ABSTRACT
Sirtuin1 is a nutrient-sensitive class III histone deacetylase which is a well-known regulator of organismal lifespan. It has been extensively studied for its role in metabolic regulation as well. Along with its involvement in ageing and metabolism, Sirtuin1 directly deacetylates many critical proteins controlling cardiovascular pathophysiology. Studies using conditional expression and deletion of Sirtuin1 have revealed that it functions in a highly tissue/organ-specific manner. In the vasculature, Sirtuin1 controls endothelial homoeostasis by governing the expression of inflammatory mediators, oxidants and essential transcription factors. Adding to this complexity, Sirtuin1 expression and/or function is also governed by some of these target proteins. Therefore, the importance of better understanding the organ and tissue specificity of Sirtuin1 is highly desirable. Considering the huge volume of research done in this field, this review focuses on Sirtuin1 targets regulating vascular endothelial function. Here, we summarize the discovery of Sirtuin1 as a transcription controller and the further identification of direct target proteins involved in the vascular physiology. Overall, this review presents a holistic picture of the complex cross-talk involved in the molecular regulation of vascular physiology by Sirtuin1.
Introduction
Sirtuin1, a nutrient-sensitive deacetylase, is one of the most-studied members of the class III family of histone deacetylases (HDACs). Sirtuin1 is highly conserved in all living organisms including bacteria, archaea, and eukaryotes [Citation1]. The deacetylase activity of Sirtuins (Sirtuin1–7) is dependent on nicotinamide adenine dinucleotide (NAD+) availability, which distinguishes Sirtuins from other classes of HDACs. Also, these isoforms significantly differ in their sub-cellular localization, targets, and function ().
Table 1. Isoforms of the Sirtuin family with their subcellular localization, catalytic activities, substrates, and functions.
Sirtuin1 is the closest mammalian ortholog of Silent Information Regulator (SIR2) protein, a longevity factor in lower eukaryotes such as C. Elegans. The association of SIR1 (an ortholog of SIR in Sachharomyces cerevisiae) with longevity in primordial organisms has spurred studies to establish its role in extending lifespan in vertebrates. These investigations sought to identify the mechanisms by which Sirtuin1 increases lifespan and have accelerated the discovery of Sirtuin1 activators and novel functional targets. Although the prolongation of lifespan by Sirtuin1 is not well established, its role in improving overall health is unquestionable. Sirtuin1 is associated with many cellular processes including metabolism, apoptosis, mitochondrial biogenesis, oxidative stress, inflammation, and autophagy. Many of these processes are actively involved in cardiovascular diseases. Here, we will summarize the progress made in understanding Sirtuin1 function with a specific emphasis on vascular health.
Background
Chromatin silencing and longevity
The concept that changes in DNA organization regulate longevity originated from the screening of genes in a stress-resistant strain of yeast which also had a longer life span. Kennedy et al. (1995) reported that mutations in 4 genes called ‘youth mutants’ (-UTH1-UTH4) are associated with resistance to cold stress and prolonged life span [Citation2]. Interestingly, out of these four genes, UTH2 was identical to SIR (Silent information repressor) 4, which was known to silence regions of yeast chromosomes [Citation3]. The SIRs (SIR2, SIR3 and SIR4) induce chromatin silencing by deacetylating histones, and thus, SIR increases life span via transcriptional silencing through histone deacetylation [Citation4]. This formed the basis for the idea that chromatin silencing could mediate an increase in life span. In 1999, Kaeberlein et al. reported that SIR2 functions independent of the SIR2/3/4 complex [Citation5] and overexpression of SIR2 further increases the life span of yeast. This function of SIR2 was also noted in Caenorhabditis elegans, where transgenic expression of SIR-2.1 (the C. elegans homolog of yeast SIR2) produced a 50% increase in its life span [Citation6]. Thus, chromatin silencing by SIR2 was established as the molecular basis for the increased lifespan in yeast and worm by this Sirtuin1 homolog [Citation7].
Sirtuins, a NAD+-dependent unique class of histone deacetylase
The finding that SIR2 can extend life span led to aggressive research in this field investigating the histone deacetylase (HDAC) function of Sir2. Three independent groups of investigators reported that the deacetylase function of SIR2 is dependent on NAD (Nicotinamide adenine dinucleotide). It was reported that the HDAC function of SIR2 is dependent on the acetylated histone and NAD+ [Citation8] and that there is a stoichiometric relationship between the deacetylase activity of Sirtuin1 and NAD+ [Citation9]. Further, deletion of the NPT1 gene (Nicotinate phosphoribosyltransferase, responsible for NAD+ synthesis) in yeast had an effect similar to that of SIR2 deletion [Citation10]. Another exhaustive study identified the specific lysines targeted by SIR2 for histone deacetylation [Citation11]. It is reported that the NAD+-dependent deacetylation of histone H3 at lysines 9 and 14, as well as of histone H4 at lysine 16, mediates SIR2-induced chromatin silencing. Another important finding by the later study was the identification of a mammalian homolog of SIR2 called mSir2α. Recombinant mSir2α could deacetylate the acetylated histone peptide in a manner similar to SIR2, thus indicating that transcriptional silencing by SIR2 may also function in mammals. The NAD+-dependence of SIR2 separated it into a different class of HDACs, the Class III HDACs, also known as Sirtuins [Citation12]. Sirtuins were identified as molecular mediators of caloric restriction-induced prolongation of lifespan. Many studies have investigated whether Sirtuin1 could prolong lifespan and the outcomes have been promising [Citation13–15]. Interestingly, transgenic mice overexpressing Sirtuin1 showed a phenotype similar to the calorie restricted mice and had improved glucose homoeostasis and lipid metabolism [Citation13]. This led to the two-way approach to target Sirtuin1: first to identify novel target proteins of Sirtuin1 deacetylase to expand the understanding of the physiological roles of Sirtuin1, and second to develop tools to activate the deacetylase function of Sirtuin1.
Non-histone targets of sirtuin1
The ability of Sirtuin1 to regulate its life span formed the basis for future studies identifying non-histone targets of Sirtuin1. Sirtuins are also present outside the nucleus, in the cytoplasm, mitochondria and the cell membrane [Citation16]. Therefore, the possibility of non-histone proteins mediating the effects of Sirtuin1 was explored. Since Sirtuin1 was primarily known to regulate transcription, one obvious target was transcription factors. We will discuss the important molecular targets of Sirtuin1 and the Sirtuin1 mediated regulation of vascular function ().
Figure 1. Molecular targets of Sirtuin1. SIRT1 deacetylates multiple transcription factors as well as proteins involved in the control of cellular physiology. Deacetylation of transcription factor affects the protein expression directly, while direct acetylation of proteins changes their function, localization, or stability. PGC-1⍺: Peroxisome proliferator-activated receptor gamma coactivator 1-alpha, PPAR-γ: Peroxisome proliferator-activated receptor γ, ER-β: Oestrogen receptor-beta, SUV39H1: Suppressor Of Variegation 3–9 Homolog 1, KU70: DNA-PK Ku70 subunit, APE: Apurinic/apyrimidinic endonuclease-1, TGF-β: Transforming growth factor β, Smad7: Mothers against decapentaplegic homolog 7, HIPK2: Homeodomain interacting protein kinase-2, LXRs: Liver X receptors, NF-kβ: Nuclear factor-ƙB, NO: Nitric oxide, eNOS: Endothelial nitric oxide, FOXO: Forkhead box class O family of transcription factor, ROS: Reactive oxygen species.
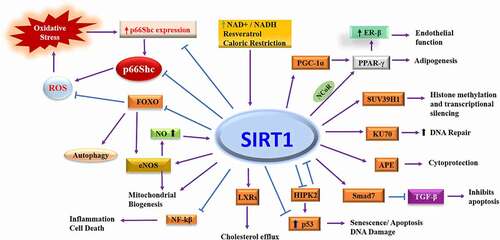
p53
Sirtuin1 protects against DNA damage [Citation17]. It is known that the tumour suppressor p53 is activated in response to DNA damage which subsequently promotes p53 acetylation by p300 [Citation18]. Interestingly, and almost simultaneously, two independent groups of investigators reported that Sirtuin1 deacetylates p53 and represses its ability to promote apoptosis in response to DNA damage and oxidative stress [Citation19,Citation20], and later that lysine 379 (equivalent to human lysine 382) is the target of Sirtuin1 [Citation21]. In addition, these studies identified p53 as the first non-histone target of Sirtuin1, and this deacetylation of p53 by Siruin1 is regarded as a standard test to determine the deacetylase activity of Sirtuin1. With the discovery of transcription factors as targets of Sirtuin1 to regulate cell survival, and the dependence of this regulation on nutrient availability, researchers focused on other transcription factors regulating energy homoeostasis.
Foxo
Forkhead proteins (Foxos- Forkhead box class O family of transcription factor) are transcription factors which regulate cell survival and longevity. The binding of growth factor activates receptor tyrosine kinase and protein kinase B (Akt) which phosphorylates and suppresses Foxos. Conversely, disrupting the PI3K (Phosphoinositide 3-kinase)-Akt pathway activates Foxos and prolongs life-span [Citation22,Citation23]. This dual regulation by Foxo was later explained as Foxo being sensitive to the degree of cellular stress and damage which decides the cell’s fate for DNA repair or apoptosis [Citation24]. The dependence of Sirtuin1 on DAF-16 for longevity and the identification of p53 as a direct target for Sirtuin1 deacetylation raised the question whether Foxos, the mammalian orthologs of DAF-16, are a target of Sirtuin1 deacetylase. The first study by Motta et al. (2004) reported that Sirtuin1 deacetylates the acetyl transferase P300 as well as Foxo3 and represses the transcription of Foxo-regulated genes [Citation25]. The regulation of Foxo3 and p53 by Sirtuin1 indicated that Foxo3 and p53 may interact mutually, which was later proven to be true [Citation26]. It was also reported that Sirtuin1 differentially affects Foxo3 function; it inhibits Foxo3-induced apoptosis but facilitates DNA repair and cell cycle arrest. The accumulating evidence that Sirtuin1, p53, and Foxo3 may have a role in regulating longevity was answered in a study investigating the relationship among these three proteins which determined that Sirtuin1 expression is regulated by p53 [Citation27]. Knockdown of p53 increases Sirtuin1 expression under basal conditions. However, under a starved condition Foxo3a binds to nuclear p53 and increases Sirtuin1 transcription.
Subsequently, Foxo4, another member of the Foxo family, was identified as a target of Sirtuin1 deacetylation [Citation28]. Foxo4 is acetylated by p300 under oxidative stress and is deacetylated by Sirtuin1. Acetylation of Foxo4, unlike Foxo3, inhibits its transcriptional activity and this offers resistance against oxidative stress [Citation28,Citation29].
Nuclear factor kappa-β (NF-kβ)
Continuing with the identification of transcription factors as targets of Sirtuin1 deacetylase, another protein, nuclear factor-kappa B (NF-κB), was examined for regulation by Sirtuin1. NF-κB was known to be acetylated by p300 and deacetylated by HDAC3 [Citation30] and HDAC2 [Citation31]. It was reported that Sirtuin1 deacetylates the RelA/p65 subunit of NF-κB at lysine 310 which inhibits its activity and promotes TNF-alpha induced cell death [Citation32]. This was the first study to report that Sirtuin1 can also promote cell death, suggesting that the regulation of cell survival by Sirtuin1 is dependent on the activation of specific signalling pathways. Later studies also reported a negative regulation of Sirtuin1 by NF-κB via the latter’s ability to promote oxidative stress, inflammation and the level of miR-34a, which degrades Sirtuin1 [Citation33].
Homeodomain-interacting protein kinase 2 (HIPK2)
HIPK2 is a serine/threonine kinase that regulates DNA damage responses and functions as a tumour suppressor by activating p53-mediated apoptosis in cancer cells [Citation34]. Investigating the possible regulation of HIPK2, Hwang et al. (2013) reported that Sirtuin1 deacetylates HIPK2 and thereby promotes the ubiquitination and proteasomal degradation of HIPK2 and that inhibition of Sirtuin1 activity increases the protein level of HIPK2 [Citation35]. Interestingly, in response to the DNA damage evoked by anticancer agents, HIPK2 physically binds to Sirtuin1 and inhibits its deacetylase function via phosphorylation of S682 [Citation36], thus suggesting the existence of a negative feedback loop similar to that seen between NF-κB and Sirtuin1.
Peroxisome proliferator-activated receptor- γ (PPAR-γ) and PPAR- γ Coactivator (PGC)-1α
Reduced white adipose tissue deposition is associated with an increased life span in mice [Citation37]. The nuclear receptor PPAR-γ promotes adipogenesis [Citation38]. There is a positive correlation between the longevity resulting from reduced adiposity and Sirtuin1 expression levels [Citation7] which indicates a possibly negative correlation between Sirtuin1 and PPAR-γ. Sirtuin1 co-represses PPAR-γ by binding to the NCoR (nuclear receptor corepressor), a co-factor of PPAR-γ in a nutrient-dependent relationship [Citation39]. It has been proposed that Sirtuin1 physically docks to NCoR and thereby represses the transcription activator function of PPAR-γ on its targets aP2 (acid-binding protein) and Pparg (PPAR-γ). Later, it was determined that the co-repressor effect of Sirtuin1 on PPAR-γ is due to its strong physical interaction with and deacetylation of PPAR-γ [Citation40].
The PPAR Gamma Coactivator (PGC)-1α is a thermosensitive transcriptional regulator of PPAR-γ [Citation41]. It controls hepatic glucose production and its expression increases under fasting conditions [Citation42]. The transcriptional control of PGC-1α by nutrient availability, the known regulation of Sirtuin1 by nutrient availability, and the recently identified regulation of PPAR-γ by Sirtuin1 indicated a strong probability of PGC-1α being the target of Sirtuin1 deacetylation. A year later, PGC-1α was identified as a target of Sirtuin1 [Citation43,Citation44]. Sirtuin1 deacetylates PGC-1α at multiple lysines. Sirtuin1, PGC-1α, and HNF4-α (hepatocyte nuclear factor-4-alpha) physically interact and PGC-1α and Sirtuin1 co-regulate the extent of gluconeogenesis and glycolysis.
Ku70 and apurinic/apyrimidinic endonuclease-1 (APE1)
During routine cell division and maintenance, DNA is damaged, and the internal homoeostatic machinery repairs this damage using enzymes like Ku70 and apurinic/apyrimidinic endonuclease-1 (APE1). Ku70 facilitates DNA repair and acetylation of Ku70 impairs Ku70 – mediated inhibition of Bax-induced apoptosis [Citation45]. Supporting the involvement of lysine acetylation in the DNA repair function of Ku70, it was reported that Sirtuin1 physically interacts with and deacetylates Ku70, thereby increasing the DNA repair response to irradiation [Citation46].
Sirtuin1 also targets APE1, a multifunctional protein involved in the repair of single-strand DNA breaks [Citation47]. APE1 is acetylated in response to hydrogen peroxide and binds to Sirtuin1, which stimulates APE1 activity thereby offering cytoprotection. This interaction also works in a feedforward loop as APE1 promotes endothelial Sirtuin1 activity by maintaining the cysteine-thiols 371 and 374 in reduced form [Citation48].
SUV39H1, smad7 and LXR
In addition to direct transcriptional control by Sirtuin1, the possibility of Sirtuin1 indirectly regulating cellular transcription was also evaluated. HDACs deacetylate histones which removes the positive charge and represses the transcriptional process. Another set of enzymes, histone methyl transferases, adds methyl groups to histones which represses transcription. Deacetylation of histones by Sirtuin1 is associated with the loss of methylation [Citation49]. Vaquero et al. (2007) identified SUV39H1 (Suppressor of Variegation 3–9 Homolog 1), a methyl transferase, as the direct target of Sirtuin1 regulating histone methylation [Citation50]. It was reported that Sirtuin1-mediated deacetylation increases the half-life of SUV39H1 by preventing its ubiquitination and degradation, thereby promoting histone methylation and silencing transcription [Citation50].
Continuing with the Sirtuin1-mediated deacetylation of nuclear proteins and regulation of cell survival, smad7, one of the mediators of transforming growth factor β (TGF- β) signalling involved in apoptosis, cell cycle, differentiation, and extracellular matrix accumulation was evaluated as Sirtuin1 target. Smad7 (Mothers against decapentaplegic homolog 7), which was known to be acetylated by p300 [Citation51], was identified as a direct target of Sirtuin1 [Citation52]. Sirtuin1 inhibits TGF-β signalling by reducing smad7 expression via its deacetylation and subsequent ubiquitination and proteasomal degradation.
Transcription factors regulating key physiological processes were continuously evaluated as potential targets of Sirtuin1 deacetylation and many of these were metabolic regulators. The first evidence of direct regulation of a metabolic enzyme by Sirtuin1 deacetylase was presented by Starai et al. [Citation53]. This study demonstrated that in Salmonella enterica, acetyl co-a synthetase (Acs) is acetylated at lysine 609 which blocks its synthetase activity. Deacetylation of Acs by CobB, a protein of Sirtuin class, activated the enzymatic activity of Acs. These findings were corroborated by studies of mammalian Acs. Hallows et al. (2006), reported that the acetylation of the conserved lysine residue in mammalian AceCS1 inactivates its synthetase activity, while Sirtuin1-catalysed deacetylation reactivates it [Citation54]. This was a milestone in terms of extending the possible roles of Sirtuin1 to include regulating metabolic enzymes and expanding our knowledge about the effect of Sirtuin1-mediated regulation of cellular, and specifically metabolic, functions.
Another essential non-histone target of Sirtuin1 deacetylation is liver X receptor (LXR) proteins. LXRs form heterodimers with retinoid X receptors and function as a sterol sensor to regulate cholesterol metabolism and hepatic fat metabolism [Citation55]. LXRs enhance reverse cholesterol transport by stimulating the expression of the ATP-binding cassette transporter A1 (ABCA1), which mediates the efflux of cholesterol from peripheral tissues to apolipoprotein A in the blood to form HDL. Sirtuin1 binds and deacetylates LXRα and LXRβ at lysines 432 and 433, respectively, which are in close proximity to the LXR’s ligand-dependent activation domain which promotes the cellular cholesterol efflux [Citation56].
Following this publication, many reports described that the effect of Sirtuin1 is not exclusively the transcriptional control of cellular processes. Supporting this notion, Tanno et al., (2007), reported dynamic translocation of Sirtuin1 between the cytoplasm and nucleus and identified the presence of a nuclear localisation and nuclear export signals at the N-terminus of Sirtuin1 [Citation57].
Sirtuin1 in the regulation of vascular function
These studies revolutionized the field of Sirtuin1 research with respect to the role of Sirtuin1 in controlling physiological homoeostasis by regulating both cytoplasmic and nuclear proteins (). The role of Sirtuin1 in the regulation of endothelial function was not previously known, although HDACs were already known to control vascular function [Citation58].
Table 2. Important molecular targets of Sirtuin1 and their effects.
As discussed earlier, the caloric restriction regulates the expression of Sirtuin1 and the level of endothelial nitric oxide (eNOS) [Citation59]. This suggested the possibility that Sirtuin1 and eNOS could interact directly and thereby allow Sirtuin1 to regulate vascular endothelial function. The study by Mattagajasingh et al., (2007) was the first to demonstrate the deacetylation of eNOS by Sirtuin1 and its role in the regulation of vascular endothelial function [Citation60]. It is shown that caloric restriction decreases the acetylation of eNOS and established that Sirtuin1 physically interacts with eNOS and deacetylates it. It is also suggested that lysines 496 and 506 are indispensable for the Sirtuin1 regulated nitric oxide (NO) production by eNOS. The identification of Sirtuin1-mediated deacetylation of eNOS led to further investigations regarding whether this regulation is altered in the settings of systemic pathologies. Another study reported that NO also regulates Sirtuin1, suggesting a positively feed-forward regulation of the Sirtuin1-eNOS axis in a NO-dependent manner [Citation61]. This corroborated an earlier report showing that mice with a genetic deletion of e-NOS exhibit diminished effects of caloric restriction on the increase in Sirtuin1 expression in white adipose tissue [Citation59]. Supporting the physiological significance of Sirtuin1 in the regulation of endothelial function, a later study presented evidence that vascular homoeostasis is maintained via the Sirtuin1-eNOS axis [Citation62]. This study found that the laminar flow-induced activation of AMP-activated protein kinase and Sirtuin1 increases eNOS-mediated NO production. Subsequent studies have identified multiple mechanisms via which Sirtuin1 regulates vascular endothelial function, including its regulation by mir-34a [Citation63,Citation64] and mir-204 [Citation65] and the regulation of endoplasmic reticulum stress in endothelial cells via mir-204 by Sirtuin1 [Citation66,Citation67]. We will focus this review on the Sirtuin1 targeted proteins regulating vascular function ().
Figure 2. Regulation of endothelial function by Sirtuin1. SIRT1 mediated deacetylation reduces oxidative stress by decreasing the level of reactive oxygen species and thereby increases nitric oxide availability. SIRT1 also regulates proteins which control inflammation and cell cycle which improves endothelial health. FOXO1: Forkhead box class O family of transcription factor, ER-β: Oestrogen receptor-beta, NLRP3: NLR family pyrin domain containing 3, ROS: Reactive oxygen species, LKB1: liver kinase β1, TGF-β: Transforming growth factor β, NO: Nitric oxide, eNOS: Endothelial nitric oxide, PAI-1: Plasminogen activator inhibitor 1.
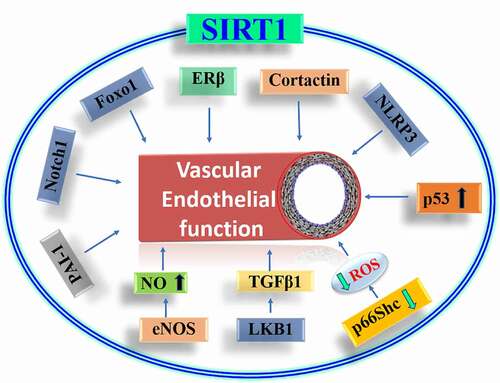
Endothelial dysfunction due to ageing
The role of Sirtuin1 in ageing-induced endothelial dysfunction was first reported by Donato et al. 2011 [Citation68]. This study showed that aged mice have impaired endothelial function, a reduced level of Sirtuin1 and serine 1177 phosphorylation of eNOS. Also, in healthy individuals, the endothelial Sirtuin1 level is positively correlated with the acetylcholine-induced endothelium-dependent vasodilation. Similar trends were noted for the Sirtuin1 level in vascular smooth muscle cells and its association with ageing where the Sirtuin1 level negatively correlated with age and atherosclerotic lesions [Citation69].
Another mechanism of Sirtuin1-mediated regulation of vascular function was presented by Kong et al. [2016, Citation70]. Oestrogen receptors are known to have a protective effect on the vasculature [Citation71]. It was reported that, in aged mice, expression of oestrogen receptor-beta (ERβ) is reduced in the vasculature, as is the deacetylase activity of Sirtuin1 [Citation70]. This study also discovered that the deacetylase function of Sirtuin1 was impaired due to reduced activity of casein kinase and subsequent phosphorylation of Sirtuin1. It is shown that rescuing Sirtuin1 phosphorylation represses ERβ expression by facilitating the binding of Sirtuin1-PPARγ/RXR(retinoid X receptor)-p300 on the PPARγ response element-binding site in the promoter region of Erβ, thereby regulating the transcriptional level of ERβ.
Sirtuin1 prevents the senescence in human endothelial cells by reducing plasminogen activator inhibitor 1 (PAI-1) and increasing e-NOS [Citation72] and KLF2 (Kruppel Like Factor 2) [Citation73] expression. It was shown that endothelium-specific transgenic overexpression of Sirtuin1 in mice reduces the expression of PAI1 [Citation74]. This study also found that Sirtuin1 binds to the PAI-1 promoter and deacetylates histone H4 at lysine 16 (H4K16) in the PAI-1 promoter region. This Sirtuin1-mediated epigenetic inhibition of PAI-1 expression also protected against vascular endothelial senescence.
Another mechanism proposed for Sirtuin1-mediated protection against endothelial senescence is via liver kinase β1 (LKB1). In porcine aortic endothelial cells, Sirtuin1 deacetylates LKB1, thereby preventing the LKB1-dependent activation of AMP-kinase and preventing senescence [Citation75]. In a subsequent study it was reported that acetylated LKB1 binds and activates the TGFβ1 promoter which is known to mediate many vascular pathologies [Citation76].
A recent study investigating the significance of Sirtuin1-Notch1 signalling (also involved in angiogenesis discussed later) in the regulation of vascular function reported that endothelium-specific transgenic overexpression of Sirtuin1 protects against the ageing-induced increase in vascular smooth muscle contraction by inhibiting the Notch1 signalling [Citation77]. Endothelium-specific expression of Sirtuin1 also rescued the ageing-induced endothelial dysfunction and hypercontractility of aortas. Interestingly, the effect of Sirtuin1 on vascular contractility was independent of nitric oxide signalling. Endothelium-specific overexpression of Sirtuin1 increased the stability and expression of soluble guanylyl cyclase in smooth muscle cells via Notch1 signalling.
Angiogenesis
Sirtuin1 regulates angiogenesis via Foxo1 [Citation78] and endothelium-specific deletion of Sirtuin1 impairs angiogenesis and induces diastolic dysfunction in pressure overload [Citation79]. In endothelial cells, Notch1 regulates angiogenesis. Acetylation in the intracellular domain of Notch1 is regulated by Sirtuin1. Sirtuin1 deacetylates the intracellular domain of Notch1 and inhibits Notch1 signalling [Citation80]. In zebrafish and mice, inhibition of Sirtuin1 in the endothelium impairs angiogenesis by increasing the stability of the Notch1 intracellular domain (NICD).
Antioxidant effect of sirtuin1
Using a rat model of vascular inflammation and oxidative stress, Csiszar et al., (2008) showed that Sirtuin1 mediates the anti-inflammatory and antioxidant effects of resveratrol on vasculature [Citation81] which was later shown to be mediated via Sirtuin1-induced eNOS deacetylation [Citation82]. In addition, administering resveratrol to diabetic mice rescued the decline in mitochondrial DNA content and mitochondrial biogenesis factors in endothelial cells. The anti-inflammatory and antioxidant effects of Sirtuin1 were also evident in human coronary artery endothelial cells [Citation83]. Another study by the same group reported that the activation of Sirtuin1 by resveratrol induces mitochondrial biogenesis in coronary arterial endothelial cells and is dependent on nitric oxide availability [Citation84]. Corroborating the antioxidant effects of Sirtuin1, we identified a novel mechanism by which Sirtuin1 regulates vascular oxidative stress. The adaptor protein p66Shc produces ROS and regulates vascular endothelial function in various pathologies including ageing, hyperglycaemia, and dyslipidemia. Interestingly, there is an inverse relationship between the expression level of p66Shc and Sirtuin1 in diseased vasculature. Knowing that in addition to nuclear proteins like histones, Sirtuin1 also targets many cytosolic proteins for deacetylation, we asked whether p66Shc is a direct target of Sirtuin1 deacetylation. Investigating the possible interaction of p66Shc and Sirtuin1 proteins, we identified a functional relationship between p66Shc and Sirtuin1 [Citation85]. Sirtuin1 directly binds to p66Shc and deacetylates it. The acetylation at lysine 81 of p66Shc is increased in hyperglycaemia and is necessary for the serine 36 phosphorylation, ROS production and mitochondrial translocation of p66Shc. Furthermore, we found that Sirtuin1 deacetylates the redox protein p66Shc which prevents the oxidative phosphorylation of p66Shc and subsequent ROS production.
Sirtuin1 and diabetic vascular dysfunction
With growing evidence of Sirtuin1 as a key metabolic enzyme [Citation86], the metabolic regulation of endothelial cell function was also evident [Citation87]. Ormia et al., (2009) reported that hyperglycaemia downregulates Sirtuin1 in vascular endothelial cells and that Sirtuin1 protects against hyperglycaemia-induced endothelial senescence, inflammation, and dysfunction by deacetylating p53 [Citation88]. Also, endothelial-specific transgenic expression of Sirtuin1 protects against diabetes-induced senescence in the endothelium [Citation89].
Zhou et al., (2011) discovered an epigenetic regulation of p66Shc expression by Sirtuin1. Sirtuin1 binds to the p66Shc promoter (508 bp-250 bp) and represses p66Shc expression by deacetylating lysine 9 of histone H3 [Citation90]. The increased level of p66Shc mRNA by high glucose and oxidized low-density lipoproteins were inhibited by the overexpression of Sirtuin1. In addition, the endothelium-specific transgenic overexpression of Sirtuin1 protected against the diabetes-induced increase in mRNA and protein levels of p66Shc compared to wild-type mice. Investigating the pathophysiological significance of Situin1 and p66Shc interaction, we reported that hyperglycaemia increases Sirtuin1-regulated p66ShcK81 acetylation [Citation85]. Transgenic mice overexpressing endothelium-specific p66ShcK81R (an acetylation-deficient mutant of p66ShcK81) were protected against hyperglycaemia-induced endothelial dysfunction and oxidative damage. In addition, endogenous mutation of p66Shc lysine 81 (p66ShcK81R knockin mice) produced a similar phenotype, thus supporting the concept that K81 modification of p66Shc drives the oxidative function of the protein in the vasculature. Remarkably, these p66ShcK81R knockin mice were also protected against diabetic downregulation of vascular Sirtuin1. This corroborated the findings of our earlier study showing that ROS produced by p66Shc downregulates the level of Sirtuin1 via upregulation of miR34a [Citation64].
Sirtuin1 and atherosclerosis
Sirtuin1 regulates lipid accumulation [Citation55] and NO level [Citation60]. In clinical studies (small sample size), an association between polymorphism in Sirtuin1 and coronary artery disease has also been noted [Citation91–93]. Using a mouse model having endothelium-specific transgenic overexpression of Sirtuin1, Zhang et al., (2008) showed that overexpression of Sirtuin1 in the endothelium protects against high fat diet-induced impairment of vascular endothelial dysfunction and reduces atherosclerotic plaque formation throughout the aortic length in ApoE−/-(mice having genetic deletion of Apolipoprotein E gene) mice on high-fat diet [Citation94]. This was also corroborated by another study showing that heterozygous deletion of Sirtuin1 promotes endothelial activation in ApoE−/- mice without affecting endothelial function [Citation95]. Cleavage of Sirtuin1 by capase1 promotes endothelial cell activation in ApoE−/- mice [Citation96]. In smooth muscle cells, resveratrol downregulated angiotension II type I receptors [Citation97] and prevented the angiotensin II–induced hypertrophy via Sirtuin1 [Citation98].
The role of Sirtuin1 in smooth muscle cells was investigated in transgenic mice with smooth muscle cell-specific expression of human Sirtuin1 [Citation99]. These mice were protected against neointima formation in injured carotid arteries, suggesting the antiproliferative function of Sirtuin1 on vascular smooth muscle cells which may be involved in the atheroprotective effect of Sirtuin1. Using an alternative approach, Gorenne et al., (2013) performed a loss of function study to evaluate the role of Sirtuin1 in smooth muscle cells. Supporting the earlier findings, smooth muscle cell-specific deletion of Sirtuin1 increased DNA damage, apoptosis, atherosclerosis, and degeneration of the medial layer [Citation100]. Using transgenic mouse lines with either smooth muscle-specific Sirtuin1 gain or loss of function, Fry et al., (2016) showed that Sirtuin1 protects against diet-induced aortic stiffness via its anti-inflammatory and antioxidant effects [Citation101]. The protective role of Sirtuin1 in vascular smooth muscle cells was also noted in abdominal aortic aneurysm (AAA). Transgenic overexpression of Sirtuin1 in smooth muscle cells abrogated AAA induced by angiotensin II and calcium chloride [Citation102]. A recent report showed that Sirtuin1 also prevents vascular calcification by regulating RUNX2 (Runt-related transcription factor 2) [Citation103]. Together, these studies established that Sirtuin1 maintains a healthy vasculature by regulating both vascular endothelial and smooth muscle cells.
In addition to the above studies showing an imperative role of Sirtuin1 in regulating vascular health and function, another mechanism for the Sirtuin1-mediated control of vascular health has also been reported. Cortactin is a ubiquitously expressed protein [Citation104]. Genetic deletion of cortactin in mice showed that this protein is essential for maintaining a functional endothelial barrier [Citation105]. Flow-induced shear stress regulates Sirtuin1 expression and activity [Citation62], and Sirtuin1 deacetylates cortactin [Citation106]. Later, Shentu et al., 2016 reported that Sirtuin1-mediated deacetylation of cortactin facilitates its translocation to the endothelial cell periphery and thereby facilitates nitric oxide production [Citation107]. The physiological significance of cortactin-mediated intracellular trafficking of eNOS is evident in the increase in atherosclerotic lesions in ApoE−/- mice with reduced cortactin levels.
Atherosclerosis also involves the activation of inflammatory pathways in vascular endothelium which are mediated via the formation of inflammasomes. Endothelial cells exposed to LPS (lipopolysaccharide) show an activation of caspase via inflammasome activation. Sirtuin1 prevents the upregulation of NLRP3 inflammasomes and protects endothelial cells against LPS- and ATP-initiated inflammation [Citation108] and the secretion of IL-1β [Citation109].
Pharmacological tools to manipulate sirtuin1
Sirtuins are ubiquitously expressed in adult and foetal human tissues [Citation110]. The discovery that Sirtuin1 bridges the caloric restriction-induced prolongation of lifespan drew great attention from academic scientists and entrepreneurs interested in developing tools to enhance Sirtuin1 function. The quest to discover small-molecule Sirtuin activators (STACs) identified many plant secondary metabolites (polyphenols). Among them, resveratrol, a molecule found in red wine, showed the maximum potential to increase the deacetylase function of Sirtuin1 [Citation111]. Based on the chemical structure of resveratrol and other natural products, many synthetic analogs were developed to regulate Sirtuin1 function (recently reviewed by Bonkowski and Sinclair, 2016) [Citation112,Citation113]. The search for better STACs continued, but the real understanding of how STACs enhance Sirtuin1 enzymatic activity came from the crystallographic studies of Sirtuin1 interaction with STACs. The binding of STACs to the STACs binding domain (SBD) of Sirtuin1 inverts the SBD at the site of interaction between the substrate and the catalytic domain. This facilitates the activation of Sirtuin1 deacetylase function and is termed the ‘bend at the elbow’ model [Citation114]. Studies investigating resveratrol as a Sirtuin1 activator showed that it could elicit many of the physiological changes reported to be associated with caloric restriction [Citation115,Citation116]. However, clinical studies with resveratrol provided mixed results in terms of efficacy [Citation116–122]. This discrepancy in the clinical efficacy of resveratrol raised several questions, including whether resveratrol is effective in higher animals and if it works only in a specific subset of a population. The few explanations offered for this variability are based on the possibility of altered bioavailability of resveratrol. Due to its hydrophobic nature, the assimilation and distribution of resveratrol may vary based on the formulation and route of administration. However, the beneficial effects of resveratrol in lower animals motivated the development of STACs with higher potency and improved biophysical properties. The compound SRT2104, with much higher potency, has entered into phase II clinical studies; however, the unfavourable pharmacokinetics of the drug remains a challenge to overcome [Citation123–125].
Another approach to promote deacetylation by Sirtuins is to raise the level of NAD+ or its precursors. Nicotinamide riboside (NR) is a recently identified physiological precursor of NAD+ having the ability to increase NAD+ levels in mouse and humans. The administration of NR extended the life span in mice [Citation126]. Subsequent study showed that NR promotes Sirtuin1 dependent oxidative phosphorylation and thereby protects against diet-induced obesity [Citation127]. Also, NR protects against diabetes associated glucose intolerance and peripheral neuropathy [Citation128] and protected against non-alcoholic fatty liver disease [Citation129]. Unlike the STACs, NR does not have the limitation of bioavailability. It is orally bioavailable in humans and mice [Citation130]. NR has also been evaluated for its beneficial effects on heart [Citation131]. Oral supplementation of NR protected against the development of dilated cardiomyopathy in mice [Citation132]. However, the vascular effect of NR has not been well investigated, although it is known that the nicotinamide mononucleotide (an intermediate of NAD+) rescues the ageing-induced vascular dysfunction and oxidative stress [Citation133]. A small clinical study showed that NR reduces systolic blood pressure and aortic stiffness [Citation134]. Future studies are required to determine whether these Sirtuin1 activators can mimic the beneficial effects of Sirtuin1 on vascular function.
Conclusion
Sirtuin1 is master regulator of cellular and organismal physiology. Experimental evidences strongly suggest that promoting Sirtuin1 activity may have the therapeutic potential in many metabolic diseases. Also, the protective role of Sirtuin1 in endothelial function is very well established. The excellent Sirtuin1 activating property of many synthetic compounds and the metabolic regulator of Sirtuin1 ‘nicotinamide riboside’ has been shown to be very effective against many pathologies. However, the therapeutic benefit of Sirtuin1 activators in vascular diseases has not been explored extensively. To harvest the beneficial effects of Sirtuin1 on vasculature greater scientific attention is required to examine whether STACs and NR could replicate the vascular effects of Sirtuin1.
Authors Contributions
SK drafted manuscript, JK prepared figures and tables; SK and JK edited and revised manuscript.
Competing Interests
The authors declare no competing interest.
Acknowledgments
S. Kumar was supported by the National Institutes of Health (NIHR01HL152132). We thank Julia Jacobs for the editorial help.
Additional information
Funding
References
- Vassilopoulos A, Fritz KS, Petersen DR, et al. The human sirtuin family: evolutionary divergences and functions. Hum Genomics. 2011;5(5):485–496.
- Kennedy BK, Austriaco NR Jr., Zhang J, et al. Mutation in the silencing gene SIR4 can delay aging in S. cerevisiae. Cell. 1995;80(3):485–496.
- Rine J, Herskowitz I. Four genes responsible for a position effect on expression from HML and HMR in Saccharomyces cerevisiae. Genetics. 1987;116(1):9–22.
- Braunstein M, Rose AB, Holmes SG, et al. Transcriptional silencing in yeast is associated with reduced nucleosome acetylation. Genes Dev. 1993;7(4):592–604.
- Kaeberlein M, McVey M, The GL. SIR2/3/4 complex and SIR2 alone promote longevity in Saccharomyces cerevisiae by two different mechanisms. Genes Dev. 1999;13(19):2570–2580.
- Tissenbaum HA, Guarente L. Increased dosage of a sir-2 gene extends lifespan in Caenorhabditis elegans. Nature. 2001;410(6825):227–230.
- Sir2 GL. links chromatin silencing, metabolism, and aging. Genes Dev. 2000;14(9):1021–1026.
- Landry J, Sutton A, Tafrov ST, et al. The silencing protein SIR2 and its homologs are NAD-dependent protein deacetylases. Proc Natl Acad Sci U S A. 2000;97(11):5807–5811.
- Tanner KG, Landry J, Sternglanz R, et al. Silent information regulator 2 family of NAD- dependent histone/protein deacetylases generates a unique product, 1-O-acetyl-ADP-ribose. Proc Natl Acad Sci U S A. 2000;97(26):14178–14182.
- Smith JS, Brachmann CB, Celic I, et al. A phylogenetically conserved NAD+-dependent protein deacetylase activity in the Sir2 protein family. Proc Natl Acad Sci U S A. 2000;97(12):6658–6663.
- Imai S, Armstrong CM, Kaeberlein M, et al. Transcriptional silencing and longevity protein Sir2 is an NAD-dependent histone deacetylase. Nature. 2000;403(6771):795–800.
- Gregoretti IV, Lee YM, Goodson HV. Molecular evolution of the histone deacetylase family: functional implications of phylogenetic analysis. J Mol Biol. 2004;338(1):17–31.
- Bordone L, Cohen D, Robinson A, et al. SIRT1 transgenic mice show phenotypes resembling calorie restriction. Aging Cell. 2007;6(6):759–767.
- Satoh A, Brace CS, Rensing N, et al. Sirt1 extends life span and delays aging in mice through the regulation of Nk2 homeobox 1 in the DMH and LH. Cell Metab. 2013;18(3):416–430.
- Mercken EM, Hu J, Krzysik-Walker S, et al. SIRT1 but not its increased expression is essential for lifespan extension in caloric-restricted mice. Aging Cell. 2014;13(1):193–196.
- Afshar G, Murnane JP. Characterization of a human gene with sequence homology to Saccharomyces cerevisiae SIR2. Gene. 1999;234(1):161–168.
- Martin SG, Laroche T, Suka N, et al. Relocalization of telomeric Ku and SIR proteins in response to DNA strand breaks in yeast. Cell. 1999;97(5):621–633.
- Lambert PF, Kashanchi F, Radonovich MF, et al. Phosphorylation of p53 serine 15 increases interaction with CBP. J Biol Chem. 1998;273(49):33048–33053.
- Vaziri H, Dessain SK, Ng Eaton E, et al. hSIR2(SIRT1) functions as an NAD-dependent p53 deacetylase. Cell. 2001;107(2):149–159.
- Luo J, Nikolaev AY, Imai S, et al. Negative control of p53 by Sir2alpha promotes cell survival under stress. Cell. 2001;107(2):137–148.
- Cheng HL, Mostoslavsky R, Saito S, et al. Developmental defects and p53 hyperacetylation in Sir2 homolog (SIRT1)-deficient mice. Proc Natl Acad Sci U S A. 2003;100(19):10794–10799.
- Brunet A, Bonni A, Zigmond MJ, et al. Akt promotes cell survival by phosphorylating and inhibiting a Forkhead transcription factor. Cell. 1999;96(6):857–868.
- Lin K, Dorman JB, Rodan A, et al. daf-16: an HNF-3/forkhead family member that can function to double the life-span of Caenorhabditis elegans. Science. 1997;278(5341):1319–1322.
- Tran H, Brunet A, Grenier JM, et al. DNA repair pathway stimulated by the forkhead transcription factor FOXO3a through the Gadd45 protein. Science. 2002;296(5567):530–534.
- Motta MC, Divecha N, Lemieux M, et al. Mammalian SIRT1 represses forkhead transcription factors. Cell. 2004;116(4):551–563.
- Brunet A, Sweeney LB, Sturgill JF, et al. Stress-dependent regulation of FOXO transcription factors by the SIRT1 deacetylase. Science. 2004;303(5666):2011–2015.
- Nemoto S, Fergusson MM, Finkel T. Nutrient availability regulates SIRT1 through a forkhead-dependent pathway. Science. 2004;306(5704):2105–2108.
- Van Der Horst A, Tertoolen LG, de Vries-Smits LM, et al. FOXO4 is acetylated upon peroxide stress and deacetylated by the longevity protein hSir2(SIRT1). J Biol Chem. 2004;279(28):28873–28879.
- Kops GJ, Dansen TB, Polderman PE, et al. Forkhead transcription factor FOXO3a protects quiescent cells from oxidative stress. Nature. 2002;419(6904):316–321.
- Chen L, Fischle W, Verdin E, et al. Duration of nuclear NF-kappaB action regulated by reversible acetylation. Science. 2001;293(5535):1653–1657.
- Ashburner BP, Westerheide SD, Baldwin AS Jr. The p65 (RelA) subunit of NF-kappaB interacts with the histone deacetylase (HDAC) corepressors HDAC1 and HDAC2 to negatively regulate gene expression. Mol Cell Biol. 2001;21(20):7065–7077.
- Yeung F, Hoberg JE, Ramsey CS, et al. Modulation of NF-kappaB-dependent transcription and cell survival by the SIRT1 deacetylase. EMBO J. 2004;23(12):2369–2380.
- Kauppinen A, Suuronen T, Ojala J, et al. Antagonistic crosstalk between NF-kappaB and SIRT1 in the regulation of inflammation and metabolic disorders. Cell Signal. 2013;25(10):1939–1948.
- Puca R, Nardinocchi L, Givol D, et al. Regulation of p53 activity by HIPK2: molecular mechanisms and therapeutical implications in human cancer cells. Oncogene. 2010;29(31):4378–4387.
- Hwang J, Lee SY, Choi JR, et al. SIRT1 negatively regulates the protein stability of HIPK2. Biochem Biophys Res Commun. 2013;441(4):799–804.
- Conrad E, Polonio-Vallon T, Meister M, et al. HIPK2 restricts SIRT1 activity upon severe DNA damage by a phosphorylation-controlled mechanism. Cell Death Differ. 2016;23(1):110–122.
- Bluher M, Kahn BB, Kahn CR. Extended longevity in mice lacking the insulin receptor in adipose tissue. Science. 2003;299(5606):572–574.
- Tontonoz P, Hu E, Spiegelman BM. Stimulation of adipogenesis in fibroblasts by PPAR gamma 2, a lipid-activated transcription factor. Cell. 1994;79(7):1147–1156.
- Picard F, Kurtev M, Chung N, et al. Sirt1 promotes fat mobilization in white adipocytes by repressing PPAR-gamma. Nature. 2004;429(6993):771–776.
- Han L, Zhou R, Niu J, et al. SIRT1 is regulated by a PPAR{gamma}-SIRT1 negative feedback loop associated with senescence. Nucleic Acids Res. 2010;38(21):7458–7471.
- Puigserver P, Wu Z, Park CW, et al. A cold-inducible coactivator of nuclear receptors linked to adaptive thermogenesis. Cell. 1998;92(6):829–839.
- Yoon JC, Puigserver P, Chen G, et al. Control of hepatic gluconeogenesis through the transcriptional coactivator PGC-1. Nature. 2001;413(6852):131–138.
- Rodgers JT, Lerin C, Haas W, et al. Nutrient control of glucose homeostasis through a complex of PGC-1alpha and SIRT1. Nature. 2005;434(7029):113–118.
- Nemoto S, Fergusson MM, Finkel T. SIRT1 Functionally Interacts with the Metabolic Regulator and Transcriptional Coactivator PGC-1α. J Biol Chem. 2005;280(16):16456–16460.
- Cohen HY, Lavu S, Bitterman KJ, et al. Acetylation of the C terminus of Ku70 by CBP and PCAF controls Bax-mediated apoptosis. Mol Cell. 2004;13(5):627–638.
- Jeong J, Juhn K, Lee H, et al. SIRT1 promotes DNA repair activity and deacetylation of Ku70. Exp Mol Med. 2007;39(1):8–13.
- Yamamori T, DeRicco J, Naqvi A, et al. SIRT1 deacetylates APE1 and regulates cellular base excision repair. Nucleic Acids Res. 2010;38(3):832–845.
- Jung S-B, Kim C-S, Kim Y-R, et al. Redox factor-1 activates endothelial SIRTUIN1 through reduction of conserved cysteine sulfhydryls in its deacetylase domain. PLoS One. 2013;8(6):e65415.
- Vaquero A, Scher M, Lee D, et al. interacts with histone H1 and promotes formation of facultative heterochromatin. Mol Cell. 2004;16(1):93–105.
- Vaquero A, Scher M, Erdjument-Bromage H, et al. SIRT1 regulates the histone methyl-transferase SUV39H1 during heterochromatin formation. Nature. 2007;450(7168):440–444.
- Gronroos E, Hellman U, Heldin C-H, et al. Control of Smad7 stability by competition between acetylation and ubiquitination. Mol Cell. 2002;10(3):483–493.
- Kume S, Haneda M, Kanasaki K, et al. SIRT1 inhibits transforming growth factor beta-induced apoptosis in glomerular mesangial cells via Smad7 deacetylation. J Biol Chem. 2007;282(1):151–158.
- Starai VJ. Sir2-dependent activation of acetyl-CoA synthetase by deacetylation of active lysine. Science. 2002;298(5602):2390–2392.
- Hallows WC, Lee S, Denu JM. Sirtuins deacetylate and activate mammalian acetyl-CoA synthetases. Proc Natl Acad Sci U S A. 2006;103(27):10230–10235.
- Kalaany NY, Mangelsdorf DJ. LXRS and FXR: the yin and yang of cholesterol and fat metabolism. Annu Rev Physiol. 2006;68(1):159–191.
- Li X, Zhang S, Blander G, et al. SIRT1 deacetylates and positively regulates the nuclear receptor LXR. Mol Cell. 2007;28(1):91–106.
- Tanno M, Sakamoto J, Miura T, et al. Nucleocytoplasmic shuttling of the NAD+-dependent histone deacetylase SIRT1. J Biol Chem. 2007;282(9):6823–6832.
- Rӧssig L, Urbich C, Brühl T, et al. Histone deacetylase activity is essential for the expression of HoxA9 and for endothelial commitment of progenitor cells. J Exp Med. 2005;201(11):1825–1835.
- Nisoli E, Tonello C, Cardile A, Cozzi V, Bracale R, Tedesco L, Falcone S, Valerio A, Cantoni O, Clementi E, Moncada S, Carruba MO. Calorie restriction promotes mitochondrial biogenesis by inducing the expression of eNOS. Science. 2005 Oct 14;310(5746):314–7. doi: 10.1126/science.1117728. PMID: 16224023
- Mattagajasingh I, Kim C-S, Naqvi A, et al. SIRT1 promotes endothelium-dependent vascular relaxation by activating endothelial nitric oxide synthase. Proc Natl Acad Sci U S A. 2007;104(37):14855–14860.
- Ota H, Eto M, Kano MR, et al. Cilostazol inhibits oxidative stress–induced premature senescence via upregulation of sirt1 in human endothelial cells. Arterioscler Thromb Vasc Biol. 2008;28(9):1634–1639.
- Chen Z, Peng I-C, Cui X, et al. Shear stress, SIRT1, and vascular homeostasis. Proc Natl Acad Sci U S A. 2010;107(22):10268–10273.
- Zhao T, Li J, Chen AF. MicroRNA-34a induces endothelial progenitor cell senescence and impedes its angiogenesis via suppressing silent information regulator 1. Am J Physiol Endocrinol Metab. 2010;299(1):E110–6.
- Li Q, Kim Y-R, Vikram A, et al. P66Shc-Induced MicroRNA-34a causes diabetic endothelial dysfunction by downregulating sirtuin1. Arterioscler Thromb Vasc Biol. 2016;36(12):2394–2403.
- Vikram A, Kim Y-R, Kumar S, et al. Vascular microRNA-204 is remotely governed by the microbiome and impairs endothelium-dependent vasorelaxation by downregulating Sirtuin1. Nat Commun. 2016;7(1):12565.
- Kassan M, Vikram A, Kim Y-R, et al. Sirtuin1 protects endothelial Caveolin-1 expression and preserves endothelial function via suppressing miR-204 and endoplasmic reticulum stress. Sci Rep. 2017;7(1):42265.
- Kassan M, Vikram A, Li Q, et al. MicroRNA-204 promotes vascular endoplasmic reticulum stress and endothelial dysfunction by targeting Sirtuin1. Sci Rep. 2017;7(1):9308.
- Donato AJ, Magerko KA, Lawson BR, et al. SIRT-1 and vascular endothelial dysfunction with ageing in mice and humans. J Physiol. 2011;589(18):4545–4554.
- Thompson AM, Wagner R, Rzucidlo EM. Age-related loss of SirT1 expression results in dysregulated human vascular smooth muscle cell function. Am J Physiol Heart Circ Physiol. 2014;307(4):H533–41.
- Kong D, Zhan Y, Liu Z, Kong D, Zhan Y, Liu Z, Ding T, Li M, Yu H, et al. SIRT1-mediated ERβ suppression in the endothelium contributes to vascular aging. Aging Cell. 2016;15(6):1092–1102.
- Zhu Y, Bian Z, Lu P, Karas RH, Bao L, Cox D, Hodgin J, Shaul PW, Thoren P, Smithies O, Gustafsson JA, Mendelsohn ME. Abnormal vascular function and hypertension in mice deficient in estrogen receptor beta. Science. 2002 Jan 18;295(5554):505–8. doi:10.1126/science.1065250. PMID: 11799247
- Ota H, Akishita M, Eto M, et al. Sirt1 modulates premature senescence-like phenotype in human endothelial cells. J Mol Cell Cardiol. 2007;43(5):571–579.
- Gracia-Sancho J, Villarreal G Jr., Zhang Y, et al. Activation of SIRT1 by resveratrol induces KLF2 expression conferring an endothelial vasoprotective phenotype. Cardiovasc Res. 2010;85(3):514–519.
- Wan Y-Z, Gao P, Zhou S, et al. SIRT 1-mediated epigenetic downregulation of plasminogen activator inhibitor-1 prevents vascular endothelial replicative senescence. Aging Cell. 2014;13(5):890–899.
- Zu Y, Liu L, Lee MYK, et al. SIRT1 promotes proliferation and prevents senescence through targeting LKB1 in primary porcine aortic endothelial cells. Circ Res. 2010;106(8):1384–1393.
- Bai B, Man AWC, Yang K, et al. Endothelial SIRT1 prevents adverse arterial remodeling by facilitating HERC2-mediated degradation of acetylated LKB1. Oncotarget. 2016;7(26):39065–39081.
- Guo Y, Xu C, Man AWC, Bai B, Luo C, Huang Y, Xu A, Vanhoutte PM, Wang Y. Endothelial SIRT1 prevents age-induced impairment of vasodilator responses by enhancing the expression and activity of soluble guanylyl cyclase in smooth muscle cells. Cardiovasc Res. 2019 Mar 1;115(3):678–690. doi: 10.1093/cvr/cvy212. PMID: 30165439
- Potente M, Ghaeni L, Baldessari D, et al. SIRT1 controls endothelial angiogenic functions during vascular growth. Genes Dev. 2007;21(20):2644–2658.
- Maizel J, Xavier S, Chen J, et al. Sirtuin 1 ablation in endothelial cells is associated with impaired angiogenesis and diastolic dysfunction. Am J Physiol Heart Circ Physiol. 2014;307(12):H1691–704.
- Guarani V, Deflorian G, Franco CA, et al. Acetylation-dependent regulation of endothelial Notch signalling by the SIRT1 deacetylase. Nature. 2011;473(7346):234–238.
- Csiszar A, Labinskyy N, Podlutsky A, et al. Vasoprotective effects of resveratrol and SIRT1: attenuation of cigarette smoke-induced oxidative stress and proinflammatory phenotypic alterations. Am J Physiol Heart Circ Physiol. 2008;294(6):H2721–35.
- Arunachalam G, Yao H, Sundar IK, et al. SIRT1 regulates oxidant- and cigarette smoke-induced eNOS acetylation in endothelial cells: role of resveratrol. Biochem Biophys Res Commun. 2010;393(1):66–72.
- Ungvari Z, Labinskyy N, Mukhopadhyay P, et al. Resveratrol attenuates mitochondrial oxidative stress in coronary arterial endothelial cells. Am J Physiol Heart Circ Physiol. 2009;297(5):H1876–81.
- Csiszar A, Labinskyy N, Pinto JT, et al. Resveratrol induces mitochondrial biogenesis in endothelial cells. Am J Physiol Heart Circ Physiol. 2009;297(1):H13–20.
- Kumar S, Kim Y-R, Vikram A, et al. Sirtuin1-regulated lysine acetylation of p66Shc governs diabetes-induced vascular oxidative stress and endothelial dysfunction. Proc Natl Acad Sci U S A. 2017;114(7):1714–1719.
- Guarente L. Sirtuins as potential targets for metabolic syndrome. Nature. 2006;444(7121):868–874.
- Sheetz MJ, and King GL. Molecular understanding of hyperglycemia’s adverse effects for diabetic complications. JAMA. 2002;288(20):2579–2588.
- Orimo M, Minamino T, Miyauchi H, et al. Protective role of SIRT1 in diabetic vascular dysfunction. Arterioscler Thromb Vasc Biol. 2009;29(6):889–894.
- Chen H, Wan Y, Zhou S, et al. Endothelium-specific SIRT1 overexpression inhibits hyperglycemia-induced upregulation of vascular cell senescence. Sci China Life Sci. 2012;55(6):467–473.
- Zhou S, Chen H-Z, Wan Y-Z, et al. Repression of P66Shc expression by SIRT1 contributes to the prevention of hyperglycemia-induced endothelial dysfunction. Circ Res. 2011;109(6):639–648.
- Kedenko L, Lamina C, Kedenko I, et al. Genetic polymorphisms at SIRT1 and FOXO1 are associated with carotid atherosclerosis in the SAPHIR cohort. BMC Med Genet. 2014;15:112.
- Ramkaran P, Phulukdaree A, Khan S, et al. Sirtuin 1 rs1467568 and rs7895833 in South African Indians with early-onset coronary artery disease. Cardiovasc J Afr. 2016;27(4):213–217.
- Nasiri M, Rauf M, Kamfiroozie H, et al. SIRT1 gene polymorphisms associated with decreased risk of atherosclerotic coronary artery disease. Gene. 2018;672:16–20.
- Zhang QJ, Wang Z, Chen HZ, et al. Endothelium-specific overexpression of class III deacetylase SIRT1 decreases atherosclerosis in apolipoprotein E-deficient mice. Cardiovasc Res. 2008;80(2):191–199.
- Stein S, Schafer N, Breitenstein A, et al. SIRT1 reduces endothelial activation without affecting vascular function in ApoE-/- mice. Aging (Albany NY). 2010;2(6):353–360.
- Yin Y, Li X, Sha X, et al. Early hyperlipidemia promotes endothelial activation via a caspase-1-sirtuin 1 pathway. Arterioscler Thromb Vasc Biol. 2015;35(4):804–816.
- Miyazaki R, Ichiki T, Hashimoto T, et al. SIRT1, a longevity gene, downregulates angiotensin II type 1 receptor expression in vascular smooth muscle cells. Arterioscler Thromb Vasc Biol. 2008;28(7):1263–1269.
- Li L, Gao P, Zhang H, et al. SIRT1 inhibits angiotensin II-induced vascular smooth muscle cell hypertrophy. Acta Biochim Biophys Sin (Shanghai). 2011;43(2):103–109.
- Li L, Zhang HN, Chen HZ, et al. SIRT1 acts as a modulator of neointima formation following vascular injury in mice. Circ Res. 2011;108(10):1180–1189.
- Gorenne I, Kumar S, Gray K, et al. Vascular smooth muscle cell sirtuin 1 protects against DNA damage and inhibits atherosclerosis. Circulation. 2013;127(3):386–396.
- Fry JL, Al Sayah L, Weisbrod RM, et al. Vascular smooth muscle sirtuin-1 protects against diet-induced aortic stiffness. Hypertension. 2016;68(3):775–784.
- Chen HZ, Wang F, Gao P, et al. Age-associated sirtuin 1 reduction in vascular smooth muscle links vascular senescence and inflammation to abdominal aortic aneurysm. Circ Res. 2016;119(10):1076–1088.
- Bartoli-Leonard F, Wilkinson FL, Schiro A, et al. Suppression of SIRT1 in diabetic conditions induces osteogenic differentiation of human vascular smooth muscle cells via RUNX2 signalling. Sci Rep. 2019;9(1):878.
- Wu H, Montone KT. Cortactin localization in actin-containing adult and fetal tissues. J Histochem Cytochem. 1998;46(10):1189–1191.
- Schnoor M, Lai FP, Zarbock A, et al. Cortactin deficiency is associated with reduced neutrophil recruitment but increased vascular permeability in vivo. J Exp Med. 2011;208(8):1721–1735.
- Zhang Y, Zhang M, Dong H, et al. Deacetylation of cortactin by SIRT1 promotes cell migration. Oncogene. 2009;28(3):445–460.
- Shentu TP, He M, Sun X, et al. AMP-Activated protein kinase and sirtuin 1 coregulation of cortactin contributes to endothelial function. Arterioscler Thromb Vasc Biol. 2016;36(12):2358–2368.
- Li Y, Wang P, Yang X, et al. SIRT1 inhibits inflammatory response partly through regulation of NLRP3 inflammasome in vascular endothelial cells. Mol Immunol. 2016;77:148–156.
- Li Y, Yang X, He Y, et al. Negative regulation of NLRP3 inflammasome by SIRT1 in vascular endothelial cells. Immunobiology. 2017;222(3):552–561.
- Frye RA. Characterization of five human cDNAs with homology to the yeast SIR2 gene: sir2-like proteins (sirtuins) metabolize NAD and may have protein ADP-ribosyltransferase activity. Biochem Biophys Res Commun. 1999;260(1):273–279.
- Kt H, KJ B, HY C, et al. Small molecule activators of sirtuins extend Saccharomyces cerevisiae lifespan. Nature. 2003;425(6954):191–196.
- Bonkowski MS, Sinclair DA. Slowing ageing by design: the rise of NAD(+) and sirtuin-activating compounds. Nat Rev Mol Cell Biol. 2016;17(11):679–690.
- Napper AD, Hixon J, McDonagh T, et al. Discovery of indoles as potent and selective inhibitors of the deacetylase SIRT1. J Med Chem. 2005;48(25):8045–8054.
- Dai H, Case AW, Riera TV, et al. Crystallographic structure of a small molecule SIRT1 activator-enzyme complex. Nat Commun. 2015;6:7645.
- Ja B, KJ P, Nl P, et al. Resveratrol improves health and survival of mice on a high-calorie diet. Nature. 2006;444(7117):337–342.
- Lagouge M, Argmann C, Gerhart-Hines Z, et al. Resveratrol improves mitochondrial function and protects against metabolic disease by activating SIRT1 and PGC-1alpha. Cell. 2006;127(6):1109–1122.
- Timmers S, Konings E, Bilet L, et al. Calorie restriction-like effects of 30 days of resveratrol supplementation on energy metabolism and metabolic profile in obese humans. Cell Metab. 2011;14(5):612–622.
- Howells LM, Berry DP, Elliott PJ, et al. Phase I randomized, double-blind pilot study of micronized resveratrol (SRT501) in patients with hepatic metastases–safety, pharmacokinetics, and pharmacodynamics. Cancer Prev Res (Phila). 2011;4(9):1419–1425.
- Brasnyo P, Molnar GA, Mohas M, et al. Resveratrol improves insulin sensitivity, reduces oxidative stress and activates the Akt pathway in type 2 diabetic patients. Br J Nutr. 2011;106(3):383–389.
- Poulsen MM, Vestergaard PF, Clasen BF, et al. High-dose resveratrol supplementation in obese men: an investigator-initiated, randomized, placebo-controlled clinical trial of substrate metabolism, insulin sensitivity, and body composition. Diabetes. 2013;62(4):1186–1195.
- Yoshino J, Conte C, Fontana L, et al. Resveratrol supplementation does not improve metabolic function in nonobese women with normal glucose tolerance. Cell Metab. 2012;16(5):658–664.
- Van Der Made SM, Plat J, Mensink RP. Resveratrol does not influence metabolic risk markers related to cardiovascular health in overweight and slightly obese subjects: a randomized, placebo-controlled crossover trial. PLoS One. 2015;10(3):e0118393.
- Baksi A, Kraydashenko O, Zalevkaya A, et al. A phase II, randomized, placebo-controlled, double-blind, multi-dose study of SRT2104, a SIRT1 activator, in subjects with type 2 diabetes. Br J Clin Pharmacol. 2014;78(1):69–77.
- Venkatasubramanian S, Noh RM, Daga S, et al. Cardiovascular effects of a novel SIRT1 activator, SRT2104, in otherwise healthy cigarette smokers. J Am Heart Assoc. 2013;2(3):e000042.
- Hoffmann E, Wald J, Lavu S, et al. Pharmacokinetics and tolerability of SRT2104, a first-in-class small molecule activator of SIRT1, after single and repeated oral administration in man. Br J Clin Pharmacol. 2013;75(1):186–196.
- Belenky P, Racette FG, Bogan KL, et al. Nicotinamide riboside promotes Sir2 silencing and extends lifespan via Nrk and Urh1/Pnp1/Meu1 pathways to NAD+. Cell. 2007;129(3):473–484.
- Canto C, Houtkooper RH, Pirinen E, et al. The NAD(+) precursor nicotinamide riboside enhances oxidative metabolism and protects against high-fat diet-induced obesity. Cell Metab. 2012;15(6):838–847.
- Trammell SA, Weidemann BJ, Chadda A, et al. Nicotinamide riboside opposes type 2 diabetes and neuropathy in mice. Sci Rep. 2016;6:26933.
- Zhou CC, Yang X, Hua X, et al. Hepatic NAD(+) deficiency as a therapeutic target for non-alcoholic fatty liver disease in ageing. Br J Pharmacol. 2016;173(15):2352–2368.
- Trammell SA, Schmidt MS, Weidemann BJ, et al. Nicotinamide riboside is uniquely and orally bioavailable in mice and humans. Nat Commun. 2016;7:12948.
- Matasic DS, Brenner C, London B. Emerging potential benefits of modulating NAD(+) metabolism in cardiovascular disease. Am J Physiol Heart Circ Physiol. 2018;314(4):H839–H52.
- Diguet N, Trammell SAJ, Tannous C, et al. Nicotinamide riboside preserves cardiac function in a mouse model of dilated cardiomyopathy. Circulation. 2018;137(21):2256–2273.
- de Picciotto NE, Gano LB, Johnson LC, et al. Nicotinamide mononucleotide supplementation reverses vascular dysfunction and oxidative stress with aging in mice. Aging Cell. 2016;15(3):522–530.
- Martens CR, Denman BA, Mazzo MR, et al. Chronic nicotinamide riboside supplementation is well-tolerated and elevates NAD(+) in healthy middle-aged and older adults. Nat Commun. 2018;9(1):1286.
- Dai JM, Wang ZY, Sun DC, et al. SIRT1 interacts with p73 and suppresses p73-dependent transcriptional activity. J Cell Physiol. 2007;210(1):161–166.
- Xiong S, Salazar G, Patrushev N, et al. FoxO1 mediates an autofeedback loop regulating SIRT1 expression. J Biol Chem. 2011;286(7):5289–5299.
- Ikenoue T, Inoki K, Zhao B, et al. PTEN acetylation modulates its interaction with PDZ domain. Cancer Res. 2008;68(17):6908–6912.
- Zhao X, Sternsdorf T, Bolger TA, et al. Regulation of MEF2 by histone deacetylase 4- and SIRT1 deacetylase-mediated lysine modifications. Mol Cell Biol. 2005;25(19):8456–8464.
- Dioum EM, Chen R, Alexander MS, et al. Regulation of hypoxia-inducible factor 2alpha signaling by the stress-responsive deacetylase sirtuin 1. Science. 2009;324(5932):1289–1293.
- Lim JH, Lee YM, Chun YS, et al. Sirtuin 1 modulates cellular responses to hypoxia by deacetylating hypoxia-inducible factor 1alpha. Mol Cell. 2010;38(6):864–878.
- Leiser SF, Kaeberlein M. A role for SIRT1 in the hypoxic response. Mol Cell. 2010;38(6):779–780.
- Muth V, Nadaud S, Grummt I, et al. Acetylation of TAF(I)68, a subunit of TIF-IB/SL1, activates RNA polymerase I transcription. EMBO J. 2001;20(6):1353–1362.
- Ponugoti B, Kim DH, Xiao Z, et al. SIRT1 deacetylates and inhibits SREBP-1C activity in regulation of hepatic lipid metabolism. J Biol Chem. 2010;285(44):33959–33970.
- Firestein R, Blander G, Michan S, et al. The SIRT1 deacetylase suppresses intestinal tumorigenesis and colon cancer growth. PLoS One. 2008;3(4):e2020.
- Schug TT, Xu Q, Gao H, et al. Myeloid deletion of SIRT1 induces inflammatory signaling in response to environmental stress. Mol Cell Biol. 2010;30(19):4712–4721.
- Nakahata Y, Kaluzova M, Grimaldi B, et al. The NAD+-dependent deacetylase SIRT1 modulates CLOCK-mediated chromatin remodeling and circadian control. Cell. 2008;134(2):329–340.
- Asher G, Gatfield D, Stratmann M, et al. SIRT1 regulates circadian clock gene expression through PER2 deacetylation. Cell. 2008;134(2):317–328.
- Fan W, Luo J. SIRT1 regulates UV-induced DNA repair through deacetylating XPA. Mol Cell. 2010;39(2):247–258.
- Zhang J. The direct involvement of SirT1 in insulin-induced insulin receptor substrate-2 tyrosine phosphorylation. J Biol Chem. 2007;282(47):34356–34364.
- Li Y, Xu W, McBurney MW, et al. SirT1 inhibition reduces IGF-I/IRS-2/Ras/ERK1/2 signaling and protects neurons. Cell Metab. 2008;8(1):38–48.
- Pediconi N, Guerrieri F, Vossio S, et al. hSirT1-dependent regulation of the PCAF-E2F1-p73 apoptotic pathway in response to DNA damage. Mol Cell Biol. 2009;29(8):1989–1998.
- Wang J, Chen J. SIRT1 regulates autoacetylation and histone acetyltransferase activity of TIP60. J Biol Chem. 2010;285(15):11458–11464.
- Vempati RK, Jayani RS, Notani D, et al. p300-mediated acetylation of histone H3 lysine 56 functions in DNA damage response in mammals. J Biol Chem. 2010;285(37):28553–28564.
- Pagans S, Pedal A, North BJ, et al. SIRT1 regulates HIV transcription via Tat deacetylation. PLoS Biol. 2005;3(2):e41.
- Bouras T, Fu M, Sauve AA, et al. SIRT1 deacetylation and repression of p300 involves lysine residues 1020/1024 within the cell cycle regulatory domain 1. J Biol Chem. 2005;280(11):10264–10276.
- North BJ, Sinclair DA. Sirtuins: a conserved key unlocking AceCS activity. Trends Biochem Sci. 2007;32(1):1–4.
- Kim MY, Woo EM, Chong YT, et al. Acetylation of estrogen receptor alpha by p300 at lysines 266 and 268 enhances the deoxyribonucleic acid binding and transactivation activities of the receptor. Mol Endocrinol. 2006;20(7):1479–1493.
- Fu M, Liu M, Sauve AA, et al. Hormonal control of androgen receptor function through SIRT1. Mol Cell Biol. 2006;26(21):8122–8135.
- Vaquero A, Sternglanz R, Reinberg D. NAD+-dependent deacetylation of H4 lysine 16 by class III HDACs. Oncogene. 2007;26(37):5505–5520.
- North BJ, Marshall BL, Borra MT, et al. The human Sir2 ortholog, SIRT2, is an NAD+-dependent tubulin deacetylase. Mol Cell. 2003;11(2):437–444.
- Scher MB, Vaquero A. SirT3 RD. is a nuclear NAD+-dependent histone deacetylase that translocates to the mitochondria upon cellular stress. Genes Dev. 2007;21(8):920–928.
- Sundaresan NR, Samant SA, Pillai VB, et al. SIRT3 is a stress-responsive deacetylase in cardiomyocytes that protects cells from stress-mediated cell death by deacetylation of Ku70. Mol Cell Biol. 2008;28(20):6384–6401.
- Someya S, Yu W, Hallows WC, et al. Sirt3 mediates reduction of oxidative damage and prevention of age-related hearing loss under caloric restriction. Cell. 2010;143(5):802–812.
- Schlicker C, Gertz M, Papatheodorou P, et al. Substrates and regulation mechanisms for the human mitochondrial sirtuins Sirt3 and Sirt5. J Mol Biol. 2008;382(3):790–801.
- Shimazu T, Hirschey MD, Hua L, et al. SIRT3 deacetylates mitochondrial 3-hydroxy-3-methylglutaryl CoA synthase 2 and regulates ketone body production. Cell Metab. 2010;12(6):654–661.
- Schwer B, Bunkenborg J, Verdin RO, et al. Reversible lysine acetylation controls the activity of the mitochondrial enzyme acetyl-CoA synthetase 2. Proc Natl Acad Sci U S A. 2006;103(27):10224–10229.
- Cimen H, Han MJ, Yang Y, et al. Regulation of succinate dehydrogenase activity by SIRT3 in mammalian mitochondria. Biochemistry. 2010;49(2):304–311.
- Chen Y, Zhang J, Lin Y, et al. Tumour suppressor SIRT3 deacetylates and activates manganese superoxide dismutase to scavenge ROS. EMBO Rep. 2011;12(6):534–541.
- Qiu X, Brown K, Hirschey MD, et al. Calorie restriction reduces oxidative stress by SIRT3-mediated SOD2 activation. Cell Metab. 2010;12(6):662–667.
- Hirschey MD, Shimazu T, Goetzman E, et al. SIRT3 regulates mitochondrial fatty-acid oxidation by reversible enzyme deacetylation. Nature. 2010;464(7285):121–125.
- Haigis MC, Mostoslavsky R, Haigis KM, et al. SIRT4 inhibits glutamate dehydrogenase and opposes the effects of calorie restriction in pancreatic beta cells. Cell. 2006;126(5):941–954.
- Nasrin N, Wu X, Fortier E, et al. SIRT4 regulates fatty acid oxidation and mitochondrial gene expression in liver and muscle cells. J Biol Chem. 2010;285(42):31995–32002.
- Liu B, Che W, Xue J, et al. SIRT4 prevents hypoxia-induced apoptosis in H9c2 cardiomyoblast cells. Cell Physiol Biochem. 2013;32(3):655–662.
- Huang FY, Wong DK, Seto WK, et al. Tumor suppressive role of mitochondrial sirtuin 4 in induction of G2/M cell cycle arrest and apoptosis in hepatitis B virus-related hepatocellular carcinoma. Cell Death Discov. 2021;7(1):88.
- Nakagawa T, Lomb DJ, Haigis MC, et al. SIRT5 Deacetylates carbamoyl phosphate synthetase 1 and regulates the urea cycle. Cell. 2009;137(3):560–570.
- Chiba T, Peasley KD, Cargill KR, et al. Sirtuin 5 Regulates Proximal Tubule Fatty Acid Oxidation to Protect against AKI. J Am Soc Nephrol. 2019;30(12):2384–2398.
- Liu B, Che W, Zheng C, et al. SIRT5: a safeguard against oxidative stress-induced apoptosis in cardiomyocytes. Cell Physiol Biochem. 2013;32(4):1050–1059.
- Kawahara TL, Michishita E, Adler AS, et al. SIRT6 links histone H3 lysine 9 deacetylation to NF-kappaB-dependent gene expression and organismal life span. Cell. 2009;136(1):62–74.
- Michishita E, McCord RA, Berber E, et al. SIRT6 is a histone H3 lysine 9 deacetylase that modulates telomeric chromatin. Nature. 2008;452(7186):492–496.
- Michishita E, McCord RA, Boxer LD, et al. Cell cycle-dependent deacetylation of telomeric histone H3 lysine K56 by human SIRT6. Cell Cycle. 2009;8(16):2664–2666.
- Ford E, Voit R, Liszt G, et al. homolog SIRT7 is an activator of RNA polymerase I transcription. Genes Dev. 2006;20(9):1075–1080.
- Vakhrusheva O, Smolka C, Gajawada P, et al. Sirt7 increases stress resistance of cardiomyocytes and prevents apoptosis and inflammatory cardiomyopathy in mice. Circ Res. 2008;102(6):703–710.