ABSTRACT
Epitranscriptomic modifications have recently emerged into the spotlight of researchers due to their vast regulatory effects on gene expression and thereby cellular physiology and pathophysiology. N6,2‘-O-dimethyladenosine (m6Am) is one of the most prevalent chemical marks on RNA and is dynamically regulated by writers (PCIF1, METTL4) and erasers (FTO). The presence or absence of m6Am in RNA affects mRNA stability, regulates transcription, and modulates pre-mRNA splicing. Nevertheless, its functions in the heart are poorly known. This review summarizes the current knowledge and gaps about m6Am modification and its regulators in cardiac biology. It also points out technical challenges and lists the currently available techniques to measure m6Am. A better understanding of epitranscriptomic modifications is needed to improve our knowledge of the molecular regulations in the heart which may lead to novel cardioprotective strategies.
Introduction
The rapidly developing research field of epitranscriptomics has recently introduced a novel layer of gene expression regulation into cardiac biology. Over 170 chemical modifications have been found in RNA so far [Citation1]. One of the most prevalent and characterized modifications is N6-methyladenosine (m6A) [Citation2,Citation3]. Similar N6,2’-O-dimethyladenosine (m6Am) is also a common form of modified adenosine (), but it is much less studied than m6A. This modification is formed by the methylation of a 2’-O-methyladenosine (Am). It has been described in two RNA classes: messenger RNA (mRNA) and small nuclear RNA (snRNA). In mRNA, m6Am is a common part of the mRNA cap and it is located at the transcription start site just next to the well-known 5-terminal modification − 7-methylguanosine (m7G) [Citation4,Citation5]. It has been found in at least 30–40% of all transcripts in vertebrate mRNA [Citation4]. However, in specific cell lines, m6Am is even more dominant. For example, HEK293T cells have 92% of 5’capped mRNAs with m6Am and only 8% with single methylated Am [Citation6]. The presence of m6Am in mRNA markedly enhances its stability due to the increased resistance of m6Am-modified mRNA to the mRNA-decapping enzyme DCP2 [Citation7]. Two different isoforms of snRNAs reflecting the methylation state of A adjacent to the 5’ cap exist – m1 (Am) and m2 (m6Am). Cells that exhibit high m2 snRNA levels show modified patterns of alternative mRNA splicing [Citation8]. m6Am is also present at the internal sites of snRNAs [Citation9]. The m6Am/A percentage is approximately 0.01% of total RNA from human hearts [Citation10]. This modification is dynamic, and it is regulated by proteins called writers (methylation deposition) and erasers (methylation removal) (). No readers mediating the biological functions of m6Am were described so far. However, many readers binding to the more explored m6A are known. The question arises whether these RNA-binding proteins also recognize the similar m6Am modification. At present, it is known that YTH domain-containing family protein 3 (YTHDF3), one of the key m6A readers, does not bind m6Am-containing transcripts [Citation10]. For a better understanding of m6Am biology, the search for m6Am readers is needed.
Figure 1. Basic overview of m6Am modification. A – adenosine; G – guanosine; FTO – fat mass and obesity-associated; m6Am – N6,2‘-O-dimethyladenosine; m7G −7-methylguanosine; METTL4 – methyltransferase-like 4; PCIF1 – phosphorylated CTD interacting factor 1.
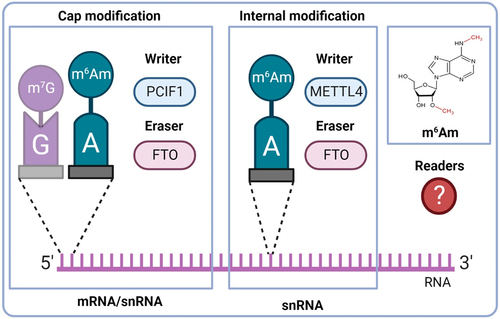
Table 1. Basic overview of adenosine methylations in RNA.
The pathological significance of m6Am and its regulation remains largely unknown. Recent data suggest that this modification plays a role in obesity [Citation11], cancer [Citation12–15], or virus–host interaction [Citation16–18], but its function in cardiac diseases has not yet been properly addressed. However, several studies have suggested the importance of fat mass and obesity-associated protein (FTO) for cardiac function. Since this demethylase has an affinity for both m6A and m6Am, the recognition of the m6Am- function of FTO is important to unravel the role of m6Am modification in cardiac physiology and pathophysiology.
m6Am writers
N6-methylation of Am to m6Am is catalysed by two known writers: phosphorylated CTD interacting factor 1 (PCIF1) and methyltransferase-like 4 (METTL4). In 2019, PCIF1 has been described as a cap-specific adenosine-N6-methyltransferase (also called CAPAM) which does not methylate adenosine residues in the RNA body [Citation6,Citation19]. However, recently it was reported that PCIF1 also has ancillary methylation activities on internal adenosines (both A and Am), although with lower affinities [Citation20]. This writer has direct and indirect impacts on RNA stability and transcription [Citation7,Citation21–23]. The second m6Am writer – METTL4 – has been described one year later in 2020. It is responsible for internal m6Am formation within U2 snRNA and affects pre-mRNA splicing [Citation24,Citation25]. METTL4 also catalyses methylation of N6-methyldeoxyadenosine (6mA), a modification in mitochondrial DNA (mtDNA), particularly under stress conditions [Citation26].
m6Am erasers
So far, the only described m6Am eraser is FTO. Originally, FTO was described as an m6A demethylase [Citation27]. However, in 2017, FTO was reported to preferentially demethylate m6Am rather than m6A [Citation7,Citation28]. Recently, it was suggested that the substrate preference of FTO might depend on its cellular localization, which varies between cell types. In the nucleus, FTO preferably targets m6A whereas cytosolic FTO demethylates especially m6Am [Citation9]. The cytosolic demethylation of m6Am was later confirmed by others [Citation12]. In cardiomyocytes, FTO is present in both the cytosol and the nucleus [Citation29]. FTO demethylates m6Am in both mRNA and snRNA [Citation9]. Additionally to m6A and m6Am, FTO can also target N1-methyladenosine (m1A) in transfer RNA (tRNA) [Citation7,Citation9].
m6Am regulation in cardiac physiology and pathophysiology
Epitranscriptomics is an emerging research field in cardiovascular physiology and pathophysiology, however, attention is mainly focused on m6A [Citation30–46]. The role of m6Am in the heart is poorly understood (). In rRNA-depleted RNA from rat adult cardiomyocytes, the m6Am levels were 9-fold higher than m6A levels, indicating the importance of m6Am modification in cellular physiology (unpublished data).
Figure 2. m6Am modification in cardiac biology. FTO – fat mass and obesity-associated; HF – heart failure; HFD – high-fat diet; hiPSC-CMs – human-induced pluripotent stem cell-derived cardiomyocytes; IF – intermittent fasting; m6Am – N6,2‘-O-dimethyladenosine; METTL4 – methyltransferase-like 4; MI – myocardial infarction; PCIF1 – phosphorylated CTD interacting factor 1; TAC – transverse aortic constriction.
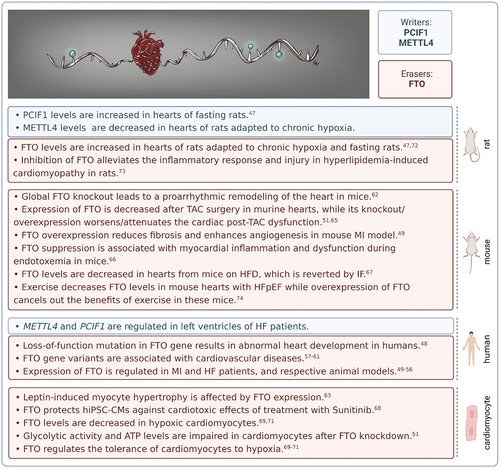
m6Am writers in cardiac physiology and pathophysiology
The role of m6Am methyltransferases in the heart is unknown. Recently, we found that METTL4 is down-regulated in the hearts of rats adapted to chronic hypoxia, a well-known cardioprotective phenomenon, while PCIF1 is not affected under these conditions (unpublished data). A regulation in protein levels of METTL4 might indicate altered mRNA splicing in hearts from chronically hypoxic animals. PCIF1, on the other hand, increased in the hearts of rats subjected to fasting, another cardioprotective intervention [Citation47]. Publicly available RNA-seq datasets generated from human left ventricles of failing and non-failing hearts report some degree of regulation of METTL4 and PCIF1. These genes were especially regulated in a large RNA-seq dataset including 356 left ventricular samples from subjects with heart failure (HF) undergoing transplantation and non-failing donors from the MAGNet consortium (). However, further research is needed to decipher the functional role of m6Am writers in HF, as there are no experimental data in animal models to date.
Table 2. Changes of m6Am regulators in left ventricles from heart failure patients (RNA-seq data).
m6Am erasers in cardiac physiology and pathophysiology
Undoubtedly, the best-studied m6Am regulator is FTO. This eraser is essential for the normal development of the cardiovascular system in humans. Loss-of-function mutation in the human FTO gene caused several heart defects (ventricular septal defect, atrioventricular defect, patent ductus arteriosus) and hypertrophic cardiomyopathy [Citation48]. Regulated expression of FTO was observed in myocardial infarction (MI) and HF patients and respective animal models [Citation49–56]. Interestingly, RNA-seq datasets that revealed regulation of m6Am writers in HF patients did not show significant changes in FTO expression (). Since HF is a heterogeneous clinical syndrome with complex pathophysiology, more studies with comparable methodology are necessary to reliably assess the role of FTO in different HF aetiologies. Gene variants of FTO were linked with various cardiovascular diseases, including MI, acute coronary syndrome, and an increased risk of rejection in heart transplant patients [Citation57–61].
Global knockout (KO) of the mouse Fto gene led to a proarrhythmic remodelling of the heart. KO mice displayed higher heart rate and heart rate variability compared to their wild-type counterparts. Moreover, they were more vulnerable to stress-induced tachyarrhythmias, their ventricular repolarization was altered, and they also developed myocardial hypertrophy [Citation62]. Studies on cultured neonatal rat cardiomyocytes showed that myocyte hypertrophy can be caused by a leptin-induced increase in FTO expression and that FTO knockdown with siRNA abrogated this event [Citation63]. Mice lacking FTO specifically in cardiomyocytes showed worsened cardiac phenotype characterized by reduced ejection fraction and increased dilatation upon transverse aortic constriction (TAC) surgery, an experimental model for pressure overload-induced cardiac hypertrophy and HF [Citation64]. TAC surgery itself led to a decreased expression of FTO while FTO overexpression attenuated the cardiac post-TAC dysfunction [Citation51,Citation65]. FTO suppression was also associated with myocardial inflammation and dysfunction during endotoxemia in mice [Citation66]. In murine isolated primary cardiomyocytes, FTO knockdown impaired the glycolytic activity and decreased ATP levels [Citation51].
Recently, it has been shown that FTO plays a role in cardioprotection. Heart tissues from mice on a high-fat diet (HFD) contained decreased levels (both mRNA and protein) of FTO, which were reversed by intermittent fasting, a nutritional approach improving HFD-induced obesity cardiomyopathy [Citation67]. These changes in FTO expression were also associated with corresponding changes in m6A/m levels (detection of m6A which does not differentiate between m6A and m6Am). Ma et al. [Citation68] showed that FTO protected human-induced pluripotent stem cell-derived cardiomyocytes (hiPSC-CMs) against treatment with Sunitinib, a tyrosine kinase inhibitor with cardiotoxic effects. Importantly, FTO also affected the tolerance of cardiomyocytes to hypoxia, a key parameter in the possible prevention of ischaemic heart disease. FTO was poorly expressed in human cardiomyocyte cell line AC16 exposed to acute hypoxia/reoxygenation (H/R) and its up-regulation improved cell viability after H/R insult [Citation69]. Likewise, in mouse cardiomyocytes, FTO overexpression inhibited apoptosis induced by acute H/R while FTO knockdown had the opposite effect [Citation70]. Moreover, Ke et al. [Citation71] observed the downexpression of FTO in mouse hearts and isolated cardiomyocytes subjected to ischaemia-reperfusion and acute H/R insults, respectively. FTO overexpression then led to attenuation of the H/R-induced apoptosis and inflammation in these cells. In our lab, we found that administration of FTO inhibitor MO-I-500 increased the lactate dehydrogenase release (which indicates cell damage) and decreased cell viability in rat cardiomyocytes under acute hypoxic conditions (unpublished data). Adaptation of rats to 3 weeks of chronic hypoxia and 3-day fasting, both cardioprotective regimes, was associated with increased myocardial levels of FTO, which might thus participate in the induction of the protection [Citation47,Citation72]. FTO overexpression also reduced fibrosis and enhanced angiogenesis in mouse models of myocardial infarction [Citation49].
Contrary to the data showing the beneficial effects of FTO on the heart, Yu et al. [Citation73] reported that inhibition of FTO using LuHui Derivative (LHD) compound alleviated the inflammatory response and injury in hyperlipidaemia-induced cardiomyopathy in rats. Similarly, mice with heart failure (HFpEF) exhibited decreased FTO levels in the heart after physical training while overexpression of FTO abolished the health benefits of exercise in these mice by promoting myocyte apoptosis, myocardial fibrosis, and myocyte hypertrophy [Citation74].
It is worth mentioning that FTO regulations in the heart might be age-dependent. According to Su et al. [Citation75], FTO levels dropped in elderly murine hearts in response to acute myocardial ischaemia/reperfusion injury while in young hearts it remained unaffected. FTO levels also changed during postnatal development [Citation76]. Moreover, the hearts of newborn male rats exhibited higher FTO protein levels than females, suggesting possible sex-dependent differences in m6Am regulations [Citation76].
These data show that FTO can have both beneficial and detrimental effects on the heart. However, current FTO studies have focused on m6A and have not included m6Am. Since many m6A detection methods do not distinguish between these two modifications, the potential involvement of m6Am could be masked. Separating the m6A-specific and m6Am-specific mechanisms of action in the future is essential for understanding the role of m6Am in cardiac biology. In rRNA-depleted RNA isolated from rat cardiomyocytes, the m6Am levels are 9-fold higher compared to m6A. Nevertheless, according to RM2Target (database for targets of writers, erasers, and readers), data on the m6Am-targets of FTO in the heart are virtually missing [Citation77].
m6Am regulators as pharmacological targets
At present, several inhibitors of demethylase FTO are available. In 2012, the natural product rhein was identified as the first cell-active inhibitor of FTO that was capable to increase the modification level of m6A in mRNA [Citation78]. Two years later, compound MO-I-500 was introduced as another specific inhibitor of this demethylase [Citation79]. A known anti-inflammatory drug meclofenamic acid (MA) was also identified as a highly selective inhibitor of FTO [Citation80]. Treatment of HeLa cells with either MO-I-500 or MA resulted in the elevation of m6A levels in mRNA [Citation79,Citation80]. Fluorescein and its derivatives were introduced as bifunctional molecules that can be used for FTO inhibition or labelling [Citation81]. Selective inhibition of FTO was also achieved by other small molecule inhibitors, such as FB23, FB232, CS1, CS2, Dac51, LHD, FTO-02, or FTO-04 [Citation73,Citation82–89]. Since FTO is a non-specific demethylase, it is expectable that its inhibition may affect levels of all its substrates. For instance, treatment of glioblastoma stem cells with FTO-04 resulted in increases in both m6A and m6Am levels [Citation87]. Unfortunately, current FTO inhibitors are not suitable for clinical use due to either low target selectivity or pharmacokinetic properties [Citation87]. Compounds affecting other m6Am regulators were not described so far. Future identification of effective pharmacological agents targeting m6Am regulation is important for the development of novel therapies for cardiac diseases.
m6Am research methods
To determine the effects of m6Am, it is necessary to properly distinguish m6Am from similar m6A. Antibodies used in the m6A detection bind both m6A and m6Am and therefore incorrectly annotate also m6Am as m6A [Citation7]. Another problem is that m6Am regulators are not m6Am-specific. METTL4 can also catalyse methylation of 6mA and FTO can demethylate m6A and m1A, so both of these regulators might affect the cardiac biology also in an m6Am-independent manner. Thus, future studies should focus on the differentiation of m6A and m6Am regulations. Various novel techniques can deal with the similarity of these two modifications and distinguish m6Am from m6A ().
Figure 3. m6Am detection methods. 2D-TLC – two‐dimensional thin‐layer chromatography; FTO – fat mass and obesity-associated protein; LC-MS/MS – liquid chromatography-tandem mass spectrometry; m6ACE-seq – m6A-crosslinking-exonuclease-sequencing; m6Am-exo-seq – m6Am-exonuclease-assisted-sequencing; m6Am-seq – m6Am-sequencing; MeRIP-seq – methyl RNA immunoprecipitation and sequencing; miCLIP – m6A individual-nucleotideresolution crosslink and immunoprecipitation; PCIF1 – phosphorylated CTD interacting factor 1; RT-Qpcr – reverse transcription quantitative real-time polymerase chain reaction.
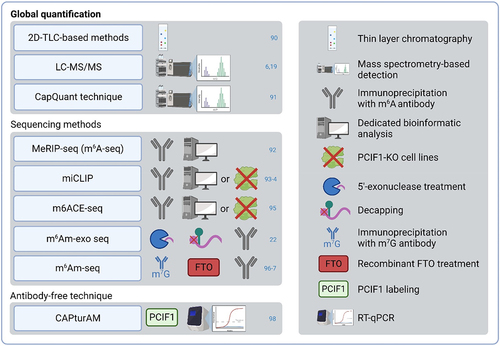
Similarly to other modifications, relative quantification of m6Am levels can be achieved by a thin layer chromatography (TLC) method or mass spectrometry-based detections such as liquid chromatography with tandem mass spectrometry (LC-MS/MS) or CapQuant technique [Citation6,Citation19,Citation90,Citation91]. Moreover, several high-throughput sequencing methods are used for m6Am profiling. The m6A antibodies used in these techniques do not differentiate between m6A and m6Am. Various approaches have been used to overcome this issue. For example, MeRIPseq (also called m6A-seq), miCLIP, or m6ACE-seq require either a dedicated bioinformatics analysis (as m6Am is present at the 5’end of mRNAs while m6A is enriched in its internal regions) or signal depletion of m6Am in the PCIF1-KO cell lines to reveal m6Am profiles [Citation92–95]. m6Am-exo-seq relies on the elimination of uncapped RNA fragments (with m6A modification), resulting in the enrichment of 5’-end RNA fragments containing m6Am. Subsequent decapping of 5’-end RNA fragments facilitates m6Am recognition by m6A antibody [Citation22]. Likewise, m6Am-seq utilizes cap-m7G immunoprecipitation to purify 5’-end RNA fragments and then uses recombinant FTO treatment to erase m6Am before sequencing. Since FTO has a higher affinity to m6Am compared to m6A in vitro, the comparison of FTO-treated and untreated samples allows the specific identification of m6Am sites [Citation96,Citation97]. Recently, CAPturAM, an antibody-independent method to selectively enrich and detect physiological targets of PCIF1 has been introduced. This technique is based on RNA labelling with recombinantly produced PCIF1 and assessment by RT-qPCR. CAPturAm allows the identification of the transcription start nucleotide N6-methylation status by comparing enrichment between WT and PCIF1-KO cells [Citation98]. In addition to these experimental methods, several computational approaches were introduced to accurately identify m6Am sites based on sequence-derived data, such as m6AmPred, MultiRM, or DLm6Am [Citation99–101].
Conclusion
m6Am is one of the most prevalent modifications of RNA. Its regulation might have a profound effect on cardiac physiology, yet the knowledge of its functional role in cardiac disease development as well as its potential value as a therapeutic target and biomarker deserves further investigation. The epitranscriptomics field remains uncharted territory that might reveal clinically relevant discoveries in the future.
Disclosure statement
No potential conflict of interest was reported by the authors.
Data availability statement
The RNA-seq data analysed in this study () are available at the Gene Expression Omnibus (GEO) data repository, which is accessible at https://www.ncbi.nlm.nih.gov/geo/.
Additional information
Funding
References
- Boccaletto P, Machnicka MA, Purta E, et al. MODOMICS: a database of RNA modification pathways. 2017 update. Nucleic Acids Res. 2018;46(D1):D303–11. DOI:10.1093/nar/gkx1030
- Desrosiers R, Friderici K, Rottman F. Identification of methylated nucleosides in messenger RNA from Novikoff hepatoma cells. Proc Natl Acad Sci U S A. 1974;71(10):3971–3975.
- Dominissini D, Moshitch-Moshkovitz S, Salmon-Divon M, et al. Transcriptome-wide mapping of N(6)-methyladenosine by m(6)A-seq based on immunocapturing and massively parallel sequencing. Nat Protoc. 2013;8(1):176–189. DOI:10.1038/nprot.2012.148
- Wei C, Gershowitz A, Moss B. N6, O2’-dimethyladenosine a novel methylated ribonucleoside next to the 5’ terminal of animal cell and virus mRnas. Nature. 1975;257(5523):251–253.
- Bokar JA. The biosynthesis and functional roles of methylated nucleosides in eukaryotic mRNA, in Fine-Tuning of RNA functions by modification and editing. H. Grosjean, Editor. Springer;Berlin Heidelberg: Berlin, Heidelberg: 2005pp. 141–177. DOI:10.1007/b106365
- Akichika S, Hirano S, Shichino Y, et al. Cap-specific terminal N 6 -methylation of RNA by an RNA polymerase II–associated methyltransferase. Science. 2019;363(6423):363(6423. DOI:10.1126/science.aav0080
- Mauer J, Luo X, Blanjoie A, et al. Reversible methylation of m6Am in the 5′ cap controls mRNA stability. Nature. 2017;541(7637):371–375. DOI:10.1038/nature21022
- Mauer J, Sindelar M, Despic V, et al. FTO controls reversible m(6)Am RNA methylation during snRNA biogenesis. Nat Chem Biol. 2019;15(4):340–347. DOI:10.1038/s41589-019-0231-8
- Wei J, Liu F, Lu Z, et al. Differential m(6)A, m(6)A(m), and m(1)A demethylation mediated by FTO in the cell nucleus and cytoplasm. Mol Cell. 2018;71(6):973–985.e5. DOI:10.1016/j.molcel.2018.08.011
- Liu J, Li K, Cai J, et al. Landscape and Regulation of m(6)A and m(6)Am methylome across human and mouse tissues. Mol Cell. 2020;77(2):426–440.e6. DOI:10.1016/j.molcel.2019.09.032
- Ben-Haim MS, Pinto Y, Moshitch-Moshkovitz S, et al. Dynamic regulation of N6,2′-O-dimethyladenosine (m6Am) in obesity. Nat Commun. 2021;12(1):7185. DOI:10.1038/s41467-021-27421-2
- Relier S, Ripoll J, Guillorit H, et al. FTO-mediated cytoplasmic m(6)A(m) demethylation adjusts stem-like properties in colorectal cancer cell. Nat Commun. 2021;12(1):1716. DOI:10.1038/s41467-021-21758-4
- Jin MZ, Zhang Y-G, Jin W-L, et al. A pan-cancer analysis of the oncogenic and immunogenic role of m6am methyltransferase PCIF1. Front Oncol. 2021;11:753393.
- Zhuo W, Sun M, Wang K, et al. M(6)am methyltransferase PCIF1 is essential for aggressiveness of gastric cancer cells by inhibiting TM9SF1 mRNA translation. Cell Discov. 2022;8(1):48. DOI:10.1038/s41421-022-00395-1
- Wang L, Wu L, Zhu Z, et al. Role of PCIF1 -mediated 5′-cap N6 -methyladeonsine mRNA methylation in colorectal cancer and anti-PD -1 immunotherapy. Embo J. 2023;42(2):e111673. DOI:10.15252/embj.2022111673
- Tartell MA, Boulias, K, Hoffmann, GB, et al. Methylation of viral mRNA cap structures by PCIF1 attenuates the antiviral activity of interferon-β. Proc Natl Acad Sci U S A. 2021;118(29):e2025769118.
- Zhang Q, Kang Y, Wang S, et al. HIV reprograms host m(6)Am RNA methylome by viral Vpr protein-mediated degradation of PCIF1. Nat Commun. 2021;12(1):5543. DOI:10.1038/s41467-021-25683-4
- Wang L, Wang S, Wu L, et al. PCIF1-mediated deposition of 5′-cap N 6 ,2′- O -dimethyladenosine in ACE2 and TMPRSS2 mRNA regulates susceptibility to SARS-CoV-2 infection. Proc Natl Acad Sci U S A. 2023;120(5):e2210361120. DOI:10.1073/pnas.2210361120
- Sun H, Zhang M, Li K, et al. Cap-specific, terminal N(6)-methylation by a mammalian m(6)Am methyltransferase. Cell Res. 2019;29(1):80–82. DOI:10.1038/s41422-018-0117-4
- Yu D, Dai N, Wolf EJ, et al. Enzymatic characterization of mRNA cap adenosine-N6 methyltransferase PCIF1 activity on uncapped RNAs. J Biol Chem. 2022;298(4):101751. DOI:10.1016/j.jbc.2022.101751
- Hirose Y, Iwamoto Y, Sakuraba K, et al. Human phosphorylated CTD-interacting protein, PCIF1, negatively modulates gene expression by RNA polymerase II. Biochem Biophys Res Commun. 2008;369(2):449–455. DOI:10.1016/j.bbrc.2008.02.042
- Sendinc E, Valle-Garcia D, Dhall A, et al. PCIF1 catalyzes m6Am mRNA methylation to regulate gene expression. Mol Cell. 2019;75(3):620–630.e9. DOI:10.1016/j.molcel.2019.05.030
- Cowling VH. CAPAM: the mRNA Cap Adenosine N6-Methyltransferase. Trends Biochem Sci. 2019;44(3):183–185.
- Goh YT, Koh C, Sim DY, et al. METTL4 catalyzes m6Am methylation in U2 snRNA to regulate pre-Mrna splicing. Nucleic Acids Res. 2020;48(16):9250–9261. DOI:10.1093/nar/gkaa684
- Chen H, Gu L, Orellana EA, et al. METTL4 is an snRNA m(6)Am methyltransferase that regulates RNA splicing. Cell Res. 2020;30(6):544–547. DOI:10.1038/s41422-019-0270-4
- Hao Z, Wu T, Cui X, et al. N(6)-Deoxyadenosine Methylation in Mammalian Mitochondrial DNA. Mol Cell. 2020;78(3):382–395.e8. DOI:10.1016/j.molcel.2020.02.018
- Jia G, Fu Y, Zhao X, et al. N6-methyladenosine in nuclear RNA is a major substrate of the obesity-associated FTO. Nat Chem Biol. 2011;7(12):885–887. DOI:10.1038/nchembio.687
- Mauer J, Jaffrey SR. FTO, m(6) A(m), and the hypothesis of reversible epitranscriptomic mRNA modifications. FEBS Lett. 2018;592(12):2012–2022.
- Phan A, Mathiyalangan P, Sahoo S. Abstract 13709: cardioprotective Mechanisms of FTO-Regulated m6A in Heart Failure. Circulation. 2022;146(Suppl_1):A13709–A13709.
- Zhang B, Jiang H, Dong Z, et al. The critical roles of m6A modification in metabolic abnormality and cardiovascular diseases. Genes Dis. 2021;8(6):746–758. DOI:10.1016/j.gendis.2020.07.011
- Longenecker JZ, Gilbert CJ, Golubeva VA, et al. Epitranscriptomics in the Heart: a Focus on m(6)A. Curr Heart Fail Rep. 2020;17(5):205–212. DOI:10.1007/s11897-020-00473-z
- Wu S, Zhang S, Wu X, et al. M(6)a RNA methylation in cardiovascular diseases. Mol Ther. 2020;28(10):2111–2119. DOI:10.1016/j.ymthe.2020.08.010
- Qin Y, Li L, Luo E, et al. Role of m6A RNA methylation in cardiovascular disease (Review). Int J Mol Med. 2020;46(6):1958–1972. DOI:10.3892/ijmm.2020.4746
- Paramasivam A, Vijayashree Priyadharsini J, Raghunandhakumar S. N6-adenosine methylation (m6A): a promising new molecular target in hypertension and cardiovascular diseases. Hypertens Res. 2020;43(2):153–154.
- Kumari R, Ranjan P, Suleiman ZG, et al. mRNA modifications in cardiovascular biology and disease: with a focus on m6A modification. Cardiovasc Res. 2022;118(7):1680–1692. DOI:10.1093/cvr/cvab160
- Leptidis S, Papakonstantinou E, Diakou K, et al. Epitranscriptomics of cardiovascular diseases (Review). Int J Mol Med. 2022;49(1).
- Sikorski V, Karjalainen P, Blokhina D, et al. Epitranscriptomics of ischemic heart disease—The IHD-EPITRAN study design and objectives. Int J Mol Sci. 2021;22(12):6630. DOI:10.3390/ijms22126630
- Chen YS, Ouyang X-P, Yu X-H, et al. N6-Adenosine Methylation (m(6)A) RNA modification: an emerging role in cardiovascular diseases. J Cardiovasc Transl Res. 2021;14(5):857–872. DOI:10.1007/s12265-021-10108-w
- Dieterich C, Völkers M. Chapter 6 - RNA modifications in cardiovascular disease—An experimental and computational perspective, in Epigenetics in Cardiovascular Disease. Y. Devaux and E.L. Robinson, Editors. Academic Press;2021pp. 113–125. DOI:10.1016/B978-0-12-822258-4.00003-1
- Zhou W, Wang C, Chang J, et al. RNA methylations in cardiovascular diseases, molecular structure, biological functions and regulatory roles in cardiovascular diseases. Front Pharmacol. 2021;12:722728.
- Peng L, Long T, Li F, et al. Emerging role of m 6 a modification in cardiovascular diseases. Cell Biol Int. 2022;46(5):711–722. DOI:10.1002/cbin.11773
- Xu Z, Lv B, Qin Y, et al. Emerging roles and mechanism of m6a methylation in cardiometabolic diseases. Cells. 2022;11(7):1101. DOI:10.3390/cells11071101
- Liu C, Gu L, Deng W, et al. N6-Methyladenosine RNA Methylation in Cardiovascular Diseases. Front Cardiovasc Med. 2022;9:887838.
- Li L, Xu N, Liu J, et al. M6a methylation in cardiovascular diseases: from mechanisms to therapeutic potential. Front Genet. 2022;13:908976.
- Fan S, Hu Y. Role of m6A methylation in the occurrence and development of heart failure. Front Cardiovasc Med. 2022;9:892113.
- Sweaad WK, Stefanizzi FM, Chamorro-Jorganes A, et al. Relevance of N6-methyladenosine regulators for transcriptome: implications for development and the cardiovascular system. J Mol Cell Cardiol. 2021;160:56–70.
- Benak D, Holzerova K, Hrdlicka J, et al. Myocardial epitranscriptomics in fasting. J Mol Cell Cardiol, ISHR Berlin. 2022;173:52. DOI:10.1016/j.yjmcc.2022.08.104
- Boissel S, Reish O, Proulx K, et al. Loss-of-function mutation in the dioxygenase-encoding FTO gene causes severe growth retardation and multiple malformations. Am J Hum Genet. 2009;85(1):106–111. DOI:10.1016/j.ajhg.2009.06.002
- Mathiyalagan P, Adamiak M, Mayourian J, et al. FTO-Dependent N 6- methyladenosine regulates cardiac function during remodeling and repair. Circulation. 2019;139(4):518–532. DOI:10.1161/CIRCULATIONAHA.118.033794
- Shi X, Cao Y, Zhang X, et al. Comprehensive analysis of N6-Methyladenosine RNA methylation regulators expression identify distinct molecular subtypes of myocardial infarction. Front Cell Dev Biol. 2021;9:756483.
- Zhang B, Jiang H, Wu J, et al. M6a demethylase FTO attenuates cardiac dysfunction by regulating glucose uptake and glycolysis in mice with pressure overload-induced heart failure. Signal Transduct Target Ther. 2021;6(1):377. DOI:10.1038/s41392-021-00699-w
- Zhang B, Xu Y, Cui X, et al. Alteration of m6A RNA methylation in heart failure with preserved ejection fraction. Front Cardiovasc Med. 2021;8:647806.
- Hinger SA, Wei J, Dorn LE, et al. Remodeling of the m(6)A landscape in the heart reveals few conserved post-transcriptional events underlying cardiomyocyte hypertrophy. J Mol Cell Cardiol. 2021;151:46–55.
- Wen C, Lan M, Tan X, et al. GSK3β Exacerbates Myocardial Ischemia/Reperfusion Injury by Inhibiting Myc. Oxid Med Cell Longev. 2022;2022:1–23.
- Wang X, Wu Y, Guo R, et al. Comprehensive analysis of n6-methyladenosine RNA methylation regulators in the diagnosis and subtype classification of acute myocardial infarction. J Immunol Res. 2022;2022:1–21.
- Vausort M, Niedolistek M, Lumley AI, et al. Regulation of N6-Methyladenosine after Myocardial Infarction. Cells. 2022;11(15):2271. DOI:10.3390/cells11152271
- Liu C, Mou S, Pan C, et al. The FTO gene rs9939609 polymorphism predicts risk of cardiovascular disease: a systematic review and meta-analysis. PLoS ONE. 2013;8(8):e71901.
- Doney ASF, Dannfald J, Kimber CH, et al. The FTO gene is associated with an atherogenic lipid profile and myocardial infarction in patients with type 2 diabetes: a genetics of diabetes audit and research study in Tayside Scotland (Go-DARTS) study. Circ Cardiovasc Genet. 2009;2(3):255–259. DOI:10.1161/CIRCGENETICS.108.822320
- Hubacek JA, Vrablik M, Dlouha D, et al. Gene variants at FTO, 9p21, and 2q36.3 are age-independently associated with myocardial infarction in Czech men. Clin Chim Acta. 2016;454:119–123.
- Hubacek JA, Stanek V, Gebauerova M, et al. A FTO variant and risk of acute coronary syndrome. Clin Chim Acta. 2010;411(15–16):1069–1072.
- Hubacek JA, Vymetalova J, Lanska V, et al. The fat mass and obesity related gene polymorphism influences the risk of rejection in heart transplant patients. Clin Transplant. 2018;32(12):e13443. DOI:10.1111/ctr.13443
- Carnevali L, Graiani G, Rossi S, et al. Signs of cardiac autonomic imbalance and proarrhythmic remodeling in FTO deficient mice. PLoS ONE. 2014;9(4):e95499.
- Gan XT, Zhao G, Huang CX, et al. Identification of fat mass and obesity associated (FTO) protein expression in cardiomyocytes: regulation by leptin and its contribution to leptin-induced hypertrophy. PLoS ONE. 2013;8(9):e74235. DOI:10.1371/journal.pone.0074235
- Berulava T, Buchholz E, Elerdashvili V, et al. Changes in m6A RNA methylation contribute to heart failure progression by modulating translation. Eur J Heart Fail. 2020;22(1):54–66. DOI:10.1002/ejhf.1672
- Li W, Xing C, Bao L, et al. Comprehensive analysis of RNA m6A methylation in pressure overload-induced cardiac hypertrophy. BMC Genomics. 2022;23(1):576. DOI:10.1186/s12864-022-08833-w
- Dubey PK, Patil M, Singh S, et al. Increased m6A-RNA methylation and FTO suppression is associated with myocardial inflammation and dysfunction during endotoxemia in mice. Mol Cell Biochem. 2022;477(1):129–141. DOI:10.1007/s11010-021-04267-2
- Xu Z, Qin Y, Lv B, et al. Intermittent fasting improves high-fat diet-induced obesity cardiomyopathy via alleviating lipid deposition and apoptosis and decreasing m6A Methylation in the Heart. Nutrients. 2022;14(2):251. DOI:10.3390/nu14020251
- Ma Y, Liu X, Bi Y, et al. Alteration of N(6)-Methyladenosine mRNA methylation in a human stem cell-derived cardiomyocyte model of tyrosine kinase inhibitor-induced cardiotoxicity. Front Cardiovasc Med. 2022;9:849175.
- Deng W, Jin Q, Li L. Protective mechanism of demethylase fat mass and obesity-associated protein in energy metabolism disorder of hypoxia-reoxygenation-induced cardiomyocytes. Exp Physiol. 2021;106(12):2423–2433.
- Shen W, Li H, Su H, et al. FTO overexpression inhibits apoptosis of hypoxia/reoxygenation-treated myocardial cells by regulating m6A modification of Mhrt. Mol Cell Biochem. 2021;476(5):2171–2179. DOI:10.1007/s11010-021-04069-6
- Ke WL, Huang Z-W, Peng C-L, et al. M 6 a demethylase FTO regulates the apoptosis and inflammation of cardiomyocytes via YAP1 in ischemia-reperfusion injury. Bioengineered. 2022;13(3):5443–5452. DOI:10.1080/21655979.2022.2030572
- Hlavackova M, Benak D, Sotakova D, et al. 4007 Fat mass and obesity-associated protein in chronically hypoxic myocardium. High Altitude Medicine & Biology. 2018;19(4):A–443. https://www.liebertpub.com/doi/10.1089/ham.2018.29015.abstracts
- Yu Y, Pan Y, Fan Z, et al. LuHui derivative, a novel compound that inhibits the fat mass and obesity-associated (FTO), alleviates the inflammatory response and injury in hyperlipidemia-induced cardiomyopathy. Front Cell Dev Biol. 2021;9:731365.
- Liu K, Ju W, Ouyang S, et al. Exercise training ameliorates myocardial phenotypes in heart failure with preserved ejection fraction by changing N6-methyladenosine modification in mice model. Front Cell Dev Biol. 2022;10:954769.
- Su X, Shen Y, Jin Y, et al. Aging-Associated Differences in Epitranscriptomic m6A regulation in response to acute cardiac ischemia/reperfusion injury in female mice. Front Pharmacol. 2021;12:654316.
- Semenovykh D, Benak D, Holzerova K, et al. Myocardial m6A regulators in postnatal development: effect of sex. Physiol Res. 2022;71(6):877–882. online. DOI:10.33549/physiolres.934970.
- Bao X, Zhang Y, Li H, et al. Rm2target: a comprehensive database for targets of writers, erasers and readers of RNA modifications. Nucleic Acids Res. 2023;51(D1):D269–d279. DOI:10.1093/nar/gkac945
- Chen B, Ye F, Yu L, et al. Development of cell-active N6-methyladenosine RNA demethylase FTO inhibitor. J Am Chem Soc. 2012;134(43):17963–17971. DOI:10.1021/ja3064149
- Zheng G, Cox T, Tribbey L, et al. Synthesis of a FTO inhibitor with anticonvulsant activity. ACS Chem Neurosci. 2014;5(8):658–665. DOI:10.1021/cn500042t
- Huang Y, Yan J, Li Q, et al. Meclofenamic acid selectively inhibits FTO demethylation of m6A over ALKBH5. Nucleic Acids Res. 2015;43(1):373–384. DOI:10.1093/nar/gku1276
- Wang T, Hong T, Huang Y, et al. Fluorescein derivatives as bifunctional molecules for the simultaneous inhibiting and labeling of FTO protein. J Am Chem Soc. 2015;137(43):13736–13739. DOI:10.1021/jacs.5b06690
- Toh JDW, Sun L, Lau LZM, et al. A strategy based on nucleotide specificity leads to a subfamily-selective and cell-active inhibitor of N 6 -methyladenosine demethylase FTO. Chem Sci. 2015;6(1):112–122. DOI:10.1039/C4SC02554G
- He W, Zhou B, Liu W, et al. Identification of a novel small-molecule binding site of the fat mass and obesity associated protein (FTO). J Med Chem. 2015;58(18):7341–7348. DOI:10.1021/acs.jmedchem.5b00702
- Svensen N, Jaffrey SR. Fluorescent RNA Aptamers as a Tool to Study RNA-Modifying Enzymes. Cell Chem Biol. 2016;23(3):415–425.
- Huang Y, Su R, Sheng Y, et al. Small- molecule targeting of oncogenic FTO Demethylase in Acute Myeloid Leukemia. Cancer Cell. 2019;35(4):677–691.e10. DOI:10.1016/j.ccell.2019.03.006
- Su R, Dong L, Li Y, et al. Targeting FTO suppresses cancer stem cell maintenance and immune Evasion. Cancer Cell. 2020;38(1):79–96.e11. DOI:10.1016/j.ccell.2020.04.017
- Huff S, Tiwari SK, Gonzalez GM, et al. M 6 A-RNA Demethylase FTO inhibitors impair self-renewal in glioblastoma stem cells. ACS Chem Biol. 2021;16(2):324–333. DOI:10.1021/acschembio.0c00841
- Qin B, Bai Q, Yan D, et al. Discovery of novel mRNA demethylase FTO inhibitors against esophageal cancer. J Enzyme Inhib Med Chem. 2022;37(1):1995–2003. DOI:10.1080/14756366.2022.2098954
- Liu Y, Liang G, Xu H, et al. Tumors exploit FTO-mediated regulation of glycolytic metabolism to evade immune surveillance. Cell Metab. 2021;33(6):1221–1233.e11. DOI:10.1016/j.cmet.2021.04.001
- Kruse S, Zhong S, Bodi Z, et al. A novel synthesis and detection method for cap-associated adenosine modifications in mouse mRNA. Sci Rep. 2011;1(1):126. DOI:10.1038/srep00126
- Wang J, Alvin Chew BL, Lai Y, et al. Quantifying the RNA cap epitranscriptome reveals novel caps in cellular and viral RNA. Nucleic Acids Res. 2019;47(20):e130. DOI:10.1093/nar/gkz751
- Schwartz S, Mumbach MR, Jovanovic M, et al. Perturbation of m6A writers reveals two distinct classes of mRNA methylation at internal and 5’ sites. Cell Rep. 2014;8(1):284–296.
- Linder B, Grozhik AV, Olarerin-George AO, et al. Single-nucleotide-resolution mapping of m6A and m6Am throughout the transcriptome. Nat Methods. 2015;12(8):767–772. DOI:10.1038/nmeth.3453
- Boulias K, Toczydłowska-Socha D, Hawley BR, et al. Identification of the m(6)Am Methyltransferase PCIF1 reveals the location and functions of m(6)Am in the Transcriptome. Mol Cell. 2019;75(3):631–643.e8. DOI:10.1016/j.molcel.2019.06.006
- Koh CWQ, Goh YT, Goh WSS. Atlas of quantitative single-base-resolution N(6)-methyl-adenine methylomes. Nat Commun. 2019;10(1):5636.
- Sun H, Li K, Zhang X, et al. M(6)am-seq reveals the dynamic m(6)Am methylation in the human transcriptome. Nat Commun. 2021;12(1):4778. DOI:10.1038/s41467-021-25105-5
- Zhang M, Sun H, Li K, et al. M(6)am RNA modification detection by m(6)Am-seq. Methods. 2022;203:242–248.
- Muthmann N, Albers M, Rentmeister A. CAPturAM, a chemo-enzymatic strategy for selective enrichment and detection of physiological CAPAM-Targets. Angew Chem Int Ed Engl. 2023;62(4):e202211957.
- Jiang J, Song B, Chen K, et al. M6ampred: identifying RNA N6, 2′-O-dimethyladenosine (m6Am) sites based on sequence-derived information. Methods. 2022;203:328–334.
- Song Z, Huang D, Song B, et al. Attention-based multi-label neural networks for integrated prediction and interpretation of twelve widely occurring RNA modifications. Nat Commun. 2021;12(1):4011. DOI:10.1038/s41467-021-24313-3
- Luo Z, Su W, Lou L, et al. Dlm6am: a deep-learning-based tool for identifying N6,2′-O-Dimethyladenosine Sites in RNA sequences. Int J Mol Sci. 2022;23(19):11026. DOI:10.3390/ijms231911026