ABSTRACT
N6-methyladenosine (m6A), the most prevalent RNA internal modification, is present in most eukaryotic species and prokaryotes. Studies have highlighted an intricate network architecture by which m6A epitranscriptome impacts on immune response and function. However, it was only until recently that the mechanisms underlying the involvement of m6A modification in immune system were uncovered. Here, we systematically review the m6A involvement in the regulation of innate and adaptive immune cells. Further, the interplay between m6A modification and anti-inflammatory, anti-viral and anti-tumour immunity is also comprehensively summarized. Finally, we focus on the future prospects of m6A modification in immune modulation. A better understanding of the crosstalk between m6A modification and immune system is of great significance to reveal new pathogenic pathways and to develop promising therapeutic targets of diseases.
Introduction
Diverse chemical modifications have been identified in the transcriptome, leading to the emerging field of epitranscriptomics [Citation1]. N6-methyladenosine (m6A) is the most prevalent internal modification in mRNA [Citation2]. Early in the 1970s, m6A was detected in poly(A)+RNA [Citation2]. The advent of transcriptome-wide profiling technologies has rendered possibilities of identifying the distribution of m6A within the R(m6A)CH consensus motif (where R = G or A; H = A, C, or U) [Citation3–5].
Generally, m6A modifications occur via the m6A methyltransferases called ‘writers’; they are removed by the demethylases called ‘erasers’ and are recognized by m6A-binding proteins called ‘readers’ [Citation6]. Studies have highlighted that m6A modification plays an indispensable role in maintaining the naive and pluripotent status of immune cells within immune regulatory circuits [Citation7–9]. The emerging concepts for m6A epitranscriptome have been described in many aspects of the immune system [Citation10,Citation11]. Above all, our study provides an in-depth summary toward better understanding the m6A involvement in immune modulation and aims to inspire the identification of new pathogenic basis and effective therapeutics for m6A-related diseases.
Dynamic transcriptomic M6A decoration
The unravelling of m6A landscape has greatly facilitated the understanding of m6A machinery in eukaryotes and virus [Citation6,Citation12]. The impact of m6A on RNA was markedly linked to the dynamic crosstalk with m6A writers, erasers, and readers [Citation13]. The knowledge of m6A machinery regarding m6A writers, erasers, and readers was summarized here to facilitate the comprehensive understanding. It is also helpful for readers to refer to other reviews concentrating on this particular subject [Citation14–16].
M6A writers
The instalment of m6A is critically induced by m6A writers, including Methyltransferase Like 3 (METTL3), METTL14, METTL16, Wilms Tumor 1 Associated Protein (WTAP), RNA-Binding Motif Protein 15 (RBM15), and zinc finger CCCH domain-containing protein 13 (ZC3H13) [Citation17–21]. METTL3, the first identified m6A methyltransferase in eukaryotes, greatly prompted the identification of the machinery of m6A writers [Citation18]. The previously uncharacterized METTL14 was elucidated to be tightly correlated with METTL3 and harboured an MTA domain [Citation18]. METTL3 and METTL14 were testified to function synergistically to elicit stronger effects on in-vitro methyltransferase activities compared with the role of the individual gene [Citation13,Citation22]. This perspective was further confirmed by many other studies [Citation23,Citation24]. However, there were apparent disputes over the respective impact induced by each of these two methyltransferases, which required to be further explored.
Emerging studies attach importance on the structural analysis of m6A writer complex and illuminate the crystal structure of a METTL3/METTL14 heterodimer [Citation12,Citation24]. It was elucidated that METTL14 possessed a degenerate active site and took an non-catalytic function in keeping complex integrity and substrate RNA binding [Citation12,Citation18]. Meanwhile, METTL3 generally acted to serve as the catalytic core. The results from other previously published studies showed that METTL14 exerted greater methyltransferase activity compared with the activity induced by METTL3, suggesting the role of METTL14 as the prominent catalytic subunit [Citation12,Citation18]. The conflicts within the activity of METTL3/METTL14 in methylation assays can be partly attributed to the contamination of METTL3. However, comprehensive detecting of METTL3/METTL14 complex structure may give new insights into the specificity of the substrate sequence.
It is notable that m6A deposition in transcripts is also critically mediated through interaction with a universally expressed nuclear protein, WTAP. Ping et al. revealed that WTAP impacted no effects on METTL3-METTL14 activities in vitro, resulting from the lack of methyltransferase domains in WTAP [Citation13,Citation19]. Instead, WTAP took a crucial part in assisting METTL3 and METTL14 to be localized in nuclear spots [Citation19]. The bridging between WTAP and mRNA-binding factor Nito, which was induced by ZC3H13, was determined to strikingly elevate m6A [Citation21]. In addition, RBM15, another m6A writer, could bind with METTL13 in a WTAP-dependent manner [Citation21]. RBM15 was clarified to preferentially interact with U-rich sequences proximal m6 A sites [Citation21]. As for METTL16, a newly-discovered methyltransferase, it evolved an extra role in regulating S-adenosylmethionine (SAM) homeostasis and regulated the expression level of human methionine adenosyltransferase 2A (MAT2A) [Citation17]. Furthermore, METTL16 was also involved in the regulation of METTL3/METTL14-induced activities [Citation17]. Specifically, the elevation of METTL16 occupancy on the MAT2A 3ʹ-UTR was determined to mediate effective splicing [Citation25].
M6A erasers
Currently, the involvement of m6A erasers is of great significance to ensure the equilibrium of m6 A modification in the transcriptome [Citation6]. Fat mass and obesity-associated protein (FTO) was initially elucidated in the regulation of overweight and obesity and determined to be the first enzyme-linked to m6A demethylation [Citation26,Citation27]. The C-terminal domain of FTO included a specific fold and participated in the selection of substrate [Citation27]. Currently, researchers have proposed that FTO deficiency could contribute to the enhancement of m6A peaks [Citation28]. By contrast, FTO elevation resulted in an accelerated removal of global m6A modification in acute myelocytic leukaemia (AML) [Citation28,Citation29]. It is interesting to find that FTO could block YTHDF2-induced mRNA degradation via suppressing m6A levels of cyclin A2 and cyclin-dependent kinase 2 (CDK2), leading to fat cell cycle progression and adipogenesis [Citation30]. Similarly, FTO knockdown significantly enhanced m6A methylation and dramatically suppressed the expression of melanoma cell-intrinsic genes PD-1, CXCR4, and SOX10 partially via YTHDF2-induced mRNA decay [Citation31].
As for alkb homolog 5 (ALKBH5), it was determined to mediate the greater involvement in m6A demethylation compared with FTO [Citation32]. Evidence demonstrated that ALKBH5 was localized in the nucleus and impacted fundamental roles in various physiological and pathological conditions through an m6A modification-dependent mode [Citation32]. Zheng et al. revealed that RNA helicase DEAD-box 46 (DDX46) suppressed antiviral innate responses through specific recruitment of m6A demethylase ALKBH5, with inhibition of interferon production [Citation7].
M6A readers
It is highlighted that m6A is involved in the interplay between RNA and proteins and functions as a key element for mediating responses upon various external stimuli [Citation33]. The action mechanism of m6A was further elucidated through identification of m6A-binding proteins [Citation33]. In YT521B homology domain family (YTHDF), the YTH domain displayed conserved m6A-binding domain and preferentially bound to m6A-modified RNA in RRm6ACH consensus sequence [Citation33].
In the cytoplasm, YTHDF1 was found to interact with initiation factors to actively promote mRNA translation and protein synthesis, guaranteeing the active protein production [Citation34,Citation35]. Wang and his colleagues uncovered that YTHDF2 could decrease the stability of m6A-modified mRNA [Citation34]. The destinies of these transcripts were partially under the control of YTHDF2-mediated degradation. It is notable that YTHDF1-3 proteins function synergistically to impact on RNA metabolism [Citation35]. For instance, YTHDF3 promoted the initiation of translation with the assistance of YTHDF1 and eukaryotic initiation factor 4A3 (eIF4A3) [Citation35]. Meanwhile, YTHDF3 cooperated with YTHDF2 to induce mRNA degradation [Citation35]. Liu et al. showed that YTHDF3/METTL3 complex could markedly elevate the stability of long non-coding RNAs (lncRNAs), such as metastasis-associated lung adenocarcinoma transcript 1 (MALAT1) [Citation36].
In the nucleus, YTH domain-containing 1 (YTHDC1) was known as an m6A writer and impacted on RNA function and metabolism [Citation37]. Current studies have demonstrated that the knockout of YTHDC1/METTL3 in embryonic stem cells elevated chromatin accessibility and promoted transcription in an m6A-dependent manner [Citation38]. Moreover, the depletion of YTHDC1 and METTL3 prompted the global level of two active marks of histone, including H3K4me3 and H3K27ac [Citation39]. Notably, Roundtree et al. provided a new action of the mechanism of YTHDC1 in transferring methylated mRNAs [Citation37]. The binding of YTHDC1 to target transcripts enabled the nuclear export and the abundance of cytoplasmic targets, contributing to translation initiation enhancement [Citation37]. YTHDC1 interacted key elements involved in mRNA exporting, such as serine and arginine-rich splicing factor 3 (SRSF3) and nuclear RNA export factor 1 (NXF1) [Citation37].
As for YTHDC2, it was shown to participate in the control of mRNA abundance and translation efficiency through recognizing the targeted m6A RNA [Citation40]. Ma et al. uncovered the crystal structure of human YTHDC2 [Citation40]. Knockdown of YTHDC2 could lead to a significant increase of m6A-decorated RNA, which was extremely critical for mammals in regulating mitosis and meiosis [Citation41,Citation42]. Insulin-like growth factor 2 mRNA-binding proteins (IGF2BP) 1–3 are newly characterized m6A readers and interact with m6A sites through their K homology domains [Citation43]. It was shown that IGF2BP1-3 acted to increase mRNA stability and prompted mRNA translation [Citation43,Citation44]. Moreover, the promotion of cap-independent translation was partially assisted by Eukaryotic initiation factor 3 (EIF3) another member of m6A readers [Citation45].
Heterogeneous nuclear ribonucleoprotein (hnRNP) A2B1, a direct nuclear reader of the m6A, was elucidated to interact with m6A-bearing RNAs both in vitro and in vivo [Citation46]. It took an important part in alternative splicing, which was similar to the effects elicited by METTL3 [Citation46]. Furthermore, HNRNPA2B1 could influence primary RNA (pri-miRNA) processing via interacting with the microprocessor complex protein DGCR8 [Citation46]. The mechanism of m6A-switch highlighted the role of m6A in controlling the RNA-structure-dependent accessibility of RNA-binding motifs (RBMs), thus impacting on RNA-protein crosstalk [Citation47]. It is notable that m6A-switch-induced enhancement of HNRNPC binding is responsible for the regulation of RNA expression and maturation [Citation47]. Similarly, HNRNPG was also found to be involved in RNA abundance and processing under the control of m6A-switch mode [Citation47].
Regulation of innate and adaptive immune cells
The innate immune system consisted of various myeloid and innate lymphoid lineages and acts to function as a rapid initial response against specific pathogens [Citation48]. Meanwhile, the innate immunity displays important effects on the activation and development of the adaptive immune system [Citation49]. Coordinated correlation among different immune cells is a hallmark of effective immune function, such as the orchestrated collaboration between B cells and T cells in the initiation of humoral immunity and in the context of immune memory. This specific cooperation could build developmental foundation for optimal affinity-matured antibody responses, which are essential for host defense and facilitate the progress of autoimmunity. In this part, the role of distinct immune cells was briefly introduced (). The systematic descriptions of the immune system can be obtained from these publications [Citation49,Citation50,Citation51]. Moreover, we focus on the current advances of m6A implicated in innate and adaptive immune cells. The interplay between m6A and innate and adaptive immune cells is summarized in .
Table 1. The interplay between m6A and innate and adaptive immune cells
Figure 1. The descriptions of innate immunity and adaptive immunity. Innate immunity and adaptive immunity are two main types of mammalian immune system, with the innate immune response as the first line of defence and protection, which primes and amplifies subsequent adaptive responses. Dendritic cells and macrophages are identified as important antigen-presenting cells (APCs) owing to their efficient ability to internalize pathogen and present pathogen peptides. T cells are generally classified into two groups expressing either cell surface CD4 or CD8 receptors. CD4 T cells are commonly referred to as helper T cells owing to their contribution to cytokine response. The activated CD4 T cells support the action of B cells to produce lots of antibodies. Once the same antigen infects the body again, memory B cells are stimulated to differentiate into effector B cells (plasma cells) to secrete large amounts of antibodies. The CD8 + T cells can be activated by the stimulation of cognate antigen and effector T cells can directly induce death in infected target cells. Meanwhile, a small group of memory T cells are left after antigen clearance
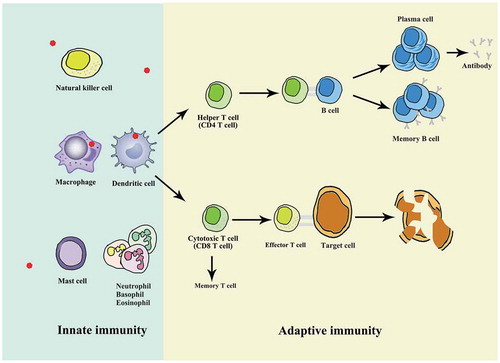
Macrophages
Macrophages are critical regulators of immune system [Citation52]. It acted to respond immediately to microbial infection and directly engulf or indirectly eliminate pathogens by releasing multiple inflammatory factors [Citation52]. Typically, the polarization of the classically activated macrophages (M1) or alternatively activated macrophages (M2) is perceived to be the driving force of various diseases [Citation53].
Recently, m6A modification has been determined to affect multiple biological activities, including those involved in macrophage polarization [Citation8]. Increased METTL3 acted to greatly promote M1 macrophage polarization, whereas it displayed the opposite effects on the M2 macrophage polarization [Citation8]. METTL3 directly methylated the transcription factor signal transducer and activator of transcription 1 (STAT1) and elevated the mRNA stability and the level of STAT1, thus facilitating M1 macrophage polarization [Citation8]. These observations highlighted the potential role of METTL3 as an inducer of M1-involved inflammatory diseases [Citation8].
A recent study by Gu et al. uncovered that FTO mediated the control of M1 and M2 in the same direction [Citation52,Citation53]. The level of m6A demethylase FTO was markedly impaired in M1 and M2 macrophages [Citation53]. It is notable that the NF-κB signalling pathway is inhibited after FTO knockdown, with decreased phosphorylation levels of IKKα/β, IκBα, and p65 [Citation53]. Moreover, the level of STAT1 was obviously decreased in M1-polarized macrophages and the level of peroxisome proliferation‐activated receptor‐γ (PPAR-γ) elicited the same trend in M2 polarization after FTO depletion [Citation53]. Collectively, FTO contributed to M1 and M2 macrophage activation in the same direction [Citation53]. These findings emphasized the critical role of m6A modification implicated in macrophages.
Dendritic cells
Dendritic cells (DCs) are specialized antigen-presenting cells (APCs) and serve as a bridge between innate and adaptive immune responses [Citation54]. It has been characterized as a class of bone-marrow-derived cells and stems from lympho-myeloid haematopoiesis [Citation55]. The dysregulation of DC activation is strongly correlated with various types of inflammatory, autoimmune, and other diseases [Citation56,Citation57]. In general, plasmacytoid DC (pDC), myeloid/conventional DC1 (cDC1) and myeloid/conventional DC2 (cDC2) consist the major subsets of DC [Citation55]. Currently, the breakthrough of the m6A epitranscriptome accelerates the understanding of m6A-dependent DC development and activation, presenting future prerequisite for translational exploitation of m6A-involved immunotherapy.
Wang et al. showed that METTL3 facilitated DC activation and function through influencing the translation of immune transcripts [Citation9]. Silencing of METTL3 in DC greatly decreased the expression level of CD40, CD80 and cytokine IL-12 and displayed an inverse effect on phenotypic and functional maturation of DC [Citation9]. Moreover, both in-vitro and in-vivo assays verified that DC-based T cell activation was remarkably impaired after METTL3 knockdown [Citation9]. The unveiling of innate immunity dependent on m6A-induced immune transcripts may shed new light on mechanism by which activated APC produces a prior expression of co-stimulatory elements and effectively initiates the immune response [Citation9].
Another study by Han et al. showed that the depletion of YTHDF1 in classical dendritic cells (cDCs) dramatically promoted the cross-presentation of tumour antigen and the cross-priming of CD8 + T cells in vivo [Citation11]. Liu et al. uncovered that knockdown of DC-specific lnc-Dpf3, a lncRNA, activated CCR7-mediated glycolytic metabolism, and DC migration via targeting HIF-1a, leading to the exaggerated inflammatory damage and adaptive immunity [Citation58]. It is worth noting that the dynamic expression of lnc-Dpf3 in DCs was altered in an m6A-dependent manner [Citation58]. The binding of YTHDF2 to lnc-Dpf3 led to a decreased level of lnc-Dpf3 in resting mature DCs [Citation58]. This observation provided a novel model of the coupling link between m6A epitranscriptome and metabolic pathways to feedback-control DC migration and inflammatory responses [Citation58].
Natural killer cells
Natural killer (NK) cells are a class of innate lymphoid immune cells and play an important role in immune surveillance [Citation59]. There is a growing number of studies showing that the regulation of NK cells can result from the crosstalk between NK cells and T cells [Citation59]. NK cells have been highlighted as key elements in both innate immunity and the regulation of the adaptive immune response [Citation59]. Han et al. demonstrated that the tumours obtained from YTHDF1−/− mice displayed a significantly higher level of NK cells than those derived from wild-type mice [Citation11]. These findings suggested the role of YTHDF1 deficiency in the activation of immunosurveillance [Citation11].
Myeloid-derived suppressor cells
Myeloid-derived suppressor cells (MDSCs) represent a heterogeneous population described in inflammatory and infectious diseases and in numerous tumours [Citation60]. MDSCs have been identified as precursors of DCs, macrophages, and granulocytes [Citation61]. Han and his colleagues evaluated the frequency of tumour-infiltrating MDSCs after 12-day tumour inoculation in wild-type or YTHDF1−/− mice [Citation11]. It was elucidated that the infiltration of MDSCs was significantly reduced in YTHDF1−/−mice than those from wild-type mice, highlighting the potential involvement of m6A in MDSC-associated immune cells [Citation11].
B cell development and B cell tumorigenesis
B cells are critical counterparts involved in the immunopathogenesis of various diseases [Citation62]. In detail, B cells could secrete antibodies to cause tissue injury and participate in disease pathogenesis in autoimmunity and alloimmunity through presenting antigens and offering costimulation and cytokines to T cells [Citation62]. Recently, Zheng et al. reported that B-cell-specific deletion of METTL14 could result in severe defects in B cell development, with inhibition of interleukin-7 (IL-7)-induced pro-B cell proliferation and blocking of large-pre-B-to-small-pre-B transition [Citation63]. By contrast, YTHDF1/2 displayed tight link with a failure to elevate key transcription factors involved in this transition [Citation63]. Collectively, m6A modification plays an essential role in cell state transitions during early B cell development [Citation63]. Another study directed by Zhang et al. identified the efficacy of m6A during B-cell development and B-cell tumorigenesis [Citation64]. It was found that half (5 of 10) of the m6A regulators could predict patient survival in mantle cell lymphoma, a type of non-Hodgkin B cell lymphoma [Citation64]. A comprehensive m6A model showed better performances in survival prediction compared with the efficacy of each individual m6A regulator, with a hazard ratio of 0.39 [Citation63]. Moreover, the aberrant level of m6A regulators was involved in the regulation of cell division and RNA metabolism pathways, leading to poor survival of mantle cell lymphoma [Citation64]. However, the research regarding m6A involvements in B cell development and tumorigenesis is still at the primary stage. Massive work should be devoted to identifying the specific role of each m6A regulator and synergistic correlation within m6A families in the regulation of B cell development and B cell tumorigenesis.
T cell development
T cells are continually arised in the thymus in a highly dynamic process and constitute the foundation for adaptive immunity [Citation65]. It can be generally classified into two groups expressing either cell surface CD4 or CD8 receptors [Citation66]. CD4 T cells are commonly referred to as helper T cells owing to their contribution to cytokine response [Citation66]. Meanwhile, the activated CD4 T cells can also support the action of B cells, leading to the production of large amounts of antibodies [Citation66].
Evidence has highlighted the fundamental role of m6A in regulating embryonic stem cells in vitro, implying that m6A may be involved in the mediation of T helper differentiation [Citation22]. Li et al. detected the impact of METTL3 in mouse T cells on T cell homeostasis and differentiation to reveal the in-vivo role of m6A in mammalian cells [Citation67]. In CD4-CRE conditional Mettl3 Lox/Lox mice, the loss of METTL3/METTL14 complex was shown in CD4 + T cells [Citation67]. Moreover, the absence of METTL3 in naïve T cells significantly reduced the proportion of Th1 and Th17 cells and markedly elevated the proportion of Th2 cells, but had no impact on Treg cells [Citation67]. The loss of METTL3 in naive T cells acted to prevent homeostatic expansion and enabled the maintenance of naïve state in the adoptive transfer model of a lymphopenic mouse [Citation67]. Mechanistically, m6A-mediated enhancement of SOCS family was able to synergistically inhibit IL-7/STAT5 pathway, thus inducing reprogramming of Naïve T cells for proliferation and differentiation [Citation67]. Concurrently, the activation of ERK/AKT pathway acted to inhibit naïve T cell proliferation and differentiation [Citation67]. Collectively, m6A was extremely essential not only for Th cell differentiation and proliferation in vivo, but also for T cells to properly exit the naïve ‘progenitor’ state [Citation67]. This observation illustrated the T cell-specific delivery of m6A-modifying agents and made new avenues for effective therapeutics against the autoimmune diseases [Citation67].
Shortly after, Furlan et al. reanalyzed the omics data from previous described research by Li and his colleagues, and synthetically elucidated the global consequences of m6A depletion on T cells RNA dynamics using INSPEcT tool [Citation68]. Further analysis proposed a yet unappreciated function for m6A in RNA synthesis and processing dynamics [Citation68]. Moreover, the depletion of m6A resulted in an overall decline for kinetic rates of RNA during T cells differentiation [Citation68]. The characterization of m6A through various advanced methods is able to further decipher the impact of m6A epitranscriptome on the regulation of T cell development [Citation68].
T cell immune function
CD8 + T cells play a leading part in protection against infections and reinfections of intra-cellular pathogens [Citation68]. The naive CD8 + T cells can be activated by the stimulation of cognate antigen and effector T cells can directly induce death in infected target cells [Citation69]. Meanwhile, a small group of memory T cells are left after antigen clearance [Citation69]. Current studies have elucidated the involvement of m6A in the regulation of CD8 + T cells both in vitro and in vivo [Citation11]. In contrast to the wide-type group, the anti-tumour response in YTHDF1−/− mice was completely abrogated in the absence of CD8 + T cells, highlighting the essential role of CD8 + T cells in the YTHDF1-deficient host [Citation11]. Concurrently, YTHDF1 deficiency in host cells intensifies the early steps of T cell priming against tumour neoantigens. Most recently, Jurczyszak et al. uncovered the crosstalk between human immunodeficiency virus (HIV) and m6A effector protein YTHDF3 during the early steps of the HIV life cycle [Citation70]. It was demonstrated that endogenous YTHDF3 expression restricted the cell’s susceptibility to HIV infection, since human CD4 + T cells with CRISPR-Cas9 edited YTHDF3 locus better supported HIV infection [Citation70]. This observation is largely consistent with the previous research reported by Lu and his colleagues, suggesting that viruses generated in YTHDF3-depleted cells are more infectious than those generated in wild-type cells [Citation71]. Another research performed by Li et al. demonstrated that lineage-specific deletion of METTL3 in CD4 + T cells contributed to the imbalance of naïve T cell homeostasis [Citation67]. In addition, the research team further detected the impact of m6A modification on Treg function in vivo and found that Tregs failed to exert inhibition effects in METTL3f/f; CD4-Cre knockout mice [Citation72]. Meanwhile, METTL3 deficiency in Treg cells contributed to the elevation of Socs genes targeting IL-2-STAT5 pathway, thereby controlling Treg functions and affecting the tumour-killing functions of CD8 + T cells [Citation72]. Moreover, Han et al. reported that there was no significant difference in the number of Treg cells in tumour samples from YTHDF1−/−mice [Citation11]. Above all, the efficiency of combined therapy with m6A regulators may offer new advantages for cancer immunotherapy.
Anti-inflammatory immunity
Currently, researchers have highlighted the role of m6A in anti-inflammatory immunity [Citation73]. Yu et al. firstly elucidated that YTHDF2 was involved in the inflammatory response of macrophages [Citation73]. The results demonstrated that YTHDF2 displayed marked elevation in macrophages with LPS stimulation and altered the stability of transcripts associated with inflammatory processes [Citation73]. Knockdown of YTHDF2 markedly increased the expression levels of IL-6, TNF-α, IL-1β, and IL-12, which were induced by LPS [Citation73]. In addition, the phosphorylation level of p65, p38, and ERK1/2 were also significantly upregulated in macrophages [Citation73]. What is more, silencing of YTHDF2 could induce remarkable upregulation of MAP2K4 and MAP4K4 through stabilizing the mRNA transcripts, thus activating MAPK and NF-κB signalling pathways and aggravating the inflammatory response in LPS-stimulated macrophages [Citation73]. Meanwhile, METTL3 and FTO were shown to be involved in M1/M2 activation, shedding new light on the therapeutics of inflammatory diseases [Citation8] [Citation53]. Liu et al. reported that YTHDF2 recognized and degraded DC-specific lnc-Dpf3, whose feedback largely inhibited CCR7-mediated DC migration and contributed to exaggerated adaptive immune responses and inflammatory responses [Citation58]. Above all, m6A involvement is critically important in inflammatory immunity and may serve as a novel target for anti-inflammatory therapeutics. The crosstalk between m6A and anti-inflammatory immunity was depicted in .
Anti-viral immunity
Studies have attached great importance on the role of m6A in virus–host interaction [Citation74]. Zheng et al. uncovered that ALKBH5 was critically recruited by DDX46 to enforce the retention of the mitochondrial antiviral signalling protein (Mavs), TNF receptor-associated factor 3 (TRAF3) and TRAF6 transcripts in the nucleus, thereby impacting on negative effects on the antiviral innate immunity [Citation7]. The crosstalk between m6A and anti-viral immunity was depicted in . Meanwhile, current advances of viral RNA and DNA regulation by m6A were summarized in . Better understanding of anti-viral immunity greatly facilitated the development of new strategies against viral infection.
Table 2. Summary of viral RNA and DNA regulation by m6A
HIV
HIV is a retrovirus responsible for causing acquired immune deficiency syndrome (AIDS) [Citation75]. The abundance of m6A at the 3′-UTR of HIV-genomic RNA mediated the interaction between host and pathogen [Citation76]. Lichinchi et al. and Tirumuru et al. revealed multiple m6A sites in RNA genome [Citation76,[Citation77,Citation77]. The presence of two m6A sites within HIV-1 Rev response element (RRE) stem IIB was determined to interact with viral protein Rev and to promote viral RNA export [Citation76,Citation77]. In contrast, Kennedy et al. found four clusters of m6A modifications in the 3ʹ UTR region of the HIV-1 RNA genome that enhanced viral gene expression, with no detection of proposed RRE m6A sites [Citation78]. The implication of various mapping techniques, cell lines and diverse reagents may account for different results from different laboratories. Specifically, the depletion of METTL3/METTL14 led to the inhibition of HIV replication, whereas silencing of ALKBH5 elicited the opposite effects [Citation76,Citation78]. As for YTHDF1-3, it was verified to suppress HIV-1 RNA through inhibiting HIV-1 reverse transcription and induced Gag expression level in virus-producing cells, implying the pivotal role of m6A in HIV-1 RNA infection and HIV-1 protein synthesis [Citation76,Citation78].
Another study reported by Karikó and his colleagues uncovered that m6A-decorated viral RNA was involved in the regulation of antiviral innate immune evasion [Citation79]. The authors showed that the presence of m6A within viral RNA significantly repressed the activation of toll-like receptor 3 (TLR3) signalling [Citation79]. Moreover, transcripts with altered m6A level were identified to participate in immune response and inflammation, which was quite consistent with expression pattern in HIV Tg rats and patients with HIV [Citation80,Citation81]. It is well known that the HIV genome harbours potential G-quadruplex-forming sequences (PQSs), which acts to fold in the DNA and RNA [Citation82]. The installation of m6A within the loops of two-tetrad PQSs in the RNA genomes of the HIV acted to drive the formation of G-quadruplexe (G4) folds, thus mediating replication of the viral genome [Citation82]. The synergistic crosstalk between m6A and G4 folds may add a new layer to the impact of viral epitranscriptome [Citation82]. As for the interplay between YTHDF3 and HIV replication, the latest research reported by Jurczyszak et al. showed that YTHDF3 functioned as a negative factor in HIV infection [Citation70]. HIV protease cleaves the virion-incorporated YTHDF3 protein and viral polyproteins, thus permitting the optimal infectivity of the mature virion [Citation70]. This newly-identified mode of m6A effector in HIV infection and replication may offer new antiviral therapeutics for patients with HIV [Citation70].
Influenza A virus
Influenza A virus (IAV), a negative sense ssRNA virus, was initially found to bear 24 m6A residues on various viral mRNAs [Citation83,Citation84]. Courtney et al. provided the detection of m6A mapping in IAV and demonstrated that METTL3 inactivation displayed repressive effects on IAV replication [Citation85]. Another study by Winkler et al. further clarified that IAV gene expression in METTL3-depleted cells was demonstrated to be partly rescued by the depletion of interferon signalling, suggesting that the depletion of m6A machinery led to an elevated type I interferon response following the infection with IAV [Citation86]. Overexpressed YTHDF2 protein activated IAV replication and infectious particle production [Citation85], whereas ectopic level of YTHDF1 and YTHDF3 proteins displayed no impact on IAV replication and viral production [Citation85]. Meanwhile, the m6A-deficient IAV mutants showed decreased IAV pathogenicity in mice [Citation85]. Importantly, m6A-induced viral RNA can effectively prevent the activation of innate immune sensor RIG-I or MDA5, thus mediating the anti-viral immunity in IAV [Citation87]. However, the specific mechanism by which m6A enhanced IAV gene expression and replication remained to be clarified.
Flaviviridae family
Recent investigations have opened the mysteries of m6A mapping on flaviviridae family, such as hepatitis C virus (HCV), Zika virus (ZIKV) and dengue virus (DENV) [Citation10,Citation74]. The crosstalk between m6A and infection of flaviviridae family may shed new light on the knowledge of how RNA modifications affect flaviviridae infection [Citation10,Citation74].
HCV is characterized as a disease with chronic progression during infection and its slow replicated rates display close association with an evasion of immune surveillance [Citation88]. It is possible that m6A mediates the viral fitness of HCV and enables the slow and continuous infection of HCV. Recently, Gokhale et al. revealed that the loss of METTL3/METTL14 complex led to the enhancement of HCV infection through promoting production of infectious viral particle [Citation74]. Meanwhile, YTHDF proteins were recognized to relocalize to sites of HCV particle production and prevented this stage of viral infection [Citation74]. Conversely, FTO depletion, but not ALKBH5, displayed the opposite effects [Citation74]. Importantly, it is possible that m6A participates in the segregation of RNA genome among each stage of HCV life cycle [Citation74]. The presence of m6A on HCV RNA genome may contribute to antiviral innate immune evasion owing to the inhibition of TLR3 signalling [Citation74]. Indeed, more works should be done to comprehensively clarify the involvement of m6 in anti-HCV immunity. These findings may better the understanding of m6A in flaviviridae infection and offer prospective therapeutics for viral pathogens [Citation74].
Similar to HCV, ZIKV, and DENV contained m6A peaks within their envelope genes [Citation74]. Further verification conducted by Gokhale et al. demonstrated that DENV contained m6A within their 3′-UTRs, which had two stem-loops to mediate the transmission between mosquito and human [Citation74,Citation89]. In addition, a definite distinction was observed in m6A distribution between the Dakar and the Puerto Rican isolates of ZIKV [Citation74]. This difference between African and the Asian lineages directed by m6A may lead to altered pathogenicity and infection outcomes [Citation74]. Lichinchi et al. uncovered that ZIKV infection acted to impact on the role and topology structure of m6A [Citation90]. Exactly, 12 m6A peaks were distributed across the full length of ZIKV RNA [Citation90]. Upon ZIKV infection, the 5′-UTR hypermethylation of host transcripts was detected [Citation90]. Importantly, these host immune-related transcripts were obviously changed in the process of ZIKV infection, implying the promoting effect of m6A on antiviral response [Citation90]. The depletion of METTL3/METTL14 complex significantly prompted ZIKV production, whereas silencing of ALKBH5 and FTO displayed reverse effects [Citation90]. Moreover, YTHDF family proteins were demonstrated to interact with ZIKV RNA and to inhibit ZIKV replication [Citation90]. Collectively, the interplay between m6A and viral RNA resulted in the inhibition of the host antiviral response. These findings may narrow the gap in understanding the m6A-mediated mechanism governing the viral life cycle and viral interactions with host cells [Citation90].
Kaposi’s sarcoma-associated herpesvirus
Kaposi’s sarcoma-associated herpesvirus (KSHV), an oncogenic double-stranded DNA virus, is identified to enter a latent phase shortly after primary infection [Citation91]. Notably, the latent infection of KSHV is tightly linked to the host immune responses and impacts on KSHV-associated diseases [Citation92]. The latent virus becomes reactivated and starts lytic replication to provide new viruses in the context of immunosuppression [Citation92]. It is worth noting that m6A-decorated RNA exhibits tight correlation with immune sensors, such as TLR3 and RIG-I, thus mediating innate antiviral immune response [Citation87].
A recent study by Tan et al. showed that the topology of the latent KSHV epitranscriptome was remarkably conserved across different cell types and the latent locus of ORF71/72/73 was the most consistently methylated locus in these latently infected cells, highlighting the potential involvement of m6A in viral latency [Citation93]. It was observed that the regulation of KSHV latency and cellular transformation was closely associated with various pathways, such as cytoskeleton and extracellular matrix signalling, cell-cell junction signalling, Rho signalling, ILK signalling, Ephrin signalling, endocytosis, and protein ubiquitination pathways, indicating that KSHV may prompt viral latency and tumorigenesis through regulating m6A-directed cellular transcripts [Citation93]. In addition, further efforts should be made to clarify viral factors contributed to the altered distribution of cellular m6A [Citation93]. Then, Tan and his colleagues further attempted to reveal the role of m6A during KSHV lytic replication [Citation93]. It is notable that YTHDF2 degrades viral transcripts and acts as a negative regulator for KSHV lytic replication [Citation93]. Conversely, Hesser et al. revealed that YTHDF2 acted to exert pro-viral roles and induced reverse impact on the m6A-directed ORF50 protein in different KSHV-infected cells [Citation94]. The main reason accounting for the opposed results will be uncovered when the implication of rapid conditional knockdown of m6A-binding proteins or specific inhibitors is feasible, thus revealing the impact of m6A on different time points [Citation93].
Ye et al. determined the function of m6A-mediated KSHV transcripts in regulating viral lytic gene expression and replication [Citation95]. It was observed that TPA activated the transcription of KSHV lytic genes and its silencing obviously inhibited FTO level, leading to an increased level of m6A and KSHV lytic gene expression [Citation95]. By contrast, METTL3 knockdown exhibited the opposite impact [Citation95]. As for YTHDC1, it acted to promote the splicing of KSHV replication transcription activator, thus inducing KSHV lytic replication [Citation95]. Most recently, RIP-seq and enhanced crosslinking and immunoprecipitation (eCLIP) analysis uncovered the staphylococcal nuclease and tudor domain containing 1 (SND1) binding profile, identifying SND1 as an m6A reader [Citation96]. Baquero-Perez et al. elucidated that SND1 identified template mRNAs containing m6A from KSHV and host cells, thus increasing protein involved in virus replication [Citation96]. Consistently, the activity of virus replication was significantly decreased in KSHV-infected human immune cells with SND1 deficiency [Citation96]. Meanwhile, knockdown of SND1 markedly suppressed KSHV early gene expression, revealing the key involvement of SND1 in KSHV lytic replication [Citation96].
Simian virus 40
Early in 1979, transcripts encoded by simian virus 40 (SV40), a simian polyomavirus, was detected to contain several m6A residues, which was also testified in the study provided by Kahana and his colleagues [Citation97,Citation98]. An average of three m6A residues per mRNA molecule was found within late SV40 16S and 19S mRNAs, occurring in sequences of Gpm6ApC and (Ap)nm6ApC [Citation97]. This observation gave the first evidence of specific localization of internal m6A decoration in coding regions of SV40 mRNAs [Citation97]. Shortly after, Finkel et al. demonstrated that m6A acted to regulate the nuclear to cytoplasmic transport of mRNA in SV40-nontransformed cells [Citation99]. Moreover, the altered structures within perinuclear regions in SV40-transformed cells may lead to the escape of these impacts [Citation99]. A recent study by Tsai et al. elucidated that approximately eleven potential m6A sites were detected in the late and early regions of SV40, respectively, using photo-cross-linking-assisted m6A sequencing (PA-m6A-seq) [Citation100]. The mutation of selective m6A sites could significantly inhibit viral gene expression and virion production [Citation100]. In detail, it was first demonstrated that overexpressed YTHDF2 protein contributed to boosted viral replication and viral plaques in SV40 infected BSC40 cells [Citation100]. Consistently, mutated YTHDF2 or METTL3 was shown to exhibit the opposite impact, identifying an m6A-directed positive role in the SV40 gene regulation [Citation100]. Overall, identification of m6A addition is a critical step for SV40 life cycle and may provide new insight into therapeutics for human polyomaviruses.
Hepatitis B virus
Hepatitis B virus (HBV), a DNA virus in hepadnaviridae family, has been identified as an important risk factor for developing cirrhosis and hepatocellular carcinoma (HCC) [Citation101]. Imam et al. revealed an m6A consensus motif within the epsilon stem-loop of HBV RNAs [Citation102]. Silencing of METTL3/METTL14 increased the stability of the HBV transcripts and reversed transcription of pgRNA [Citation102]. The depletion of YTHDF2 or YTHDF3 displayed similar effects [Citation102]. As for FTO and ALKBH5, their decreased level exhibited the opposite results [Citation102]. It was found that m6A played a positive effect on reverse transcription [Citation102]. Conversely, m6A displayed a negative impact on the stability of HBV mRNAs, leading to the inhibition of HBV viral proteins [Citation102]. It is highly possible that m6A modification of HBV may offer a new understanding of HBV pathogenesis.
Most recently, Imam et al. further elucidated that interferon-stimulated genes 20 (ISG20) could selectively recognize m6A-directed HBV transcripts to induce degradation [Citation103]. The degradation of HBV transcripts induced by ISG20 was obviously suppressed in HBV expressing cells with the knockdown of METTL3/METTL14 or YTHDF2 [Citation103]. Above all, this study gave the first evidence of the assembled complex of ISG20/YTHDF2/HBV RNA, paving a new therapeutic avenue for HBV patients [Citation103].
Anti-tumour immunity
Evidence has highlighted the involvement of m6A in regulating malignant phenotype and tumour intrinsic oncogenic pathways [Citation104,Citation105], which has been roundly summarized in other reviews [Citation106]. Currently, researchers have begun to reveal the mysteries of m6A-induced impact on anti-tumour immunity [Citation11]. In this section, we focus on the advances of m6A involvements in anti-tumour immunoregulation and further discuss the deficiencies in this research field. The crosstalk between m6A and anti-tumour immunity was depicted in .
Su et al. reported that R-2-hydroxyglutarate (R-2HG), an oncometabolite, showed a broad anti-leukemic activity with inhibition of leukaemia cell proliferation and activation of apoptosis [Citation107]. R-2HG was found to inhibit FTO activity through elevating the global level of m6A in R-2HG-sensitive leukaemia cells, thus leading to the suppression of the stability of MYC and CEBPA [Citation107]. Subsequently, Su et al. further uncovered that the genetic depletion and pharmacological inhibition of FTO obviously suppressed the self-renewal of leukaemia stem cell and induced immune response through inhibiting expression of immune checkpoint gene expression [Citation108]. It was shown that targeting FTO could sensitize leukaemia cells to T cell cytotoxicity and overcome hypomethylating agent-induced immune evasion, implying the potential value of targeting FTO in cancer therapeutics [Citation108]. Chen et al. found that YTHDF2 could sequester m6A-modified circRNA and suppress innate immunity [Citation109]. As for unmodified circRNAs, it functioned as effective adjuvants to induce antigen-specific T cell activation, antibody production, and anti-tumour immunity [Citation109].
It is well known that the lack of adequate and durable antitumor immune response contributes to the incomplete tumour elimination in patients with malignant diseases [Citation110]. Han et al. uncovered the mysteries behind the durable neoantigen-specific immunity were closely linked to m6A-dependent mechanism [Citation11]. In-vivo assays demonstrated that antigen-specific CD8 + T cell antitumor response was significantly improved in YTHDF1-deficient (YTHDF1−/−) mice as compared with wild-type group [Citation11]. Furthermore, the anti-tumour response in the YTHDF1-deficient host was fully eliminated in the context without CD8 + T cells [Citation11]. Interestingly, YTHDF1−/− mice displayed a significantly larger amount of IFN-γ spot-forming cells than in WT mice, implying that decreased YTHDF1 played a positive impact on the early steps of T-cell priming against tumour neoantigens [Citation11]. Finally, the authors uncovered that PD-L1 blockade could induce complete tumour regression in YTHDF1 −/− mice [Citation11]. Collectively, the combined targeting of a checkpoint blockade with YTHDF1 deficiency could bring advantages to patients with low response to checkpoint blockade [Citation11]. Importantly, more efforts should be paid to further the current knowledge of m6A-induced complexity in anti-tumour immunity.
Most recently, FTO was determined to significantly activate melanoma progression, while METTL3 and METTL14 generated the opposite effects [Citation31]. Meanwhile, FTO significantly elevated the expression level of PD-1, CXCR4, and SOX10 in melanoma and mediated the tumour response against anti-PD-1 blockade through m6A-mediated mechanism [Citation31]. These findings suggested that the combined targeting of FTO knockdown with anti-PD-1 blockade may result in elevated sensitivity to immunotherapy and boosted anti-melanoma response [Citation31]. At present, m6A epitranscriptome and anti-tumour immunity are gaining a great deal of interest in scientific community. However, the current knowledge is still in its infancy owing to the limitation of techniques and high expenses. Further identification of other m6A-related modulators may largely enrich the current mechanism of tumour immune regulation and provide effective anti-tumour therapeutics for patients with cancers.
Therapeutic efficacy of M6A regulators in immunotherapy
Currently, evidence has demonstrated the m6A involvements in immune regulation, highlighting the therapeutic efficacy of m6A regulators in immunotherapy [Citation108,Citation111]. In this section, we provide a summary of the therapeutic efficacy of m6A regulators in immunotherapy. The knowledge of m6A regulators implicated in various cancers and their intervention on various clinical treatments, such as chemotherapy and radiotherapy, has been well summarized in other reviews, and we will not repeat here [Citation14,Citation16,Citation106].
Li et al. reported that the ALKBH5 gene mutation and expression status of melanoma patients tightly linked with their response to immunotherapy [Citation111]. ALKBH5 knockout in tumour cells contributed to the enhanced efficacy of immunotherapy, identifying the therapeutic value of ALKBH5 in melanoma immunotherapy [Citation111]. Shen et al. revealed that ALKBH5 was essential for AML pathogenesis and leukaemia stem/initiating cells (LSCs/LICs) self-renewal, but not normal hematopoiesis [Citation112]. Therefore, shen et al. reported that targeting ALKBH5 represented a very promising strategy for AML patients [Citation112]. However, subsequent verified assays and clinical experiments are in an urgent need. Additionally, YTHDF1 could improve the therapeutic efficacy of immunotherapy through regulating transcripts encoding lysosomal proteases [Citation11]. Recently, Yang et al. found that the depletion of FTO increased the sensitivity of tumour cells to interferon gamma (IFNg) and prompted melanoma response to anti-PD-1 antibody in mice, highlighting that the combination of m6A regulators and anti-PD-1/PD-L1 blockade may improve the efficacy of anticancer immunotherapy [Citation31]. Shortly after, Su et al. revealed that targeting FTO by effective inhibitors could inhibit immune checkpoint gene expression and induce immune evasion, implying the potential of targeting FTO in cancer therapeutics [Citation108]. Most recently, Xu et al. detected the m6A regulator level between high and low lung squamous cell carcinoma (LUSC) risk patients [Citation113]. It was shown that high-risk LUSC patients had significantly lower rates of ALKBH5, METTL3, HNRNPC, and KIAA1429 compared with the proportions of low-risk LUSC group [Citation113]. Notably, high-risk LUSC patients displayed more promising treatment to respond to anti-PD-1 treatments [Citation113]. Overall, identification of m6A involvements in immune system may shed new light on current therapeutics, including immunotherapy. Importantly, in-depth investigations of m6A-involved mechanisms and advancements of targeting technologies are urgently needed to facilitate the translational progress.
Conclusions and perspectives
The advancements of high-throughput technologies greatly facilitated the identification of m6A modification in multiple RNA species [Citation114]. The research field of m6A epitranscriptome has attracted tremendous attention in the last several decades. Efforts have been devoted to detecting m6A involvements in innate immunity and adaptive immunity [Citation11]. Moreover, a tight correlation has been found between m6A readers and MDSCs, which are precursors of DCs, macrophages, and granulocytes [Citation8,Citation11].
Researchers have highlighted the m6A regulators as key layers in mediating anti-inflammatory, anti-viral and anti-tumour immunity [Citation8,Citation11]. Deciphering the m6A epitranscriptome in diverse virus infection and malignancies is of vital significance to reveal new pathogenic pathways and develop novel strategies. For instance, targeting of m6A writers and erasers with small molecule inhibitors is a prospective anti-viral strategies [Citation115]. Moreover, Han et al. highlighted that the combined targeting of a checkpoint blockade with YTHDF1 deficiency may bring extra benefits to patients with low response to checkpoint blockade [Citation11]. However, the exact role of m6A in anti-bacterial immunity required much attention despite the detection of m6A in bacteria within various species. Based on the current research status, m6A epitranscriptome opens a new charter in immune system logic. However, there are still massive questions and controversies to be regarding the m6A epitranscriptome and how this dynamic modification altered the critical elements and steps involved in the immune regulation. The controversy among different m6A-related studies can derive from the implication of various mapping techniques, cell lines, and diverse reagents. Therefore, a large quantity of studies are urgently required to enrich current knowledge and fill the scientific gap. In conclusion, the m6A epitranscriptome opens a new charter in immune system logic and may have great usages as promising therapeutic targets of the disease.
Acknowledgments
ZHM, XFX and JFJ developed the concept. ZHM, XYG and YS reviewed the literature, wrote the manuscript and prepared the tables and figures. JFJ and XFX provided the full guidance. All authors read and approved the final manuscript.
Disclosure statement
The authors report no conflict of interest.
Additional information
Funding
References
- Delaunay S, Frye M. RNA modifications regulating cell fate in cancer. Nat Cell Biol. 2019;21(5):552–559.
- Desrosiers R, Friderici K, Rottman F. Identification of methylated nucleosides in messenger RNA from Novikoff hepatoma cells. Proc Natl Acad Sci U S A. 1974;71(10):3971–3975.
- Chen Z, Zhao P, Li F, et al. Comprehensive review and assessment of computational methods for predicting RNA post-transcriptional modification sites from RNA sequences. Brief Bioinform. 2019. DOI:10.1093/bib/bbz112
- Meyer KD. DART-seq: an antibody-free method for global m(6)A detection. Nat Methods. 2019;16(12):1275–1280.
- Liu J, Li K, Cai J, et al. Landscape and regulation of m(6)A and m(6)am methylome across human and mouse tissues. Mol Cell. 2020;77(2):426–440.
- Yang Y, Hsu PJ, Chen YS, et al. Dynamic transcriptomic m(6)A decoration: writers, erasers, readers and functions in RNA metabolism. Cell Res. 2018;28(6):616–624.
- Zheng Q, Hou J, Zhou Y, et al. The RNA helicase DDX46 inhibits innate immunity by entrapping m(6)A-demethylated antiviral transcripts in the nucleus. Nat Immunol. 2017;18(10):1094–1103.
- Liu Y, Liu Z, Tang H, et al. The N(6)-methyladenosine (m(6)A)-forming enzyme METTL3 facilitates M1 macrophage polarization through the methylation of STAT1 mRNA. Am J Physiol Cell Physiol. 2019;317(4):C762–C775.
- Wang H, Hu X, Huang M, et al. Mettl3-mediated mRNA m(6)A methylation promotes dendritic cell activation. Nat Commun. 2019;10(1):1898.
- Gokhale NS, McIntyre A, Mattocks MD, et al. Altered m(6)A modification of specific cellular transcripts affects flaviviridae infection. Mol Cell. 2020;77(3):542–555.
- Han D, Liu J, Chen C, et al. Anti-tumour immunity controlled through mRNA m(6)A methylation and YTHDF1 in dendritic cells. Nature. 2019;566(7743):270–274.
- Sledz P, Jinek M. Structural insights into the molecular mechanism of the m(6)A writer complex. Elife. 2016;5. DOI:10.7554/eLife.18434.
- Liu J, Yue Y, Han D, et al. A METTL3-METTL14 complex mediates mammalian nuclear RNA N6-adenosine methylation. Nat Chem Biol. 2014;10(2):93–95.
- Zhang S. Mechanism of N(6)-methyladenosine modification and its emerging role in cancer. Pharmacol Ther. 2018;189(173–183). DOI:10.1016/j.pharmthera.2018.04.011
- Hu Y, Wang S, Liu J, et al. New sights in cancer: component and function of N6-methyladenosine modification. Biomed Pharmacother. 2020;122(109694). DOI:10.1016/j.biopha.2019.109694
- Lan Q, Liu PY, Haase J, et al. The critical role of RNA m(6)A methylation in cancer. Cancer Res. 2019;79(7):1285–1292.
- Mendel M, Chen KM, Homolka D, et al. Methylation of structured RNA by the m(6)A writer METTL16 is essential for mouse embryonic development. Mol Cell. 2018;71(6):986–1000.
- Wang X, Feng J, Xue Y, et al. Structural basis of N(6)-adenosine methylation by the METTL3-METTL14 complex. Nature. 2016;534(7608):575–578.
- Ping XL, Sun BF, Wang L, et al. Mammalian WTAP is a regulatory subunit of the RNA N6-methyladenosine methyltransferase. Cell Res. 2014;24(2):177–189.
- Lan T, Li H, Zhang D, et al. KIAA1429 contributes to liver cancer progression through N6-methyladenosine-dependent post-transcriptional modification of GATA3. Mol Cancer. 2019;18(1):186.
- Knuckles P, Lence T, Haussmann IU, et al. Zc3h13/Flacc is required for adenosine methylation by bridging the mRNA-binding factor Rbm15/Spenito to the m(6)A machinery component Wtap/Fl(2)d. Genes Dev. 2018;32(5–6):415–429.
- Wang Y, Li Y, Toth JI, et al. N6-methyladenosine modification destabilizes developmental regulators in embryonic stem cells. Nat Cell Biol. 2014;16(2):191–198.
- Zhou KI, Pan T. Structures of the m(6)A methyltransferase complex: two subunits with distinct but coordinated roles. Mol Cell. 2016;63(2):183–185.
- Yao QJ, Sang L, Lin M, et al. Mettl3-Mettl14 methyltransferase complex regulates the quiescence of adult hematopoietic stem cells. Cell Res. 2018;28(9):952–954.
- Pendleton KE, Chen B, Liu K, et al. The U6 snRNA m(6)A methyltransferase METTL16 regulates SAM synthetase intron retention. Cell. 2017;169(5):824–835.
- Frayling TM, Timpson NJ, Weedon MN, et al. A common variant in the FTO gene is associated with body mass index and predisposes to childhood and adult obesity. Science. 2007;316(5826):889–894.
- Jia G, Fu Y, Zhao X, et al. N6-methyladenosine in nuclear RNA is a major substrate of the obesity-associated FTO. Nat Chem Biol. 2011;7(12):885–887.
- Huang Y, Su R, Sheng Y, et al. Small-molecule targeting of oncogenic FTO demethylase in acute myeloid leukemia. Cancer Cell. 2019;35(4):677–691.
- Li Z, Weng H, Su R, et al. FTO plays an oncogenic role in acute myeloid leukemia as a N(6)-methyladenosine RNA demethylase. Cancer Cell. 2017;31(1):127–141.
- Wu R, Liu Y, Yao Y, et al. FTO regulates adipogenesis by controlling cell cycle progression via m(6)A-YTHDF2 dependent mechanism. Biochim Biophys Acta Mol Cell Biol Lipids. 2018;1863(10):1323–1330.
- Yang S, Wei J, Cui YH, et al. m(6)A mRNA demethylase FTO regulates melanoma tumorigenicity and response to anti-PD-1 blockade. Nat Commun. 2019;10(1):2782.
- Tang C, Klukovich R, Peng H, et al. ALKBH5-dependent m6A demethylation controls splicing and stability of long 3ʹ-UTR mRNAs in male germ cells. Proc Natl Acad Sci U S A. 2018;115(2):E325–E333.
- Patil DP, Pickering BF, Jaffrey SR. Reading m(6)A in the transcriptome: m(6)A-binding proteins. Trends Cell Biol. 2018;28(2):113–127.
- Wang X, Zhao BS, Roundtree IA, et al. N(6)-methyladenosine modulates messenger RNA translation efficiency. Cell. 2015;161(6):1388–1399.
- Shi H, Wang X, Lu Z, et al. YTHDF3 facilitates translation and decay of N(6)-methyladenosine-modified RNA. Cell Res. 2017;27(3):315–328.
- Jin D, Guo J, Wu Y, et al. m(6)A mRNA methylation initiated by METTL3 directly promotes YAP translation and increases YAP activity by regulating the MALAT1-miR-1914-3p-YAP axis to induce NSCLC drug resistance and metastasis. J Hematol Oncol. 2019;12(1):135.
- Roundtree IA, Luo GZ, Zhang Z, et al. YTHDC1 mediates nuclear export of N(6)-methyladenosine methylated mRNAs. Elife. 2017;6. DOI:10.7554/eLife.31311.
- Liu J, Dou X, Chen C, et al. N (6)-methyladenosine of chromosome-associated regulatory RNA regulates chromatin state and transcription. Science. 2020;367(6477):580–586.
- Wu R, Jiang D, Wang Y, et al. N (6)-methyladenosine (m(6)A) methylation in mRNA with a dynamic and reversible epigenetic modification. Mol Biotechnol. 2016;58(7):450–459.
- Ma C, Liao S, Zhu Z. Crystal structure of human YTHDC2 YTH domain. Biochem Biophys Res Commun. 2019;518(4):678–684.
- Bailey AS, Batista PJ, Gold RS, et al. The conserved RNA helicase YTHDC2 regulates the transition from proliferation to differentiation in the germline. Elife. 2017;6. DOI:10.7554/eLife.26116.
- Soh Y, Mikedis MM, Kojima M, et al.:Meioc maintains an extended meiotic prophase I in mice. Plos Genet. 2017;13(4):e1006704.
- Huang H, Weng H, Sun W, et al. Author correction: recognition of RNA N(6)-methyladenosine by IGF2BP proteins enhances mRNA stability and translation. Nat Cell Biol. 2018;20(9):1098.
- Samuels TJ, Jarvelin AI, Ish-Horowicz D, et al. Imp/IGF2BP levels modulate individual neural stem cell growth and division through myc mRNA stability. Elife. 2020;9. DOI:10.7554/eLife.51529.
- Hutt DM, Loguercio S, Roth DM, et al. Correcting the F508del-CFTR variant by modulating eukaryotic translation initiation factor 3-mediated translation initiation. J Biol Chem. 2018;293(35):13477–13495.
- Alarcon CR, Goodarzi H, Lee H, et al. HNRNPA2B1 is a mediator of m(6)A-dependent nuclear RNA processing events. Cell. 2015;162(6):1299–1308.
- Liu N, Dai Q, Zheng G, et al. N(6)-methyladenosine-dependent RNA structural switches regulate RNA-protein interactions. Nature. 2015;518(7540):560–564.
- Laslo P, Pongubala JM, Lancki DW, et al. Gene regulatory networks directing myeloid and lymphoid cell fates within the immune system. Semin Immunol. 2008;20(4):228–235.
- Ziauddin J, Schneider DS. Where does innate immunity stop and adaptive immunity begin? Cell Host Microbe. 2012;12(4):394–395.
- Parkin J, Cohen B. An overview of the immune system. Lancet. 2001;357(9270):1777–1789.
- Tomar N. De RK A brief outline of the immune system. Methods Mol Biol. 2014;1184(3–12). DOI:10.1007/978-1-4939-1115-8_1
- Raggi F, Pelassa S, Pierobon D, et al. Regulation of human macrophage M1-M2 polarization balance by hypoxia and the triggering receptor expressed on myeloid cells-1. Front Immunol. 2017;8(1097). DOI:10.3389/fimmu.2017.01097
- Gu X, Zhang Y, Li D, et al. N6-methyladenosine demethylase FTO promotes M1 and M2 macrophage activation. Cell Signal. 2020;69(109553). DOI:10.1016/j.cellsig.2020.109553
- Ait-Oufella H, Sage AP, Mallat Z, et al. Adaptive (T and B cells) immunity and control by dendritic cells in atherosclerosis. Circ Res. 2014;114(10):1640–1660.
- Granot T, Senda T, Carpenter DJ, et al. Dendritic cells display subset and tissue-specific maturation dynamics over human life. Immunity. 2017;46(3):504–515.
- Palomino-Segura M, Perez L, Farsakoglu Y, et al. Protection against influenza infection requires early recognition by inflammatory dendritic cells through C-type lectin receptor SIGN-R1. Nat Microbiol. 2019;4(11):1930–1940.
- Saadeh D, Kurban M, Abbas O. Update on the role of plasmacytoid dendritic cells in inflammatory/ autoimmune skin diseases. Exp Dermatol. 2016;25(6):415–421.
- Liu J, Zhang X, Chen K, et al. CCR7 chemokine receptor-inducible lnc-Dpf3 restrains dendritic cell migration by inhibiting HIF-1alpha-mediated glycolysis. Immunity. 2019;50(3):600–615.
- Poli A, Michel T, Patil N, et al. Revisiting the functional impact of NK cells. Trends Immunol. 2018;39(6):460–472.
- Penaloza HF, Alvarez D, Munoz-Durango N, et al. The role of myeloid-derived suppressor cells in chronic infectious diseases and the current methodology available for their study. J Leukoc Biol. 2019;105(5):857–872.
- Ko HJ, Lee JM, Kim YJ, et al. Immunosuppressive myeloid-derived suppressor cells can be converted into immunogenic APCs with the help of activated NKT cells: an alternative cell-based antitumor vaccine. J Immunol. 2009;182(4):1818–1828.
- Hoffman W, Lakkis FG, Chalasani G, et al. Antibodies, and more. Clin J Am Soc Nephrol. 2016;11(1):137–154.
- Zheng Z, Zhang L, Cui XL, et al. Control of early B cell development by the RNA N(6)-methyladenosine methylation. Cell Rep. 2020;31(13):107819.
- Zhang W, He X, Hu J, et al. Dysregulation of N(6)-methyladenosine regulators predicts poor patient survival in mantle cell lymphoma. Oncol Lett. 2019;18(4):3682–3690.
- Takaba H, Takayanagi H. The mechanisms of T cell selection in the thymus. Trends Immunol. 2017;38(11):805–816.
- Scully C, Georgakopoulou EA, Hassona Y. The immune system: basis of so much health and disease: 4. immunocytes. Dent Update. 2017;44(5):436–438, 441–442.
- Li HB, Tong J, Zhu S, et al. m(6)A mRNA methylation controls T cell homeostasis by targeting the IL-7/STAT5/SOCS pathways. Nature. 2017;548(7667):338–342.
- Furlan M, Galeota E, de Pretis S, et al. m6A-dependent RNA dynamics in T cell differentiation. Genes (Basel). 2019;10(1). DOI:10.3390/genes10010028
- Gerritsen B, Pandit A. The memory of a killer T cell: models of CD8(+) T cell differentiation. Immunol Cell Biol. 2016;94(3):236–241.
- Jurczyszak D, Zhang W, Terry SN, et al. HIV protease cleaves the antiviral m6A reader protein YTHDF3 in the viral particle. Plos Pathog. 2020;16(2):e1008305.
- Lu W, Tirumuru N, St GC, et al. N(6)-methyladenosine-binding proteins suppress HIV-1 infectivity and viral production. J Biol Chem. 2018;293(34):12992–13005.
- Tong J, Cao G, Zhang T, et al. m(6)A mRNA methylation sustains Treg suppressive functions. Cell Res. 2018;28(2):253–256.
- Yu R, Li Q, Feng Z, et al. m6A reader YTHDF2 regulates LPS-induced inflammatory response. Int J Mol Sci. 2019;20(6). DOI:10.3390/ijms20061323
- Gokhale NS, McIntyre A, McFadden MJ, et al. N6-methyladenosine in flaviviridae viral RNA genomes regulates infection. Cell Host Microbe. 2016;20(5):654–665.
- Cohen J. HIV/AIDS. Dreams meet realities at AIDS conference. Science. 2012;337(6094):509–510.
- Lichinchi G, Gao S, Saletore Y, et al. Dynamics of the human and viral m(6)A RNA methylomes during HIV-1 infection of T cells. Nat Microbiol. 2016;1(16011). DOI:10.1038/nmicrobiol.2016.11
- Tirumuru N, Zhao BS, Lu W, et al. N(6)-methyladenosine of HIV-1 RNA regulates viral infection and HIV-1 Gag protein expression. Elife. 2016;5. DOI:10.7554/eLife.15528.
- Kennedy EM, Bogerd HP, Kornepati A, et al. Posttranscriptional m(6)A editing of HIV-1 mRNAs enhances viral gene expression. Cell Host Microbe. 2017;22(6):830.
- Kariko K, Buckstein M, Ni H, et al. Suppression of RNA recognition by toll-like receptors: the impact of nucleoside modification and the evolutionary origin of RNA. Immunity. 2005;23(2):165–175.
- Repunte-Canonigo V, Lefebvre C, George O, et al. Gene expression changes consistent with neuroAIDS and impaired working memory in HIV-1 transgenic rats. Mol Neurodegener. 2014;9(26). DOI:10.1186/1750-1326-9-26
- Sanna PP, Repunte-Canonigo V, Masliah E, et al. Gene expression patterns associated with neurological disease in human HIV infection. Plos One. 2017;12(4):e175316.
- Fleming AM, Nguyen N, Burrows CJ. Colocalization of m(6)A and G-quadruplex-forming sequences in viral RNA (HIV, Zika, Hepatitis B, and SV40) suggests topological control of adenosine N (6)-methylation. ACS Cent Sci. 2019;5(2):218–228.
- Krug RM, Morgan MA, Shatkin AJ. Influenza viral mRNA contains internal N6-methyladenosine and 5ʹ-terminal 7-methylguanosine in cap structures. J Virol. 1976;20(1):45–53.
- Narayan P, Ayers DF, Rottman FM, et al. Unequal distribution of N6-methyladenosine in influenza virus mRNAs. Mol Cell Biol. 1987;7(4):1572–1575.
- Courtney DG, Kennedy EM, Dumm RE, et al. Epitranscriptomic enhancement of influenza a virus gene expression and replication. Cell Host Microbe. 2017;22(3):377–386.
- Winkler R, Gillis E, Lasman L, et al. m(6)A modification controls the innate immune response to infection by targeting type I interferons. Nat Immunol. 2019;20(2):173–182.
- Durbin AF, Wang C, Marcotrigiano J, et al. RNAs containing modified nucleotides fail to trigger RIG-I conformational changes for innate immune signaling. Mbio. 2016;7(5). DOI:10.1128/mBio.00833-16
- Horner SM, Gale MJ. Regulation of hepatic innate immunity by hepatitis C virus. Nat Med. 2013;19(7):879–888.
- Villordo SM, Filomatori CV, Sanchez-Vargas I, et al. Dengue virus RNA structure specialization facilitates host adaptation. Plos Pathog. 2015;11(1):e1004604.
- Lichinchi G, Zhao BS, Wu Y, et al. Dynamics of human and viral RNA methylation during zika virus infection. Cell Host Microbe. 2016;20(5):666–673.
- Purushothaman P, Uppal T, Verma SC. Molecular biology of KSHV lytic reactivation. Viruses. 2015;7(1):116–153.
- Aneja KK, Yuan Y. Reactivation and lytic replication of Kaposi’s sarcoma-associated herpesvirus: an update. Front Microbiol. 2017;8(613). DOI:10.3389/fmicb.2017.00613
- Tan B, Gao SJ. The RNA Epitranscriptome of DNA Viruses. J Virol. 2018;92(22). DOI:10.1128/JVI.00696-18
- Hesser CR, Karijolich J, Dominissini D, et al. N6-methyladenosine modification and the YTHDF2 reader protein play cell type specific roles in lytic viral gene expression during Kaposi’s sarcoma-associated herpesvirus infection. Plos Pathog. 2018;14(4):e1006995.
- Ye F. RNA N(6)-adenosine methylation (m(6)A) steers epitranscriptomic control of herpesvirus replication. Inflamm Cell Signal. 2017;4:3.
- Baquero-Perez B, Antanaviciute A, Yonchev ID, et al. The Tudor SND1 protein is an m(6)A RNA reader essential for replication of Kaposi’s sarcoma-associated herpesvirus. Elife. 2019;8. DOI:10.7554/eLife.47261.
- Canaani D, Kahana C, Lavi S, et al. Identification and mapping of N6-methyladenosine containing sequences in simian virus 40 RNA. Nucleic Acids Res. 1979;6(8):2879–2899.
- Kahana C, Gidoni D, Canaani D, et al. Simian virus 40 early mRNA’s in lytically infected and transformed cells contain six 5ʹ-terminal caps. J Virol. 1981;37(1):7–16.
- Finkel D, Groner Y. Methylations of adenosine residues (m6A) in pre-mRNA are important for formation of late simian virus 40 mRNAs. Virol. 1983;131(2):409–425.
- Tsai K, Courtney DG, Cullen BR. Addition of m6A to SV40 late mRNAs enhances viral structural gene expression and replication. Plos Pathog. 2018;14(2):e1006919.
- Chen XP, Long X, Jia WL, et al. Viral integration drives multifocal HCC during the occult HBV infection. J Exp Clin Cancer Res. 2019;38(1):261.
- Imam H, Khan M, Gokhale NS, et al. N6-methyladenosine modification of hepatitis B virus RNA differentially regulates the viral life cycle. Proc Natl Acad Sci U S A. 2018;115(35):8829–8834.
- Imam H, Kim GW, Mir SA, et al. Interferon-stimulated gene 20 (ISG20) selectively degrades N6-methyladenosine modified hepatitis B virus transcripts. Plos Pathog. 2020;16(2):e1008338.
- Liu J, Eckert MA, Harada BT, et al. m(6)A mRNA methylation regulates AKT activity to promote the proliferation and tumorigenicity of endometrial cancer. Nat Cell Biol. 2018;20(9):1074–1083.
- Wang Q, Chen C, Ding Q, et al. METTL3-mediated m(6)A modification of HDGF mRNA promotes gastric cancer progression and has prognostic significance. Gut. 2019. DOI:10.1136/gutjnl-2019-319639
- Huang H, Weng H, Chen J. m(6)a modification in coding and non-coding RNAs: roles and therapeutic implications in cancer. Cancer Cell. 2020;37(3):270–288.
- Su R, Dong L, Li C, et al. R-2HG exhibits anti-tumor activity by targeting FTO/m(6)A/MYC/CEBPA signaling. Cell. 2018;172(90–105):1–2.
- Su R, Dong L, Li Y, et al. Targeting FTO suppresses cancer stem cell maintenance and immune evasion. Cancer Cell. 2020;38(1):79–96.
- Chen YG, Chen R, Ahmad S, et al. N6-methyladenosine modification controls circular RNA immunity. Mol Cell. 2019;76(1):96–109.
- Gubin MM, Artyomov MN, Mardis ER, et al. Tumor neoantigens: building a framework for personalized cancer immunotherapy. J Clin Invest. 2015;125(9):3413–3421.
- Li N, Kang Y, Wang L, et al. ALKBH5 regulates anti-PD-1 therapy response by modulating lactate and suppressive immune cell accumulation in tumor microenvironment. Proc Natl Acad Sci U S A. 2020;117(33):20159–20170.
- Shen C, Sheng Y, Zhu AC, et al. RNA demethylase ALKBH5 selectively promotes tumorigenesis and cancer stem cell self-renewal in acute myeloid leukemia. Cell Stem Cell. 2020;27(1):64–80.
- Xu F, Zhang H, Chen J, et al. Immune signature of T follicular helper cells predicts clinical prognostic and therapeutic impact in lung squamous cell carcinoma. Int Immunopharmacol. 2020;81(105932). DOI:10.1016/j.intimp.2019.105932
- Motorin Y, Helm M. Methods for RNA modification mapping using deep sequencing: established and new emerging technologies. Genes (Basel). 2019;10(1). DOI:10.3390/genes10010035
- Bader JP, Brown NR, Chiang PK, et al. 3-Deazaadenosine, an inhibitor of adenosylhomocysteine hydrolase, inhibits reproduction of Rous sarcoma virus and transformation of chick embryo cells. Virol. 1978;89(2):494–505.