ABSTRACT
The discovery of new biomarkers would be very valuable to improve the detection of early Alzheimer’s disease (AD). DNA methylation marks may serve as epigenetic biomarkers of early AD. Here we identified epigenetic marks that are present in the human hippocampus from the earliest stages of AD. A previous methylome dataset of the human AD hippocampus was used to select a set of eight differentially methylated positions (DMPs) since early AD stages. Next, bisulphite pyrosequencing was performed in an expanded homogeneous cohort of 18 pure controls and 35 hippocampal samples with neuropathological changes of pure AD. Correlation between DNA methylation levels in DMPs and phospho-tau protein burden assessed by immunohistochemistry in the hippocampus was also determined. We found four DMPs showing higher levels of DNA methylation at early AD stages compared to controls, involving ELOVL2, GIT1/TP53I13 and the histone gene locus at chromosome 6. DNA methylation levels assessed by bisulphite pyrosequencing correlated with phospho-tau protein burden for ELOVL2 and HIST1H3E/HIST1H3 F genes. In this discovery study, a set of four epigenetic marks of early AD stages have been identified in the human hippocampus. It would be worth studying in-depth the specific pathways related to these epigenetic marks. These early alterations in DNA methylation in the AD hippocampus could be regarded as candidate biomarkers to be explored in future translational studies.
Abbreviations
AD: Alzheimer’s disease; DMPs: Differentially methylated positions; CSF: Cerebrospinal fluid; βA42: β-amyloid 42; PET: positron emission tomography; 5mC: 5-methyl cytosine; CpG: cytosine-guanine dinucleotides; ANK1: ankyrin-1; BIN1: amphiphysin II; p-tau: hyperphosphorylated tau; CERAD: Consortium to Establish A Registry for Alzheimer’s Disease; SD: standard deviation; ANOVA: one-way analysis of variance; VLCFAs: very long-chain fatty acids; DHA: docosahexaenoic acid; mTOR: mechanistic target of rapamycin.
Introduction
Alzheimer´s disease (AD) is a chronic neurodegenerative disorder with huge medical, social, and economic impacts [Citation1]. Currently, AD is the leading cause of age-related dementia. Over the last few decades, dementia prevalence has dramatically increased due to population ageing, and it is expected to affect over 152 million people worldwide by 2050 (World Alzheimer Report, 2018). Therefore, AD is becoming one of the major global challenges of our time.
Diagnostic tests and treatment options for AD are still very limited. In this scenario, a number of biomarkers have been investigated to aid diagnosis of AD. Cerebrospinal fluid (CSF) core biomarkers such as β-amyloid 42 (βA42) peptide and total/phosphorylated tau protein levels correlate well with AD brain changes [Citation2,Citation3]. Likewise, neuroimaging biomarkers such as positron emission tomography (PET) scans with tracers that bind to β-amyloid or tau protein are also promising tools for detecting AD [Citation4]. Nevertheless, there are still several roadblocks hindering the successful implementation of such biomarkers in clinical practice. For instance, lumbar puncture to obtain CSF is an invasive procedure that is often not well accepted by patients. On the other hand, PET scan is an expensive test and is not readily available in the majority of health centres. Therefore, the discovery of new biomarkers has been encouraged to increase the accuracy of clinical and pre-clinical AD diagnosis. In particular, the latter is receiving increasing attention, as the pre-clinical stage is now considered the optimal therapeutic time window in AD.
Epigenetic modifications are innovative biomarkers owing to their stability, frequency, reversibility and accessibility in body fluids, entailing great potential for assays development to assist in patient management [Citation5]. Moreover, epigenetic mechanisms are quite stable but, at the same time, can be dynamically modulated by the effect of environmental factors, such as diet and drugs. Among epigenetic mechanisms, DNA methylation is probably the one most widely studied. DNA methylation involves the attachment of a methyl group to the 5-carbon position of a cytosine residue (5mC) and usually occurs at cytosine-guanine dinucleotides (CpG). Interestingly, DNA methylation is known to be altered in the brain of AD patients. A number of genome-wide studies have revealed differentially methylated genes in AD cases compared to controls, including genes previously related to the pathogenesis or the genetic background of AD such as ANK1 (ankyrin-1) or BIN1 (amphiphysin II) [Citation6–Citation11]. All this brain-specific information may become a source of candidate epigenetic biomarkers to aid AD clinical management. In this regard, DNA methylation levels of specific genes correlate well with brain AD pathological hallmarks [Citation10] and most importantly, some of these DNA methylation marks are present in the early stages of AD, suggesting that such changes might play a role in the onset of the disease [Citation25], and may become candidate biomarkers for the early diagnosis of AD.
Our investigation focussed on the human hippocampus, a brain region most vulnerable to AD [Citation12–Citation14] and the core of pathological tau protein deposits [Citation15]. In a previous study, we profiled the methylome of the human hippocampus in AD identifying a set of differentially methylated positions (DMPs) related to the disease status [Citation16]. Here, following a translational approach, we used this methylome information along with an expanded cohort of post-mortem human hippocampal samples to identify epigenetic changes at early stages of AD. These changes in DNA methylation represent a source of new candidate epigenetic biomarkers of early AD, worth exploring in living patients.
Materials/subjects and methods
Human hippocampal samples and neuropathological examination
Brain hippocampal post-mortem samples from 53 subjects (35 AD cases and 18 controls) were provided by Navarrabiomed Brain Bank. After death, half brain specimens from donors were cryopreserved at −80°C. Neuropathological examination was completed in all cases and controls following the usual recommendations [Citation17] and according to the updated National Institute on Aging-Alzheimer’s Association guidelines [Citation18]. Assessment of β-amyloid deposition was carried out by immunohistochemical staining of paraffin-embedded sections (3–5 µm-thick) with a mouse monoclonal (S6 F/3D) anti-β-amyloid antibody (Leica Biosystems Newcastle Ltd, Newcastle upon Tyne, United Kingdom). Evaluation of neurofibrillary pathology was performed with a mouse monoclonal antibody anti-human PHF-TAU, clone AT-8 (Tau AT8) (Innogenetics, Gent, Belgium), which identifies hyperphosphorylated tau (p-tau) [Citation14]. The reaction product was visualized using an automated slide immunostainer (Leica Bond Max) with Bond Polymer Refine Detection (Leica Biosystems, Newcastle Ltd). Other protein deposits, such as synuclein deposits, were ruled out by a monoclonal antibody against α-synuclein (NCL-L-ASYN; Leica Biosystems, Wetzlar, Germany).
The staging of AD was performed by using the ABC score according to the updated National Institute on Aging-Alzheimer’s Association guidelines [Citation18]. ABC score combines histopathologic assessments of β-amyloid deposits determined by the method of Thal (A) [Citation18], staging of neurofibrillary tangles by Braak and Braak classification (B) [Citation14], and scoring of neuritic plaques by the method of CERAD (Consortium to Establish A Registry for Alzheimer’s Disease) (C) [Citation19] to characterize AD neuropathological changes. Thus, the ABC score shows three levels of AD neuropathological severity: low, intermediate, and high. For the purpose of this study, we considered early AD stage equivalent to ‘low’ ABC score. A summary of the characteristics of subjects considered in this study is shown in Supplemental Table S1.
Selection of candidate epigenetic marks in early AD
A set of differentially methylated positions (DMPs) in early stages of AD was produced from a previously generated methylome dataset [Citation16]. Full explanation of the previous methylome study is described elsewhere [Citation16]. In brief, the Infinium HumanMethylation450 BeadChip array (Illumina, Inc., San Diego, CA, USA) was performed at the Roswell Park Cancer Institute Genomics Shared Resource (Buffalo, NY, USA) to measure DNA methylation levels in CpG sites (also named positions) in a cohort of 26 pure AD cases and 12 controls. A total of 118 AD-related DMPs were identified in the hippocampus of AD cases compared to controls. For the purpose of the present study, in the set of 118 AD-related DMPs, a linear model adjusted for age and gender was fitted by using limma (RRID:SCR_010943) to determine DMPs since the early stage of AD (ABC score = low).
DNA methylation levels assessed by bisulphite pyrosequencing
Genomic DNA was isolated from frozen hippocampal tissue by phenol-chloroform method [Citation20]. Next, 500 ng of genomic DNA were bisulphite converted using the EpiTect Bisulfite Kit (Qiagen, Redwood City, CA, USA) according to the manufacturer’s protocol. Primer pairs to amplify and sequence the chosen CpG genomic positions were designed with PyroMark Assay Design version 2.0.1.15 (Qiagen) (Supplemental Table S2), and bisulphite PCR reactions were carried out on a VeritiTM Thermal Cycler (Applied Biosystems, Foster City, CA, USA). Next, 20 μl of biotinylated PCR product was immobilized using streptavidin-coated sepharose beads (GE Healthcare Life Sciences, Piscataway, NJ, USA) and 0.4 μM sequencing primer was annealed to purified DNA strands. Pyrosequencing was performed using the PyroMark Gold Q96 reagents (Qiagen) on a PyroMark™ Q96 ID System (Qiagen). For each particular CpG, methylation levels were expressed as percentage of methylated cytosines over the sum of total cytosines. Unmethylated and methylated DNA samples (EpiTect PCR Control DNA Set, Qiagen) were used as controls for the pyrosequencing reaction.
Quantitative assessment of p-tau deposits in hippocampal samples
To quantitatively assess p-tau burden in the hippocampal samples of AD subjects we applied a method described in detail elsewhere [Citation21]. In brief, sections of the hippocampus were examined after performing immunostaining with anti-p-tau antibody (clone AT-8) (dilution 1/1000), and representative images were analysed with ImageJ software to obtain an average quantitative measure of the global p-tau deposit for each section and patient.
Statistical data analysis
Statistical analysis was performed with SPSS 21.0 (IBM, Inc., USA). First, adjustment to normal distribution was tested for all continuous variables as per one-sample Kolgomorov-Smirnov test and the normal quantil-quantil (QQ) plots. Data represent the mean ± standard deviation (SD) or the median (interquartile range). Pyrosequencing intergroup differences were tested by one-way analysis of variance (ANOVA) or Kruskal Wallis test. Post-hoc Scheffe or Games-Howell tests were used to identify which pairs of groups showed a statistically significant difference when ANOVA was performed. Pearson or Spearman correlation coefficient was used to determine correlation between microarray and pyrosequencing DNA methylation levels. Spearman correlation coefficient was used to correlate tau burden and DNA methylation levels for each DMPs. For all the comparisons, significance level was set at p-value < 0.05. GraphPad Prism version 6.00 for Windows (GraphPad Software, La Jolla, CA, USA) was used to draw graphs.
Ethics, consent, and permissions
The Ethics Committee of the ‘Complejo Hospitalario de Navarra’ approved the use of human subjects for this study (Pyto 90/2014). Written informed consent was obtained from all subjects or next of kin.
Results
Screening for candidate epigenetic marks at early AD stages
In order to select a set of candidate epigenetic marks, we used previously generated DNA methylation microarray data [Citation16] to identify CpG sites showing differential DNA methylation at early AD stages. Infinium HumanMethylation450 BeadChip array had been performed in a set of 38 post-mortem hippocampal samples (26 pure AD patients and 12 controls). In that study, stringent criteria were applied to select pure AD cases and controls preventing the recruitment of samples with multi-protein deposits. This approach maximizes the chances of finding true molecular associations with AD, even though reducing the number of samples. Characteristics of samples and microarray analysis methods were fully described elsewhere [Citation16]. A total of 118 differentially methylated positions (DMPs) were found in the hippocampus of pure AD cases compared to controls. Within this set of 118 DMPs, we fitted a linear model adjusted for age and gender to identify those DMPs that were differentially methylated since the early stage of AD (ABC score = low). Thus, we found eight DMPs in the early stage of AD (ABC score = low) compared to controls (absolute β-difference ≥ 0.085 and p-value ≤ 0.05) (; Supplemental Figure S1).
Table 1. Differentially methylated positions (DMPs) in the hippocampus of early-stage AD in the 450 K microarray.
Validation of candidate epigenetic marks at early AD stages
An expanded cohort of 53 post-mortem human hippocampal samples (18 controls, 12 low ABC score, 14 intermediate ABC score, and 9 high ABC score), which included all the samples analysed in the methylome study [Citation16], was used to validate the candidate epigenetic marks (; Supplemental Table S1). DNA methylation levels at the eight DMPs were quantified by bisulphite pyrosequencing. Significant positive correlation was observed in DNA methylation levels between 450 k microarray results and pyrosequencing measurements for the samples included in the 450 K microarray (n = 38) (Supplemental Table S3 and Supplemental Figure S2).
In the validation stage, a total of four CpG sites (DMPs) showed significant differences in DNA methylation levels in early-stage AD cases (n = 12) compared to controls (n = 18) (). DNA methylation levels were significantly increased in early AD (ABC score = low) samples compared to controls for CpG sites associated with ELOVL2 (28.9 ± 0.7% vs. 23 ± 1.4%, p-value < 0.01), GIT1/TP53I13 (5.4 ± 0.9% vs. 1.8 ± 0.6%, p-value < 0.01), HIST1H1A (29.5 ± 2.1% vs. 22.4 ± 2.9%, p-value < 0.05) and HIST1H3E/HIST1H3 F (11.2 ± 1.5% vs. 8.1 ± 0.4%, p-value < 0.01) genes. Interestingly, DNA methylation levels at these four genomic positions remained significantly elevated across all AD stages compared to controls ().
Figure 1. Early epigenetic marks of AD validated by bisulphite pyrosequencing results. DNA methylation levels in AD stages vs. control samples at validated CpG sites ELOVL2-cg16867657 (a), GIT1/TP53I13-cg05877788 (b), HIST1H1A-cg07816556 (c) and HIST1H3E/HIST1H3 F- cg13836098 (d). *p-value<0.05; **p-value<0.01; ***p-value <0.001, ****p-value <0.0001. Grey dots: outlier samples.
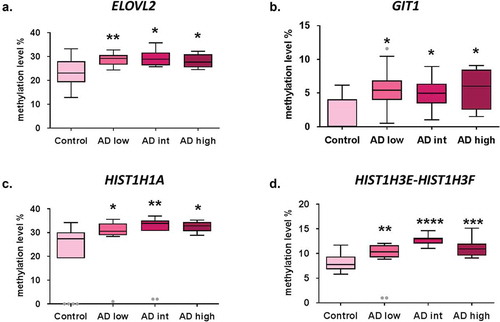
Correlation between validated epigenetic marks and p-tau deposits
Since hyperphosphorylated tau (p-tau) protein deposits occur in the hippocampus at the very initial stages of AD, we aimed to correlate DNA methylation levels at each of the four validated CpG sites and p-tau protein burden in the hippocampal samples. p-tau burden was measured and averaged for each subject by a semi-automated quantitative method by using the ImageJ software (see Methods section). Following this approach, we found that the average area of p-tau protein burden in the hippocampus was positively correlated with ELOVL2 gene methylation levels [r = 0.350; p-value < 0.05] and HIST1H3E/HIST1H3 F gene methylation levels [r = 0.469; p-value < 0.01] ( and ).
Table 2. Correlation between DNA methylation levels at each validated DMP and tau burden.
Figure 2. Scatter plots graphs of correlation analysis result for DNA methylation levels at each validated DMP and tau burden. Correlation between validated epigenetic marks and p-tau protein burden is graphically showed for ELOVL2 (a), GIT1/TP53I13 (b), HIST1H1A (c) and HIST1H3E/HIST1H3 F (d) genes. A significant positive correlation was found for ELOVL2 [r = 0.350; p-value < 0.05] and HIST1H3E/HIST1H3 F [r = 0.469; p-value < 0.01] genes.
![Figure 2. Scatter plots graphs of correlation analysis result for DNA methylation levels at each validated DMP and tau burden. Correlation between validated epigenetic marks and p-tau protein burden is graphically showed for ELOVL2 (a), GIT1/TP53I13 (b), HIST1H1A (c) and HIST1H3E/HIST1H3 F (d) genes. A significant positive correlation was found for ELOVL2 [r = 0.350; p-value < 0.05] and HIST1H3E/HIST1H3 F [r = 0.469; p-value < 0.01] genes.](/cms/asset/117f60fe-a503-4032-ab8e-5ea2ec2f4ef5/kepi_a_1748917_f0002_b.gif)
Discussion
Here we report increased levels of DNA methylation at four distinct genomic positions in the human hippocampus from the earliest stage of AD, involving ELOVL2, GIT1/TP53I13 and the histone gene cluster. Interestingly, ELOVL2 and HIST1H3E/HIST1H3 F methylation levels positively correlated with deposits of p-tau protein in the hippocampus samples. These epigenetic marks may represent early alterations in DNA methylation in the AD hippocampus and could be regarded as candidate biomarkers to be explored in future translational studies. As only pure AD cases, with no other protein deposits, were considered for the present study, the chance of finding epigenetic disturbances truly related with AD molecular pathways is increased.
A CpG site located within a CpG island in the promoter region of ELOVL2 gene showed an increase in DNA methylation in early-stage AD cases compared to controls. This gene encodes an endoplasmic reticulum-bound enzyme that participates specifically in the production of polyunsaturated very long-chain fatty acids (VLCFAs) [Citation22,Citation23]. VLCFAs play important roles in membrane stabilization, cell signalling, and modulation of inflammatory responses [Citation24]. Interestingly, VLCFAs accumulate in cortical brain regions at advanced AD stages [Citation25] which may contribute to neuron dysfunction by impairment of mitochondrial functions [Citation26]. Moreover, the ablation of Elovl2 in mice has been proved detrimental for the systemic synthesis of an omega-3 fatty acid, the docosahexaenoic acid (DHA), [Citation27], which is being considered a promising treatment for AD [Citation28].
Even though no monogenic disease has been related with ELOVL2 so far, mutations involving another fatty acid elongase enzyme have been reported to cause disease with important brain involvement. That is the case of ELOVL4, which has been identified to cause autosomal dominant spinocerebellar ataxia with erythrokeratodermia [Citation29] and mental retardation, seizures and quadriplejia [Citation24], pointing to a crucial role for long-chain fatty acids biosynthesis in neuronal functioning and development. On the other hand, ELOLV2 gene may play a central role in the innate immune defence system, particularly in the macrophage function. It has been observed that macrophages derived from Elovl2-/- mice display an alteration in M1/M2 polarization with M1 being hyperactive and more pro-inflammatory while M2 less protective [Citation30]. The latter report supports the notion that ELOVL2 may be crucial in inflammatory processes and macrophage polarization, and this association is remarkable since the idea that microglia plays a fundamental role in the development of AD is gaining strength in recent years.
It is also known that ELOVL2 is epigenetically regulated in glioma stem cells and participates in glioblastoma cell growth and tumour initiation [Citation31]. In addition, ELOVL2 methylation levels have been previously related to chronological age prediction across different tissues [Citation32,Citation33]. In the present study, ELOVL2 methylation levels were directly correlated with p-tau protein deposits in the human hippocampus suggesting that this gene might be involved in the initiation and progression of AD.
A second differentially methylated CpG was found within a CpG island at the 3ʹ end of GIT1 and TP53I13 genes. GIT1 gene is highly expressed in the brain compared with other human tissues while TP53I13 displays the lowest expression levels in the brain [Citation34]. GIT1 encodes a signalling scaffold protein that participates in the regulation of cytokinesis by increasing the speed of cell migration and the formation of protrusions [Citation35]. It has also been implicated in angiogenesis [Citation36] and cerebral vascular stability [Citation37,Citation38]. GIT1 has been found to be overexpressed in macrophages from unstable carotid plaques [Citation39] and participates in migration of endothelial and vascular smooth muscle cell in atherosclerotic plaques [Citation40].
Most interestingly, the protein encoded by GIT1 seems to exert relevant effects in the brain, particularly in synapsis formation and dendritic spine pruning in hippocampal neurons [Citation41–Citation46]. Thus, Git1-knockout mice develop severe learning and memory defects and reduced synaptic plasticity [Citation47]. Importantly, GIT1 seems a driver of interneuron and hippocampal neurite differentiation in vitro [Citation48], probably through accelerating microtubule assembly [Citation49]. Its relevance is further supported by the finding of loss-of-function rare variants in GIT1 in schizophrenia, a brain disorder that involves significant synaptic dysfunction [Citation50]. Another interesting issue is that GIT1 binds to the mechanistic target of rapamycin (mTOR) protein to promote astrocyte survival [Citation51].
The most statistically consistent results were found at two CpG sites located within the histone gene cluster in the short arm of chromosome 6 (6p21-p22). The first CpG site was at the 3ʹ end of HIST1H1A gene, separated from HIST1H4A/HIST1H3A promoter by only 3500 bp. The second CpG site was located in a CpG island shore in the promoter of HIST1H3E/HIST1H3 F genes. Histones are proteins responsible for DNA wrapping in the nucleosome structure of chromosomes. Apart from structural function, histones also play a relevant role in gene transcription, DNA repair and chromosome stabilization. HIST1H1A encodes a histone H1 protein (H1.1) which binds to DNA linker between nucleosomes and is necessary for condensation and organization of chromatin [Citation52]. The other genes encode three of the six subunits that constitute histone H3, which is a core component of the nucleosome structure [Citation53].
The occurrence of epigenetic alterations in the histone gene cluster at early stages of AD is intriguing. Interestingly, histone H1 stimulates DNA cleavage during the apoptotic process [Citation54] and is lost in cellular senescence [Citation55]. It is also known that HIST1H1A modulates the susceptibility to aggressive prostate cancer, probably by influencing Wnt pathway genes [Citation56] and has been related to breast cancer [Citation57] and acute myeloid leukaemia [Citation58]. However, no previous relationship of HIST1H1A with AD or neurodegeneration has been described so far. On the contrary, HIST1H3E mRNA transcript has been found to be differentially expressed in peripheral blood samples of Parkinson disease patients compared to controls [Citation59] in a pilot study. Moreover, using a methyl-DNA immunoprecipitation approach, researchers founded hypermethylation in the promoter region of HIST1H3E gene in glioma versus normal brain [Citation60]. Hypermethylation of HIST1H3E has been also found in lung adenocarcinoma in a genome-wide methylation study [Citation61].
A limitation of the current study is that causal or mechanistic conclusions cannot be drawn since this is an observational, cross-sectional study. While the findings of cross-sectional studies should be interpreted carefully, new interesting hypothesis may emerge from our results. We observed that these four validated genomic positions increased their methylation levels from the very early stages of the disease and were differentially methylated across all stages of AD. Therefore, it would be worthy to further investigate if they may have a causal role in AD pathology by designing additional experimental studies. Particularly, for those mark that were significantly and positively correlated with the burden of p-tau protein in the hippocampus, i.e., ELOVL2 and HIST1H3E genes. Furthermore, this is a study in the discovery phase in brain tissue, so the results are not directly applicable to clinical practice.
Conclusions
To sum up, in this discovery study a set of four epigenetic marks of early AD stages have been identified and validated in the human hippocampus. Early epigenetic changes in DNA methylation may contribute to support or discover pathways involved in AD pathogenesis, such as the long-chain fatty acid biogenesis or histone functions. With a translational approach, it is worth considering them as candidate biomarkers of early-stage AD. The next step would be to measure DNA methylation levels of these candidate genes in a more accessible biological fluid, such as blood or saliva, and validate results in independent and larger cohorts. Early detection of AD would give patients a better chance to benefit from clinical trials and prompt interventions and nowadays the preclinical stage of the disease is considered the optimal time window to test disease-modifying drugs. Therefore, defining early-stage AD biomarkers is a critical need in fighting neurodegeneration.
Authors’ contributions
IBL contributed to data collection, analysis of data, statistical analysis, and drafting/revising the manuscript for content. BA contributed to running experiments, acquisition, and interpretation of data and revising the manuscript for content. AUC contributed to data analysis, figure drawing, and drafting/revising the manuscript for content. JSR contributed to analysis and interpretation of data (p-tau deposits), sorting of patients into different ABC stages and acquisition of image data. JV contributed to acquisition of data, figure drawing and revising the manuscript for content. MRA contributed to drawing figures, performed bisulfite pyrosequencing experiments, and was involved in interpretation of data. AL contributed to bioinformatics analysis and drafting the manuscript. MVZ participated in revising subject diagnosis, classification of patients, and contributed to drafting/revising the manuscript for content. CC contributed to data collection, diagnosis of subjects and revising the manuscript for content. IM contributed to study concept and design, literature searching, and drafting/revising the manuscript for content. MM contributed to study concept and design, data collection, interpretation and analysis, statistical analysis, drafting/revising the manuscript for content, study supervision, and obtaining funding.
Ethics approval and consent to participate
This study was carried out in accordance with the principles of the Declaration of Helsinki and handling of human brain samples was performed according to the current Spanish national legislation (Royal Decree RD1716/2011). Written informed consent was obtained from all subjects or next of kin. The study was approved by the Ethics Committee of the Complejo Hospitalario de Navarra and the Scientific Committee of Navarrabiomed Brain Bank, Spain.
Supplemental Material
Download PDF (1.6 MB)Acknowledgments
We want to kindly thank Teresa Tuñón M.D., Ph.D (Department of Pathology, Complejo Hospitalario de Navarra, technical support), Federico García-Bragado M.D., Ph.D (Department of Pathology, Complejo Hospitalario de Navarra, technical support), and Isabel Gil M.D., Ph.D (Navarrabiomed BrainBank, technical support) for their help. Finally, we are very grateful to the patients and relatives that generously donor the brain tissue to the Navarrabiomed Brain Bank.
Disclosure statement
The authors report no conflict of interest.
Supplementary material
Supplemental data for this article can be accessed here.
Additional information
Funding
References
- Wimo A, Jonsson L, Bond J, et al. The worldwide economic impact of dementia 2010. Alzheimer’s Dementia 2013; 9(1): 1–11 e13.
- Molinuevo JL, Gispert JD, Dubois B, et al. The AD-CSF-index discriminates Alzheimer’s disease patients from healthy controls: a validation study. J Alzheimers Dis. 2013;36(1):67–77. .
- McKhann GM, Knopman DS, Chertkow H, et al. The diagnosis of dementia due to Alzheimer’s disease: recommendations from the National Institute on Aging-Alzheimer’s Association workgroups on diagnostic guidelines for Alzheimer’s disease. Alzheimer’s Dementia. 2011;7(3):263–269.
- Arbizu J, Garcia-Ribas G, Carrio I, et al. Recommendations for the use of PET imaging biomarkers in the diagnosis of neurodegenerative conditions associated with dementia: SEMNIM and SEN consensus. Revista espanola de medicina nuclear e imagen Molecular. 2015;34(5):303–313.
- Costa-Pinheiro P, Montezuma D, Henrique R, et al. Diagnostic and prognostic epigenetic biomarkers in cancer. Epigenomics. 2015;7(6):1003–1015.
- Bakulski KM, Dolinoy DC, Sartor MA, et al. Genome-wide DNA methylation differences between late-onset Alzheimer’s disease and cognitively normal controls in human frontal cortex. J Alzheimers Dis. 2012;29(3):571–588.
- Sanchez-Mut JV, Aso E, Heyn H, et al. Promoter hypermethylation of the phosphatase DUSP22 mediates PKA-dependent TAU phosphorylation and CREB activation in Alzheimer’s disease. Hippocampus. 2014;24(4):363–368.
- Lunnon K, Smith R, Hannon E, et al. Methylomic profiling implicates cortical deregulation of ANK1 in Alzheimer’s disease. Nat Neurosci. 2014;17(9):1164–1170.
- De Jager PL, Srivastava G, Lunnon K, et al. Alzheimer’s disease: early alterations in brain DNA methylation at ANK1, BIN1, RHBDF2 and other loci. Nat Neurosci. 2014;17(9):1156–1163.
- Yu L, Chibnik LB, Srivastava GP, et al. Association of Brain DNA methylation in SORL1, ABCA7, HLA-DRB5, SLC24A4, and BIN1 with pathological diagnosis of Alzheimer disease. JAMA Neurol. 2015;72(1):15–24. .
- Watson CT, Roussos P, Garg P, et al. Genome-wide DNA methylation profiling in the superior temporal gyrus reveals epigenetic signatures associated with Alzheimer’s disease. Genome Med. 2016;8(1):5.
- Wenk GL. Neuropathologic changes in Alzheimer’s disease. J Clin Psychiatry. 2003;64(Suppl 9):7–10.
- Braak H, Braak E. Neuropathological stageing of Alzheimer-related changes. Acta Neuropathol. 1991;82(4):239–259.
- Braak H, Alafuzoff I, Arzberger T, et al. Staging of Alzheimer disease-associated neurofibrillary pathology using paraffin sections and immunocytochemistry. Acta Neuropathol. 2006;112(4):389–404.
- Lace G, Savva GM, Forster G, et al. Hippocampal tau pathology is related to neuroanatomical connections: an ageing population-based study. Brain. 2009;132(Pt 5):1324–1334.
- Altuna M, Urdánoz-Casado A, Sánchez-ruiz de Gordoa J, et al. DNA methylation signature of human hippocampus in Alzheimer’s disease is linked to neurogenesis. Clin Epigenetics. 2019;11(1):91.
- Bell JE, Alafuzoff I, Al-Sarraj S, et al. Management of a twenty-first century brain bank: experience in the BrainNet Europe consortium. Acta Neuropathol. 2008;115(5):497–507.
- Montine TJ, Phelps CH, Beach TG, et al. National Institute on Aging-Alzheimer’s Association guidelines for the neuropathologic assessment of Alzheimer’s disease: a practical approach. Acta Neuropathol. 2012;123(1):1–11.
- Mirra SS, Heyman A, McKeel D, et al. The consortium to establish a registry for Alzheimer’s Disease (CERAD). Part II. Standardization of the neuropathologic assessment of Alzheimer’s disease. Neurology. 1991;41(4):479–486.
- Miller SA, Dykes DD, Polesky HF. A simple salting out procedure for extracting DNA from human nucleated cells. Nucleic Acids Res. 1988;16(3):1215.
- Celarain N, Sanchez-ruiz de Gordoa J, Zelaya MV, et al. TREM2 upregulation correlates with 5-hydroxymethycytosine enrichment in Alzheimer’s disease hippocampus. Clin Epigenetics. 2016;8:37.
- de Antueno RJ, Knickle LC, Smith H, et al. Activity of human Delta5 and Delta6 desaturases on multiple n-3 and n-6 polyunsaturated fatty acids. FEBS Lett. 2001;509(1):77–80.
- Leonard AE, Kelder B, Bobik EG, et al. Identification and expression of mammalian long-chain PUFA elongation enzymes. Lipids. 2002;37(8):733–740.
- Aldahmesh MA, Mohamed JY, Alkuraya HS, et al. Recessive mutations in ELOVL4 cause ichthyosis, intellectual disability, and spastic quadriplegia. Am J Hum Genet. 2011;89(6):745–750.
- Kou J, Kovacs GG, Höftberger R, et al. Peroxisomal alterations in Alzheimer’s disease. Acta Neuropathol. 2011;122(3):271–283.
- Zarrouk A, Vejux A, Nury T, et al. Induction of mitochondrial changes associated with oxidative stress on very long chain fatty acids (C22:0, C24:0, or C26:0)-treated human neuronal cells (SK-NB-E). Oxid Med Cell Longev. 2012;2012:623257.
- Pauter AM, Olsson P, Asadi A, et al. Elovl2 ablation demonstrates that systemic DHA is endogenously produced and is essential for lipid homeostasis in mice. J Lipid Res. 2014;55(4):718–728.
- Thomas J, Thomas CJ, Radcliffe J, et al. Omega-3 fatty acids in early prevention of inflammatory neurodegenerative disease: a focus on Alzheimer’s disease. Biomed Res Int. 2015;2015:172801.
- Cadieux-Dion M, Turcotte-Gauthier M, Noreau A, et al. Expanding the clinical phenotype associated with ELOVL4 mutation: study of a large French-Canadian family with autosomal dominant spinocerebellar ataxia and erythrokeratodermia. JAMA Neurol. 2014;71(4):470–475.
- Talamonti E, Pauter AM, Asadi A, et al. Impairment of systemic DHA synthesis affects macrophage plasticity and polarization: implications for DHA supplementation during inflammation. Cell Mol Life Sci. 2017;74(15):2815–2826.
- Gimple RC, Kidwell RL, Kim LJY, et al. Glioma stem cell specific super enhancer promotes polyunsaturated fatty acid synthesis to support EGFR Signaling. Cancer Discov. 2019;9(9):1248–1267.
- Slieker RC, Relton CL, Gaunt TR, et al. Age-related DNA methylation changes are tissue-specific with ELOVL2 promoter methylation as exception. Epigenetics Chromatin. 2018;11(1):25.
- Garagnani P, Bacalini MG, Pirazzini C, et al. Methylation of ELOVL2 gene as a new epigenetic marker of age. Aging Cell. 2012;11(6):1132–1134.
- Melé M, Ferreira PG, Reverter F, et al. Human genomics. The human transcriptome across tissues and individuals. Science. 2015;348(6235):660–665.
- Manabe R, Kovalenko M, Webb DJ, et al. GIT1 functions in a motile, multi-molecular signaling complex that regulates protrusive activity and cell migration. J Cell Sci. 2002;115(Pt 7):1497–1510.
- Pang J, Hoefen R, Pryhuber GS, et al. G-protein-coupled receptor kinase interacting protein-1 is required for pulmonary vascular development. Circulation. 2009;119(11):1524–1532.
- Jones CA, Nishiya N, London NR, et al. Slit2-Robo4 signalling promotes vascular stability by blocking Arf6 activity. Nat Cell Biol. 2009;11(11):1325–1331.
- Liu J, Zeng L, Kennedy RM, et al. βPix plays a dual role in cerebral vascular stability and angiogenesis, and interacts with integrin αvβ8. Dev Biol. 2012;363(1):95–105.
- Slevin M, Elasbali AB, Miguel Turu M, et al. Identification of differential protein expression associated with development of unstable human carotid plaques. Am J Pathol. 2006;168(3):1004–1021.
- Wang J, Taba Y, Pang J, et al. GIT1 mediates VEGF-induced podosome formation in endothelial cells: critical role for PLCgamma. Arterioscler Thromb Vasc Biol. 2009;29(2):202–208.
- Kehoe LA, Bellone C, De Roo M, et al. GluN3A promotes dendritic spine pruning and destabilization during postnatal development. J Neurosci. 2014;34(28):9213–9221.
- Hong ST, Mah W. A critical role of GIT1 in vertebrate and invertebrate brain development. Exp Neurobiol. 2015;24(1):8–16.
- Smith KR, Davenport EC, Wei J, et al. GIT1 and βPIX are essential for GABA A receptor synaptic stability and inhibitory neurotransmission. Cell Rep. 2014;9(1):298–310.
- Lim J, Ritt DA, Zhou M, et al. The CNK2 scaffold interacts with vilse and modulates Rac cycling during spine morphogenesis in hippocampal neurons. Curr Biol. 2014;24(7):786–792.
- Menon P, Deane R, Sagare A, et al. Impaired spine formation and learning in GPCR kinase 2 interacting protein-1 (GIT1) knockout mice. Brain Res. 2010;1317:218–226.
- Zhang H, Webb DJ, Asmussen H, et al. Synapse formation is regulated by the signaling adaptor GIT1. J Cell Biol. 2003;161(1):131–142.
- Martyn AC, Toth K, Schmalzigaug R, et al. GIT1 regulates synaptic structural plasticity underlying learning. PLoS One. 2018;13(3):e0194350.
- Franchi SA, Astro V, Macco R, et al. Identification of a protein network driving neuritogenesis of MGE-derived GABAergic interneurons. Front Cell Neurosci. 2016;10:289.
- Li YS, Qin LX, Liu J, et al. GIT1 enhances neurite outgrowth by stimulating microtubule assembly. Neural Regen Res. 2016;11(3):427–434.
- Kim MJ, Biag J, Fass DM, et al. Functional analysis of rare variants found in schizophrenia implicates a critical role for GIT1-PAK3 signaling in neuroplasticity. Mol Psychiatry. 2017;22(3):417–429.
- Smithson LJ, Gutmann DH. Proteomic analysis reveals GIT1 as a novel mTOR complex component critical for mediating astrocyte survival. Genes Dev. 2016;30(12):1383–1388.
- Th’ng JP, Sung R, Ye M, et al. H1 family histones in the nucleus. Control of binding and localization by the C-terminal domain. J Biol Chem. 2005;280(30):27809–27814.
- Albig W, Kardalinou E, Drabent B, et al. Isolation and characterization of two human H1 histone genes within clusters of core histone genes. Genomics. 1991;10(4):940–948.
- Widlak P, Kalinowska M, Parseghian MH, et al. The histone H1 C-terminal domain binds to the apoptotic nuclease, DNA fragmentation factor (DFF40/CAD) and stimulates DNA cleavage. Biochemistry. 2005;44(21):7871–7878.
- Funayama R, Saito M, Tanobe H, et al. Loss of linker histone H1 in cellular senescence. J Cell Biol. 2006;175(6):869–880.
- Williams KA, Lee M, Winter JM, et al. Prostate cancer susceptibility gene. Oncotarget. 2018;9(47):28532–28546.
- Harshman SW, Hoover ME, Huang C, et al. Histone H1 phosphorylation in breast cancer. J Proteome Res. 2014;13(5):2453–2467.
- Tiberi G, Pekowska A, Oudin C, et al. PcG methylation of the HIST1 cluster defines an epigenetic marker of acute myeloid leukemia. Leukemia. 2015;29(5):1202–1206.
- Grünblatt E, Zehetmayer S, Jacob CP, et al. Pilot study: peripheral biomarkers for diagnosing sporadic Parkinson’s disease. J Neural Transm (Vienna). 2010;117(12):1387–1393.
- Zhang Z, Tang H, Wang Z, et al. MiR-185 targets the DNA methyltransferases 1 and regulates global DNA methylation in human glioma. Mol Cancer. 2011;10:124.
- Daugaard I, Dominguez D, Kjeldsen TE, et al. Identification and validation of candidate epigenetic biomarkers in lung adenocarcinoma. Sci Rep. 2016;6:35807.