ABSTRACT
An earlier study of ours investigating the effect of dietary lipid levels on the choline requirement of Atlantic salmon showed increasing severity of intestinal steatosis with increasing lipid levels. As choline is involved in epigenetic regulation by being the key methyl donor, pyloric caeca samples from the study were analysed for epigenetic effects of dietary lipid and choline levels. The diets varied in lipid levels between 16% and 28%, and choline levels between 1.9 and 2.3 g/kg. The diets were fed for 8 weeks to Atlantic salmon of 25 g of initial weight. Using reduced representation bisulfite sequencing (RRBS), this study revealed that increasing dietary lipid levels induced methylation differences in genes involved in membrane transport and signalling pathways, and in microRNAs important for the regulation of lipid homoeostasis. Increasing choline levels also affected genes involved in fatty acid biosynthesis and transport, lipolysis, and lipogenesis, as well as important immune genes. Our observations confirmed that choline is involved in epigenetic regulation in Atlantic salmon, as has been reported for higher vertebrates. This study showed the need for the inclusion of biomarkers of epigenetic processes in studies that must be conducted to define optimal choline levels in diets for Atlantic salmon.
Introduction
Steady increase in the replacement of fishmeal and fish oil with plant ingredients and increased lipid levels in diets for farmed Atlantic salmon have caused challenges related to the health and welfare of the fish, and fish husbandry [Citation1–3]. Excessive lipid accumulation (steatosis) in the pyloric caeca and mid intestine is an example of such health challenges and is frequently observed in today’s farmed salmon [Citation4]. A mild intestinal steatosis is a ubiquitous condition. In extreme situations, dietary fat accumulates throughout the gastrointestinal tract and lipidic faeces are discarded and float in the surrounding waters [Citation5]. With the huge changes in salmon diets in the past decades, fish have experienced changes in the contents of nutrients and other ingredient components, i.e., non-nutrients and antinutrients, with subsequent challenges, such as disturbed nutrient digestion and absorption [Citation1–3]. Increased prevalence and severity of steatosis could therefore be a consequence of complications in lipid digestion and absorption caused by an insufficient supply of essential nutrients or increased supply of antinutrients. Recently, through a series of studies, choline was established as an essential nutrient for Atlantic salmon [Citation5–9], and that plant-based diets must be supplied with choline to prevent steatosis in Atlantic salmon.
Choline is necessary for many metabolic and physiological processes by being a component of lipoproteins, membrane lipids, and a precursor for the neurotransmitter acetylcholine [Citation10,Citation11]. In the main ingredients used in salmon diets, choline is present mostly as phosphatidylcholine (PC), which is hydrolysed into lysophosphatidylcholine, absorbed, and then re-esterified to PC [Citation8]. Choline chloride is the most common choline supplement, a form which is absorbed by enterocytes and further incorporated into PC via the Kennedy pathway, sequentially reacting with ATP and cytidine triphosphate, and finally fusing with diacylglycerol [Citation8,Citation12,Citation13]. Approximately 95% of choline in the body exists as PC, which is the main component of the phospholipid bilayer of cell membranes, and thereby influences signalling and transport across membranes [Citation14]. PC is necessary for the transport of lipids from enterocytes [Citation8] and for the regulation of lipid, lipoprotein, and whole-body energy homoeostasis [Citation13].
Choline is the key source of methyl groups for DNA and histone methylation. Choline is oxidized to betaine, from which a methyl group is donated to homocysteine producing methionine, which is subsequently converted to the universal methyl donor S-adenosylmethionine (SAM) [Citation11,Citation15]. During DNA methylation, DNA methyltransferases (DNMTs) transfer a methyl group from SAM to the fifth carbon of a cytosine nucleotide, followed by a guanine nucleotide (CpG), to form 5-methylcytosine along with S-adenosylhomocysteine (SAH) [Citation16,Citation17].
Epigenetic modifications, including DNA methylation, modulate gene expression without altering the genome sequence [Citation18]. DNA methylation is the most stable and most studied epigenetic mechanism. Alterations in DNA methylation in different genomic regions differentially influence gene activity, resulting in diverse physiological and phenotypic changes [Citation16,Citation17]. DNA methylation can be modulated by environmental factors including diet and nutritional status [Citation19,Citation20]. Macro- and micronutrients have been reported to influence DNA methylation and affect health and disease conditions [Citation21–24]. Several in vivo and in vitro studies have demonstrated that changes in choline intake alter global and gene-specific DNA methylation [Citation10,Citation15,Citation25,Citation26].
In previous studies, we have demonstrated that the choline requirement of Atlantic salmon varies with variations in production-related conditions, such as dietary lipid levels [Citation6,Citation9]. In a study by Siciliani et al. [Citation9], fish in fresh water, averaging 25 g at the start, fed choline-deficient diets varying in lipid levels from 16%, 20%, 25%, and 28% for 8 weeks, showed clear, increasing effects of lipid level on intestinal steatosis, as well as a decreasing effect on lipid digestibility. Growth performance was, however, not affected by variations in the dietary lipid levels. These results support the hypothesis that dietary lipid levels affect choline requirements and are necessary for optimal feed utilization. Considering the importance of choline as a key methyl donor in DNA methylation, the present study employed reduced representation bisulfite sequencing (RRBS) to describe genome-wide DNA methylation patterns in pyloric caeca samples from the dose–response study mentioned above by Siciliani et al. [Citation9].
Results
As described in detail by Siciliani et al. [Citation9], a challenge was encountered at the start of the feeding period of the experiment. Among the newly delivered diets, the one with the highest lipid levels showed lipid leakage. A new batch was made. However, soybean lecithin was added to prevent leakage, resulting in higher choline levels. Hence, the diets with 16% (L16), and 25% (L25) lipid contained 1.9 g of choline/kg, and the diet with 28% (L28) lipid contained 2.3 g/kg of choline. See Siciliani et al. [Citation9] for further details regarding diet composition and Supplementary Table S1 for the analysed nutrient content. However, ‘every cloud has a silver lining.’ The event provided an opportunity to get indications also of effects of choline levels in epigenetic regulation in pyloric caeca tissues. L16 and L25 diets, which were similar in choline level, differed by 9% in lipid content, while the L25 and L28 diets, differed in choline by 0.4 g/kg, i.e., about 22%, but only 3% in lipid. Accordingly, the difference in results for the diets L16 and L25 indicates effects of lipid level, whereas that for L28 and L25 provides information elucidating the impact of a substantial difference in degree of choline deficiency confounded with a small difference in lipid level on the observed diet effects.
RRBS library characterization
Using the RRBS technique, we assessed epigenetic differences in the pyloric caeca of Atlantic salmon fed the L16, L25, and L28 diets. RRBS generated 21.6–40 million reads, with an average of 27.5 million raw reads per sample (Supplementary Figure S1). After quality and adaptor trimming, an average of 25.4 million reads was obtained per sample. Of them, an average of 10 million reads were uniquely mapped, with an approximately 39% mapping efficiency to the Atlantic salmon genome (Suplementary Figure S1). An average of 12.8 million reads were not mapped uniquely (approximately 51% of the trimmed reads), even though the average total mapping efficiency was 90%. Low unique mapping efficiency is a common factor experienced during RRBS studies, as reported for several fish species [Citation20,Citation24,Citation27].
Characterization of pyloric caeca methylome of Atlantic salmon
An average of 158 million cytosines were analysed per sample, including an average of 27.7 million methylated cytosines in total (). Of those methylated cytosines, an average of 26.6 million were in CpG context. An average of 4.9 million was also observed for unmethylated cytosines in CpG context, totalling up to 31.5 million cytosines in both methylated and unmethylated cytosines in CpG context. The percentage of methylated cytosines calculated as a percentage from the total sum of methylated and unmethylated cytosines respectively in CpG, CHG, and CHH context (where H is A, C, or T) are given in . Observed average percentage were 84.4, 0.9, and 0.8 methylated cytosines for CpG, CHG, and CHH contexts, respectively per diet group (). The percentage of methylated CpGs, CHGs and CHHs did not differ between the diet groups ().
Table 1. Global cytosine methylation levels detected in CpG, CHG and CHH contexts.
Differentially methylated CpGs (DMCs), their chromosomal distribution and annotation
Pairwise comparisons of DMCs among different diet groups are presented in . DMCs were presented for diets with higher lipid levels compared to diets with lower lipid levels in each comparison. The results presented below focus on the observed differences between diets L25 and L16, that is, the difference between the diets with similar choline levels and the greatest difference in lipid levels, and the difference between the diets with different choline levels and the smallest difference in lipid levels, that is, L28 and L25.
Table 2. General statistics on significantly differentially methylated cytosines in CpG context.
The chromosomal distributions of DMCs that differed between diet groups are shown in . Comparison of L25 vs L16 groups showed the highest DMCs distribution on chromosome 24, followed by 5 and 27 ()), while comparison of L28 vs L25 showed the highest distribution in chromosome 28 followed by 14 ()).
Figure 1. Bar plots showing the percentage of hyper- and hypomethylated CpGs per chromosome over all the covered CpGs in a given chromosome. (a) Comparison of L25 vs L16 diet groups and (b) L28 vs L25 diet groups. CpGs with q < 0.1 and methylation differences > 10% were considered as hyper- and hypomethylated.
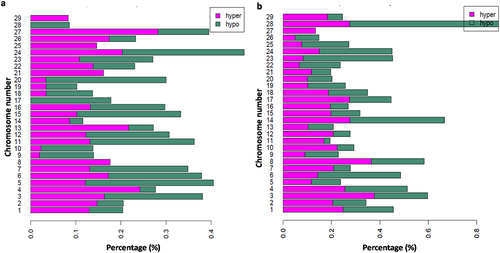
Genomic feature analysis revealed that the number of DMCs in genic regions comprising the promoter region (−1 kb to +100 bp from the transcription start site [TSS]), exons, introns, and transcription termination sites (TTS, −100 bp to +1 kb) was higher than the number of DMCs in intergenic regions for both pairwise comparisons ().
Genes associated with DMCs
The total number of genes associated with DMCs for each comparison is shown in . Approximately 50% of the genes associated with DMCs could not be assigned a gene name/symbol since the Atlantic salmon genome is still not well annotated compared to the genomes of model fish species. The number of genes associated with DMCs followed the same order as the number of DMCs found in the pairwise comparisons.
Table 3. The total number of genes associated with DMCs in each pairwise comparison of diet groups.
Gene ontology (GO) enrichment analysis of genes associated with DMCs in each comparison showed few enriched biological processes, molecular functions, and cellular components. The L25 vs L16 comparison showed enrichment of molecular functions related to transmembrane transporter activity, transcription regulator activity, oxidoreductase activity, and protein-binding activity (Supplementary Table S2). On the other hand, L28 vs L25 showed enriched biological processes, including cellular response to stimulus (Supplementary Table S2).
Supplementary File 1 presents all genes associated with DMCs in each of the comparisons between diet groups that were assigned to the gene symbol/name. Genes considered functionally relevant to the topic of the present study are presented in .
Table 4. Selected genes associated with DMCs in the pyloric caeca genome of Atlantic salmon fed diets with increasing lipid or choline content.
Few but important, relevant genes were associated with DMCs in fish fed L25 compared to those fed L16 (), that is, diets with 9% difference in lipid levels and similar choline levels. A higher number of genes were associated with DMCs in fish fed L28 compared to those fed L25, that is, diets with a difference in choline level of 0.4 g/kg (22%) and a 3% difference in lipid level ().
L25 vs L16 – effects of a 9% difference in lipid level
Relatively few genes were associated with DMCs in fish fed L25 compared with those fed L16. The promoter region of the tight junction associated peripheral protein tjap1, playing an important role in the formation of tight junctions and may participate in vesicle trafficking [Citation28,Citation29], showed hypermethylation ().
The gene coding for ARF GTPase-activating protein Git2, git2, which is important for the regulation of the toll-like receptor signalling pathway, uniquely showed hypermethylation of the promoter region of L25 fish compared to the L16 fed fish.
The gene coding for kelch-like protein 28, klhl28, showed hypomethylation in the promoter region of L25 fed fish compared to L16 fed fish. At present, there is little information available on the specific function of klhl28, but members of the kelch-like protein family are known to play important roles in ubiquitination processes mediated by E3 ligases, a processes involved in almost all life activities of eukaryotes [Citation30].
Small non-coding microRNAs which suppress the expression of targeting mRNAs were also differentially methylated, i.e., were hypermethylated in the intron region of mir-212, which is important for lipid homoeostasis [Citation31], in fish fed L25 compared to those fed L16 ().
The gene encoding potassium voltage-gated channel subfamily A member 1 (kcna1), involved in the regulation of voltage-dependent potassium ion permeability of excitable membranes [Citation32], showed hypomethylation of the exon region in fish fed L25 compared to fish fed L16 ().
L28 vs L25 – effects of a 22% difference in choline and 3% difference in lipid level
A higher number and different genes were associated with DMCs in the L28 vs L25 comparison than in the L25 vs L16 comparison (). Hypomethylation of the promoter region of tjap1 was observed, in contrast to the hypermethylation observed in the L25 vs L16 comparison. Hypomethylation of the promoter region of the carnitine and betaine transporter slc22a15 was also observed in fish fed L28 compared to those fed L16 (). A member of the major facilitator superfamily (mfs) of transporters, mfsd1 showed hypomethylation of cytosines in the transcription termination site in L28 fed fish compared to L25 fed fish. These transporters are important for the passage of a variety of small molecules across cell membranes, i.e., important for nutrient absorption [Citation33]. The lysosomal transporter mfsd1 has been shown to be vital for liver homoeostasis and liver health in mice [Citation33]. Their roles in the intestine remain to be evaluated.
Two genes belonging to the GTPase activating protein (GAP) family, acap3 and agap3 were hypermethylated in the intron region of fish fed L28 compared to the fish fed L25. GAPs bind to GTPase and accelerate the conversion of GTP to GDP, and this regulation of GTPase activity by GAPs causes a series of signalling changes [Citation34]. The GTPase activating protein acap3 is specific to the small GTPase, arf6, and those arfGAPs can be activated by lipid kinase, phosphatidylinositol 4,5-bisphosphate, and phosphatidic acids [Citation35]. However, acap3 has been mostly reported as a regulator of neuronal migration and growth [Citation36,Citation37]. Furthermore, agap3 has also been identified as an NMDA-interacting signalling protein that is important for regulating NMDA receptors [Citation38]. NMDA receptors are glutamate-gated ion channels present in excitatory synapses in the central nervous system and are important for mediating sodium, calcium, and potassium ion flow in the cells [Citation39].
The promoter region of scd, a gene encoding acyl-CoA desaturase involved in fatty acid biosynthesis, showed hypomethylation in L28 fed fish compared to L25 fed fish ().
In contrast to the effect of lipid levels on hypermethylation of the intron region of mir-212, this microRNA was not significantly affected by DMCs in the L28 vs L25 comparison.
Among the immune genes associated with DMCs were transcription factor, irf4a, hypomethylated in the intron region, and somatostatin receptor, sstr2a, hypermethylated in the promoter region of fish fed L28 compared to those fed L25 ().
Several genes related to the nervous system and neurotransmitters showed differences in epigenetic regulation between the L28 and L25 diet fed fish, possibly linking to the role of choline as precursor for the neurotransmitter acetylcholine. In addition to the previously mentioned acap3 and agap3, gabrb2, a multi-subunit chloride channel that mediates inhibitory synaptic transmission, showed differences in epigenetic regulation between L28 and L25 fed fish. Hypermethylation of kcna1 observed in the L28 vs L25 comparison, in contrast to hypomethylation of kcna1 observed in the L25 vs L16 comparison ().
Discussion
Studies describing diet-related epigenetic changes in fish are few [Citation20,Citation24,Citation40]. The present study adds new information to the currently weak knowledge base. The comparison of the epigenetic differences in fish fed the L25 to L16 diet, differing by 9% in lipid content and with similar choline levels, suggests functional effects that deviate substantially from those observed in fish fed the L28 and the L25 diet, differing by 3% in lipid level and 22% in choline level. Therefore, in the following discussion, the results for the comparison of the L25 and L16 diets are considered to represent the effects of lipid level, whereas differences in the results for L28 and L25 are considered mainly to represent the effects of variation in choline level.
Effects of dietary lipid level
The observed effects of increased dietary lipid levels are related to membrane transport and signalling pathways. The effect on hypermethylation of promoter of tjap1, a peripheral tight junction protein involved in control of paracellular permeability and movement of ions, macromolecules and immune cells [Citation28,Citation29], may have contributed to the alterations in the lipid and fatty acid transport capacity in the pyloric caeca mucosa with different degrees of excessive lipid accumulation (see the results published by Siciliani et al. [Citation9]). It is commonly used as a biomarker of intestinal integrity in broilers [Citation41,Citation42].
Small non-coding microRNA, mir-212 has been observed to be a key regulator of hepatic lipid homoeostasis, suppressing multiple-target genes leading to hepatic steatosis [Citation31]. As such, the hypermethylation of the intron region of mir-212 observed with increasing dietary lipid levels may be related to the increase in steatosis symptoms with increasing lipid levels. Gene body methylation is positively correlated with gene expression rates, as it blocks the transcription of repetitive DNA elements and regulates alternative promoters and splicing [Citation43,Citation44]. The tjap1 has also been identified as a potential target of mir-212 [Citation45]. Therefore, epigenetic modulation of mir-212 could be important for L25 fed fish as an attempt to regulate lipid homoeostasis in pyloric caeca tissue with severe steatosis.
Effects of dietary choline level
The effects of increased dietary choline levels include the epigenetic regulation of several genes involved in fatty acid transport and metabolism, as summarized in .
Figure 3. Increased dietary choline levels induced epigenetic regulation of several genes involved in fatty acid (FA) transport and metabolism. Peripheral tight junction protein tjap1, solute carrier, slc22a15, and scd involved in fatty acid biosynthesis showed hypomethylation in promoter region and major facilitator superfamily transporter mfsd1 showed hypomethylation in TTS with increased dietary choline levels. Cd36 - cluster of differentiation 36, CptI and CptII - carnitine palmitoyltransferase I and II. Figure created with Biorender.com.
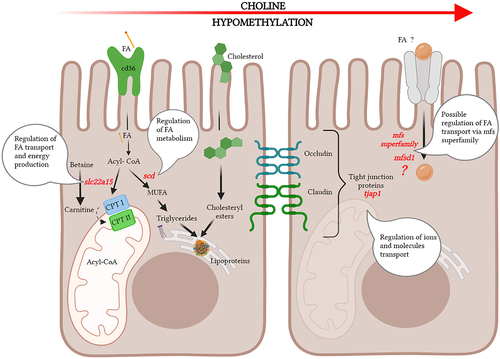
In contrast to the hypermethylation observed with the increased lipid levels and low choline levels, hypomethylation of the promoter region of tjap1 was observed with increased choline levels. Generally, methylation in promoter regions is inversely correlated with gene expression rates [Citation46], but sometimes varies with promoter sequences and gene function [Citation47]. Thus, hyper- and hypomethylation likely cause differential regulation of expression of tjap1 in respective diet fed fish. Our results indicate that the degree of methylation of the tjap1 promoter may be used as an epigenetic biomarker in pyloric caeca to assess dietary choline levels. However, our observations need to be validated further by supporting gene expression and functional studies.
Hypomethylation of the promoter of the solute carrier, slc22a15, which is important for regulating systemic and tissue levels of betaine and carnitine [Citation48], indicates that variation in choline levels has consequences for the regulation of betaine and carnitine transport. Betaine is an intermediate in the transfer of methyl groups from choline. The likely increase in the level of betaine, resulting from the elevation of choline levels, could have led to the epigenetic regulation of slc22a15, which is needed to regulate systemic and tissue levels of betaine. The effects on carnitine, which is involved in fatty acid transport, may also be underlying the observed effects of lipid levels on fatty acid levels in the intestinal mucosa [Citation49].
The hypomethylation of promoter of scd observed with increasing dietary choline level, a gene involved in regulation of the biosynthesis of monosaturated fatty acids [Citation50], could correspond to a reduction in content of monounsaturated fatty acids (MUFA) in the pyloric caeca (See the results published by Siciliani et al. [Citation9]. This observation may be related to a decrease in the severity of choline deficiency symptoms with increasing choline levels.
Among the immune genes affected by the increase in choline levels, was transcription factor irf4a, which is vital for the regulation of immune cell differentiation, including T and B lymphocytes, macrophages, and dendritic cells [Citation51]. The gene showed hypomethylation in the intron region with increasing dietary choline levels, indicating that dietary choline levels may play a role in salmon mucosal immune functions. Studies in mice have reported that irf4 is a negative regulator of inflammation in diet-induced obesity and a key regulator of lipolysis and lipogenesis [Citation52]. The dominant somatostatin receptor, sstr2a, was hypermethylated in the promoter region of L28 with increasing choline levels. Somatostatin receptors mediate the action of somatostatin, which function as an endogenous inhibitory regulator of secretory and proliferative responses of target cells [Citation53] and sstr2a reported to regulate T cell IFNγ release from inflammatory cells in mice [Citation54]. These effects support the indications discussed above regarding the involvement of choline in mucosal immune function in Atlantic salmon. Such possible effects of choline should be investigated in follow-up choline requirement studies.
Conclusion
Variation in dietary lipid levels affected the methylation of genes coding for membrane components and transporters and microRNAs important for lipid homoeostasis. Variation in choline levels affected the methylation of genes involved in fatty acid biosynthesis and transport, lipolysis and lipogenesis, and genes related to immune functions, including genes involved in the differentiation of immune cells. Among the genes affected by choline level, the tight junction protein tjap1 showed hypomethylation of the promoter with increased choline levels, indicating the possibility of its use as an epigenetic biomarker for dietary choline levels. Our observations support the vital role of choline in epigenetic regulation, as reported in higher vertebrates as well as in Atlantic salmon. These results call for research for revealing the role of choline in mucosal immune function in Atlantic salmon.
Materials and methods
Diet composition and feeding trial
The samples processed in this study were part of a larger feeding trial conducted to estimate the influence of increasing lipid levels and water temperature on the choline requirement. All details regarding the feed production and feeding trial are presented in Siciliani et al. [Citation9] and are briefly summarized below.
Three diets characterized by a high content of plant ingredients and an equally low choline level were formulated with increasing levels of lipids: 16% (L16), 25% (L25), and 28% (L28). To achieve iso-nitrogenous diets, ingredients with a low protein content were proportionally substituted with ingredients with a higher protein content in parallel with the increasing lipid level. The diets were supplemented with standard vitamins and mineral premixes and produced by extrusion. A summary of the diet compositions as analysed is presented in Supplementary Table S1. The feeding trial was conducted at Nofima’s Research Station in Sunndalsøra, Norway. Atlantic salmon with an initial weight of 25 g were raised at a temperature of 8°C using duplicate tanks per diet group.
After 8 weeks of feeding, three fish of mixed gender in the fed state were randomly sampled from each tank (n = 6 per diet group), anaesthetised with tricaine methane sulphonate (MS-222), and killed by a sharp blow to the head, according to the Norwegian Animal Welfare Act guidelines. The abdominal cavity of the fish was opened longitudinally, and the internal organ package was removed. The mesenteric fat was removed from the pyloric intestine, and a small section from the pyloric caeca was sectioned, collected in a 1.5 ml Eppendorf tube, snap-frozen in liquid N₂ and stored at −80°C.
Reduced representation bisulfite sequencing (RRBS)
DNA was extracted from 80 to 100 µg of pyloric caeca sample using an E.Z.N.A. insect DNA kit (Omega Bio-tek, Norcross, GA, USA), according to the manufacturer’s protocol. DNA quantity was assessed using a Qubit fluorometer (Invitrogen, Thermo Fisher Scientific, Waltham, MA, USA), whereas DNA quality was assessed using an Agilent 2200 TapeStation (Agilent Technologies, Santa Clara, CA, USA). Library preparation was performed using the NuGen ovation RRBS methyl-seq system 1–16 (Tecan Genomics, Inc., Redwood City, CA, USA), according to the manufacturer’s instructions. Genomic DNA was digested by incubating the samples with the MspI enzyme at 37°C for 1 h. Following adapter ligation and final repair, bisulfite conversion was performed according to the manufacturer’s instructions. The resulting bisulfite-converted libraries were subjected to desulfonation and purification and then amplified with 12 PCR cycles. The quality and quantity of the resulting RRBS libraries were assessed using the TapeStation. Two sequencing runs were performed for two pools containing equal representation from the diet groups. Sequencing was performed on an Illumina NextSeq platform (San Diego, CA, USA) using a single-end 75 bp high-throughput sequencing kit with 4% Phix control DNA (Illumina) as an internal control, following the instructions for RRBS sequencing from the NuGen RRBS protocol.
Bioinformatics analysis of RRBS data
RRBS data analysis was performed as previously described [Citation20]. Briefly, quality and diversity adapter trimming of raw data was processed using Trim Galore (Babraham Bioinformatics) without the – rrbs option and using a specific script provided by NuGen. This preserves the first base of the MspI fragment, which contains the CpG methylation measurement. Quality trimmed reads were aligned to the Atlantic salmon genome (http://www.ensembl.org, Ssal_v3.1, GCA_905237065.2) using Bismark aligner [Citation55] with directional options, and other default parameters. The percentage methylation of cytosines in CpG, CHG or CHH context were calculated by default method in Bismark aligner as follows: % methylation (context) = 100 * methylated Cs (context)/(methylated Cs (context) + unmethylated Cs (context)). Methylation information was extracted and used in the methylkit package [Citation56].
Differentially methylated cytosines in the CpG context in the genomes of Atlantic salmon between the diet groups were analysed using the methylKit package following the recommendation from the developers. Sorted SAM files from the Bismark aligner used to create a methylRaw object for CpG methylation as per the instruction from methylKit. Descriptive statistics were performed on each sample to test methylation coverage and percent methylation. Then, all the samples were filtered based on read coverage, as recommended by the methylKit, to discard bases that have coverage below 10×, and bases that have above the 99.9th percentile of coverage in each sample to avoid PCR bias. Replicate samples from each pairwise comparison were merged to one object using the unite () function with default settings in methylKit to produce bases/regions covered in all samples. DMCs were extracted by logistic regression using the statistical criteria of q-value <0.1, and methylation differences >10%. The chromosomal distribution of DMCs was also analysed for each comparison between the diet groups using the same package.
Genomic feature analysis and functional annotation of DMCs
Genomic feature analysis and annotation of DMCs were performed using the HOMER package [Citation57]. Using default parameters, annotatePeaks.pl () function was used with Salmo_salar.Ssal_v3.1.dna.primary_assembly_fa for all the known 29 chromosomes and Salmo_salar.Ssal_v3.1.108.chr.gtf from ensembl. Overlapping annotations were prioritized by HOMER in the following order: transcription start sites (TSS, including promoter regions −1 kb to +100 bp from TSS) > transcription termination sites (TTS, from −100 bp to +1 kb) > exon (coding) > 5´ UTR exon > 3´ UTR exon > CpG islands > repeats > introns > intergenic regions. HOMER identifies and assigns DMCs in intergenic regions to the gene with the nearest TSS.
Functional annotation of the genes associated with DMCs was performed using the g:Profiler online tool [Citation58] and manually inspecting the Ensembl and NCBI (https://www.ncbi.nlm.nih.gov/) databases for the respective Ensembl gene Ids resulted from HOMER. Gene ontology enrichment analysis (GO) was performed using the same tool. All the known genes of the Atlantic salmon in the Ensembl database (Ensembl 107, Ensembl genome 54) were considered, and the threshold for determining GO terms was set as a Benjamini-Hochberg FDR (False Discovery Rate) value of 0.1. The enriched GO terms were then summarized by removing redundant GO terms using the REVIGO online tool [Citation59].
Ethics statement
All experiments involving Atlantic salmon were conducted in accordance with the Norwegian Animal Research Authority guidelines.
Authors’ contributions
AD: Laboratory work, bioinformatics analysis, data interpretation, and writing of the original draft of the manuscript. DS: Sample collection, laboratory work, bioinformatics analysis, and manuscript development. TMK: Project administration and manuscript development. ÅK: Project leadership, experimental design, and manuscript development. All the authors have read, revised, and approved the manuscript.
Supplemental Material
Download Zip (86.8 KB)Acknowledgments
The authors would like to acknowledge researchers and technicians at the NOFIMA’s research station in Sunndalsøra, Norway, for conducting the feeding trials and preparation for sampling. We are also grateful to technicians Ellen Hage, Kirsti E. Præsteng and Ellen C. Valen at Nutrition and health group, Faculty of Veterinary Medicine, Norwegian University of Life Sciences (NMBU), Norway.
Disclosure statement
No potential conflict of interest was reported by the author(s).
Data availability statement
The sequence data were deposited in the NCBI Sequence Read Archive (SRA) with reference number (PRJNA992799).
Supplementary material
Supplemental data for this article can be accessed online at https://doi.org/10.1080/15592294.2024.2305079.
Additional information
Funding
References
- Bjørgen H, Li Y, Kortner TM, et al. Anatomy, immunology, digestive physiology and microbiota of the salmonid intestine: knowns and unknowns under the impact of an expanding industrialized production. Fish Shellfish Immunol. 2020;107:172–12. doi: 10.1016/j.fsi.2020.09.032
- Hardy RW. Utilization of plant proteins in fish diets: effects of global demand and supplies of fishmeal. Aquacult Res. 2010;41(5):770–776. doi: 10.1111/j.1365-2109.2009.02349.x
- Krogdahl Å, Penn M, Thorsen J, et al. Important antinutrients in plant feedstuffs for aquaculture: an update on recent findings regarding responses in salmonids. Aquacult Res. 2010;41(3):333–344. doi: 10.1111/j.1365-2109.2009.02426.x
- Krogdahl Å, Midtlyng P, Berge G, et al. GutMatters – Defining and improving intestinal health in farmed salmon. Norway: FHF - Norwegian Seafood Research Fund; 2022. ( Final Report- FHF Project 901435). https://www.fhf.no/prosjekter/prosjektbasen/901435/.
- Penn M. Lipid malabsorption in Atlantic Salmon–the recurring problem of floating feces. Fiskehelse Tekna Fiskehelseforeningen. 2011;Oslo:6–11.
- Hansen AKG, Kortner TM, Denstadli V, et al. Dose–response relationship between dietary choline and lipid accumulation in pyloric enterocytes of Atlantic salmon (salmo salar L.) in seawater. Br J Nutr. 2020;123(10):1081–1093. doi: 10.1017/S0007114520000434
- Hansen AKG, Kortner TM, Krasnov A, et al. Choline supplementation prevents diet induced gut mucosa lipid accumulation in post-smolt Atlantic salmon (Salmo salar L.). BMC Vet Res. 2020;16(1):32. doi: 10.1186/s12917-020-2252-7
- Krogdahl Å, Hansen AKG, Kortner TM, et al. Choline and phosphatidylcholine, but not methionine, cysteine, taurine and taurocholate, eliminate excessive gut mucosal lipid accumulation in Atlantic salmon (Salmo salar L). Aquaculture. 2020;528:735552. doi: 10.1016/j.aquaculture.2020.735552
- Siciliani D, Kortner TM, Berge GM, et al. Effects of dietary lipid level and environmental temperature on lipid metabolism in the intestine and liver, and choline requirement in Atlantic salmon (Salmo salar L) parr. J Nutr Sci. 2023;12:e61. doi: 10.1017/jns.2023.45
- Korsmo HW, Jiang X, Caudill MA. Choline: exploring the growing science on its benefits for moms and babies. Nutrients. 2019;11(8):1823. doi: 10.3390/nu11081823
- Ueland PM. Choline and betaine in health and disease. J Inherit Metabol Disease. 2011;34(1):3–15. doi: 10.1007/s10545-010-9088-4
- Gibellini F, Smith TK. The Kennedy pathway—De novo synthesis of phosphatidylethanolamine and phosphatidylcholine. IUBMB Life. 2010;62(6):414–428. doi: 10.1002/iub.337
- van der Veen JN, Kennelly JP, Wan S, et al. The critical role of phosphatidylcholine and phosphatidylethanolamine metabolism in health and disease. Biochim Biophys Acta - Biomembr. 2017;1859(9, Part B):1558–1572. doi: 10.1016/j.bbamem.2017.04.006
- Ridgway ND. Chapter 7 - phospholipid synthesis in Mammalian Cells. In: Ridgway ND McLeod RS, editors. Biochemistry of lipids, lipoproteins and membranes. Sixth Edition ed. Boston: Elsevier; 2016. pp. 209–236.
- Zeisel S. Choline, Other Methyl-Donors and Epigenetics. Nutrients. 2017;9(5):445. doi: 10.3390/nu9050445
- Metzger DCH, Schulte PM. Epigenomics in marine fishes. Mar Genomics. 2016;30:43–54. doi: 10.1016/j.margen.2016.01.004
- Moore LD, Le T, Fan G. DNA methylation and its basic function. Neuropsychopharmacology. 2013;38(1):23–38. doi: 10.1038/npp.2012.112
- Bird A. Perceptions of epigenetics. Nature. 2007;447(7143):396–398. doi: 10.1038/nature05913
- Amenyah SD, Hughes CF, Ward M, et al. Influence of nutrients involved in one-carbon metabolism on DNA methylation in adults—a systematic review and meta-analysis. Nutr Rev. 2020;78(8):647–666. doi: 10.1093/nutrit/nuz094
- Dhanasiri A, Chen X, Dahle D, et al. Dietary inclusion of plant ingredients induces epigenetic changes in the intestine of zebrafish. Epigenetics. 2020;15(10):1035–1051. doi: 10.1080/15592294.2020.1747777
- Anderson OS, Sant KE, Dolinoy DC. Nutrition and epigenetics: an interplay of dietary methyl donors, one-carbon metabolism and DNA methylation. J Nutr Biochem. 2012;23(8):853–859. doi: 10.1016/j.jnutbio.2012.03.003
- Dhar GA, Saha S, Mitra P, et al. DNA methylation and regulation of gene expression: guardian of our health. Nucleus. 2021;64(3):259–270. doi: 10.1007/s13237-021-00367-y
- Jones AC, Irvin MR, Claas SA, et al. Lipid phenotypes and DNA methylation: a review of the literature. Curr Atheroscler Rep. 2021;23(11):71. doi: 10.1007/s11883-021-00965-w
- Saito T, Whatmore P, Taylor JF, et al. Micronutrient supplementation affects transcriptional and epigenetic regulation of lipid metabolism in a dose-dependent manner. Epigenetics. 2021;16(11):1217–1234. doi: 10.1080/15592294.2020.1859867
- Bai ZY, Zheng H, Luo Z, et al. Dietary choline mitigates high-fat diet-impaired chylomicrons assembly via UPRer modulated by perk DNA methylation. Cells. 2022;11(23):3848. doi: 10.3390/cells11233848
- Jiang X, Greenwald E, Jack-Roberts C. Effects of choline on DNA methylation and macronutrient metabolic gene expression in in vitro models of hyperglycemia. Nutr Metab Insights. 2016;9:11–7. doi: 10.4137/NMI.S29465
- Podgorniak T, Dhanasiri A, Chen X, et al. Early fish domestication affects methylation of key genes involved in the rapid onset of the farmed phenotype. Epigenetics. 2022;17(10):1281–1298. doi: 10.1080/15592294.2021.2017554
- Rodgers LS, Beam MT, Anderson JM, et al. Epithelial barrier assembly requires coordinated activity of multiple domains of the tight junction protein ZO-1. J Cell Sci. 2013;126(7):1565–1575. doi: 10.1242/jcs.113399
- Zihni C, Mills C, Matter K, et al. Tight junctions: from simple barriers to multifunctional molecular gates. Nat Rev Mol Cell Biol. 2016;17(9):564–580. doi: 10.1038/nrm.2016.80
- Shi X, Xiang S, Cao J, et al. Kelch-like proteins: physiological functions and relationships with diseases. Pharmacol Res. 2019;148:104404. doi: 10.1016/j.phrs.2019.104404
- Hanin G, Yayon N, Tzur Y, et al. miRNA-132 induces hepatic steatosis and hyperlipidaemia by synergistic multitarget suppression. Gut. 2018;67(6):1124–1134. doi: 10.1136/gutjnl-2016-312869
- Bachmann M, Li W, Edwards MJ, et al. Voltage-Gated Potassium Channels as Regulators of Cell Death. Front Cell Dev Biol. 2020;8. doi: 10.3389/fcell.2020.611853
- Massa López D, Thelen M, Stahl F, et al. The lysosomal transporter MFSD1 is essential for liver homeostasis and critically depends on its accessory subunit GLMP. Elife. 2019;8. doi: 10.7554/eLife.50025
- He H, Huang J, Wu S, et al. The roles of GTPase-activating proteins in regulated cell death and tumor immunity. J Hematol Oncol. 2021;14(1):171. doi: 10.1186/s13045-021-01184-1
- Honda A, Nogami M, Yokozeki T, et al. Phosphatidylinositol 4-phosphate 5-kinase α is a downstream effector of the small G protein ARF6 in membrane ruffle formation. Cell. 1999;99(5):521–532. doi: 10.1016/S0092-8674(00)81540-8
- Miura Y, Hongu T, Yamauchi Y, et al. ACAP3 regulates neurite outgrowth through its GAP activity specific to Arf6 in mouse hippocampal neurons. Biochem J. 2016;473(17):2591–2602. doi: 10.1042/BCJ20160183
- Miura Y, Kanaho Y. ACAP3, the GTPase-activating protein specific to the small GTPase Arf6, regulates neuronal migration in the developing cerebral cortex. Biochem Biophys Res Commun. 2017;493(2):1089–1094. doi: 10.1016/j.bbrc.2017.09.076
- Oku Y, Huganir RL. AGAP3 and Arf6 regulate trafficking of AMPA receptors and synaptic plasticity. J Neurosci. 2013;33(31):12586–98. doi: 10.1523/JNEUROSCI.0341-13.2013
- Fan X, Jin WY, Wang YT. The NMDA receptor complex: a multifunctional machine at the glutamatergic synapse. Front Cell Neurosci. 2014;8:160. doi: 10.3389/fncel.2014.00160
- Skjærven KH, Jakt LM, Fernandes JMO, et al. Parental micronutrient deficiency distorts liver DNA methylation and expression of lipid genes associated with a fatty-liver-like phenotype in offspring. Sci Rep. 2018;8(1):3055. doi: 10.1038/s41598-018-21211-5
- Santos RR, Ooosterveer-van der Doelen MAM, Tersteeg-Zijderveld MHG, et al. Induction of gut leakage in young broiler chickens fed a diet with low rye inclusion. Heliyon. 2021;7(12):e08547. doi: 10.1016/j.heliyon.2021.e08547
- Slawinska A, Dunislawska A, Plowiec A, et al. Modulation of microbial communities and mucosal gene expression in chicken intestines after galactooligosaccharides delivery in ovo. PloS One. 2019;14(2):e0212318. doi: 10.1371/journal.pone.0212318
- Jjingo D, Conley AB, Yi SV, et al. On the presence and role of human gene-body DNA methylation. Oncotarget. 2012;3(4):462–74. doi: 10.18632/oncotarget.497
- Maunakea AK, Nagarajan RP, Bilenky M, et al. Conserved role of intragenic DNA methylation in regulating alternative promoters. Nature. 2010;466(7303):253–257. doi: 10.1038/nature09165
- Burek M, König A, Lang M, et al. Hypoxia-induced MicroRNA-212/132 alter blood-brain barrier integrity through inhibition of tight junction-associated proteins in human and mouse brain microvascular endothelial cells. Transl Stroke Res. 2019;10(6):672–683. doi: 10.1007/s12975-018-0683-2
- Jones PA. Functions of DNA methylation: islands, start sites, gene bodies and beyond. Nat Rev Genet. 2012;13(7):484–492. doi: 10.1038/nrg3230
- Weber M, Hellmann I, Stadler MB, et al. Distribution, silencing potential and evolutionary impact of promoter DNA methylation in the human genome. Nat Genet. 2007;39(4):457–66. doi: 10.1038/ng1990
- Yee SW, Buitrago D, Stecula A, et al. Deorphaning a solute carrier 22 family member, SLC22A15, through functional genomic studies. FASEB J. 2020;34(12):15734–15752. doi: 10.1096/fj.202001497R
- Longo N, Frigeni M, Pasquali M. Carnitine transport and fatty acid oxidation. Biochim Biophys Acta, Mol Cell Res. 2016;1863(10):2422–2435. doi: 10.1016/j.bbamcr.2016.01.023
- Paton CM, Ntambi JM. Biochemical and physiological function of stearoyl-CoA desaturase. Am J Physiol Endocrinol Metab. 2009;297(1):E28–E37. doi: 10.1152/ajpendo.90897.2008
- Nam S, Lim J-S. Essential role of interferon regulatory factor 4 (IRF4) in immune cell development. Arch Pharm Res. 2016;39(11):1548–1555. doi: 10.1007/s12272-016-0854-1
- Eguchi J, Wang X, Yu S, et al. Transcriptional control of adipose lipid handling by IRF4. Cell Metab. 2011;13(3):249–259. doi: 10.1016/j.cmet.2011.02.005
- Patel YC. Somatostatin and its receptor family. Front Neuroendocrinol. 1999;20(3):157–198. doi: 10.1006/frne.1999.0183
- Elliott DE, Li J, Blum AM, et al. SSTR2A is the dominant somatostatin receptor subtype expressed by inflammatory cells, is widely expressed and directly regulates T cell IFN-γ release. Eur J Immunol. 1999;29(8):2454–2463. doi: 10.1002/(SICI)1521-4141(199908)29:08<2454:AID-IMMU2454>3.0.CO;2-H
- Krueger F, Andrews SR. Bismark: a flexible aligner and methylation caller for bisulfite-seq applications. Bioinformatics. 2011;27(11):1571–2. doi: 10.1093/bioinformatics/btr167
- Akalin A, Kormaksson M, Li S, et al. methylKit: a comprehensive R package for the analysis of genome-wide DNA methylation profiles. Genome Biol. 2012;13(10):R87. doi: 10.1186/gb-2012-13-10-r87
- Heinz S, Benner C, Spann N, et al. Simple combinations of lineage-determining transcription factors prime cis-regulatory elements required for macrophage and B cell identities. Mol Cell. 2010;38(4):576–89. doi: 10.1016/j.molcel.2010.05.004
- Raudvere U, Kolberg L, Kuzmin I, et al. G: profiler: a web server for functional enrichment analysis and conversions of gene lists (2019 update). Nucleic Acids Res. 2019;47(W1):W191–W198. doi: 10.1093/nar/gkz369
- Supek F, Bošnjak M, Škunca N, et al. REVIGO summarizes and visualizes long lists of gene ontology terms. PLoS One. 2011;6(7):e21800. doi: 10.1371/journal.pone.0021800