based diet on its future acceptance and utilisation in rainbow trout. PLoS One. 2013;8(12):e83162.
- Bird A. DNA methylation patterns and epigenetic memory. Genes Dev. 2002;16(1):6–21.
- Krueger F, Andrews SR. Bismark: a flexible aligner and methylation caller for Bisulfite-Seq applications. Bioinformatics. 2011;27(11):1571–1572.
- Akalin A, Kormaksson M, Li S, et al. methylKit: a comprehensive R package for the analysis of genome-wide DNA methylation profiles. Genome Biol. 2012;13(10):R87–R87.
- Heinz S, Benner C, Spann N, et al. Simple combinations of lineage-determining transcription factors prime cis-regulatory elements required for macrophage and B cell identities. Mol Cell. 2010;38(4):576–589.
- Huang DW, Sherman BT, Lempicki RA. Systematic and integrative analysis of large gene lists using DAVID bioinformatics resources. Nat Protoc. 2008 Dec 18 online;4:44.
- Huang DW, Sherman BT, Lempicki RA. Bioinformatics enrichment tools: paths toward the comprehensive functional analysis of large gene lists. Nucleic Acids Res. 2008;37(1):1–13.
- Supek F, Bošnjak M, Škunca N, et al. REVIGO summarizes and visualizes long lists of gene ontology terms. PLoS One. 2011;6(7):e21800.
- Berger MF, Levin JZ, Vijayendran K, et al. Integrative analysis of the melanoma transcriptome. Genome Res. 2010;20:413–427.