ABSTRACT
When synchronized with the light/dark cycle the circadian rhythm is termed a diurnal rhythm and this organizes an organism’s daily life cycle in relation to the metabolic shifts during the day/night cycles. This is a complex task, particularly under stress conditions. Accurate maintenance of the diurnal rhythm becomes an issue under environmental extremes, such as drought due to the impairment of metabolism, redox balance, and structural integrity. In plants, the non-proteinogenic amino acid GABA accumulates to high levels in response to several stress factors but this is not always dependent on the activation of its biosynthesis. Here we propose a regulatory role to GABA during the diurnal rhythm in plants which is similar to its function in animals where it adjusts the circadian rhythm. Here we investigated whether GABA-biosynthesis was affected by drought stress during the diurnal cycle. For this, we took samples from leaves of N. tabacum plants subjected to PEG-mediated drought stress (−0.73 MPa) during the day and night cycle during a 24 hour period. Glutamate, GABA, and proline contents, along with GDH, GAD enzyme activities and transcript profiles were analyzed. Overall, we conclude that the oscillations in GABA biosynthesis during day and night cycle have an impact on drought stress responses which needs to be elucidated by further analysis.
Introduction
Drought stress is a serious environmental constraint for sustainable agriculture practices affecting crop quality and yield worldwide. Plants can adapt to or avoid drought by controlling stomatal movements or by deep rooting, which are all costly processes, especially under limited photosynthetic capacity. Adjustments at the leaf level should be followed by adaptations at the whole plant level in order to continue to growth and development under drought conditions.Citation1,Citation2 When plants are exposed to drought stomatal response, metabolism, and photosynthesis are all affected leading to decreased growth rates in order to survival.Citation3 Survival at the expense of growth in terms of drought tolerance is generally not favored for agricultural production; therefore, the selection of unique strategies used by xerophytes or resurrection plants is not feasible for crop plants.
The response time to water stress is important even in drought-tolerant plants, for example, on exposure to water stress, leaf and root elongation are reduced within hours while the rate of leaf emergence decreases within days.Citation4 Accordingly, features such as biological and circadian clocks also act on plants' responses to different environmental factors that become significant in terms of stress tolerance.Citation5 Light/dark cycles are sensed by organisms’ circadian clock which can also be reset by environmental inputs, such as light and temperature.Citation6,Citation7 This clock coordinates an organism’s internal physiology and behavior with the external environment through a coordinated network and is known to be permanent at constant environmental conditions.Citation8,Citation9
The circadian rhythm influences several physiological processes, such as photosynthesis, stomatal and leaf movement, and stem extension in plants.Citation10 Accordingly, almost one-third of the Arabidopsis thaliana genome was found out to be controlled by the circadian rhythm.Citation10,Citation11 The circadian rhythm has tissue specificity and is controlled by environmental inputs; however, we still do not know how the timing is controlled across the plant and what type of long-distance signaling molecules are involved.Citation10,Citation12 Circadian rhythms can be “diurnal” and “free running”; the former one is usually defined as the circadian rhythm that is synchronized with the day and night cycle within 24 h while, the latter one is a type of circadian rhythm with an endogenous period without time-cues.Citation13,Citation14 Diurnal rhythm affects how plants respond and cope with environmental stresses as shown in several studies.Citation5 Accordingly, abscisic acid (ABA) signaling and drought-responsive genes were found out to be regulated by diurnal rhythm in A. thaliana.Citation15 However, discrepancies in the efficiency of nutrient uptake and stomatal conductance in maize (Zea mays) and rice (Oryza sativa) as a consequence of diurnal variations have also been reported.Citation5,Citation16,Citation17 In rice, a reciprocal relationship was proposed between transcript abundances of salt overly sensitive (SOS) pathway related genes and diurnal rhythm.Citation18 Moreover, growth and ethanol production in submerged rice roots exhibited fluctuations during day and night cycles as compared to non-submerged ones.Citation19 In a recent study, drought response in rice was found out to be diurnally regulated with genome-wide analysis revealing that more than half of the up and down-regulated genes were synchronized to the diurnal rhythm.Citation5
The circadian rhythm in mammals is regulated by neurons and neurotransmitter molecules found in hypothalamic suprachiasmatic nucleus (SCN).Citation20,Citation21 Gamma amino butyric acid (GABA) is among the neurotransmitters recently reported to have a critical role in refining the circadian rhythm in mice.Citation21 GABA is a non-proteinogenic amino acid that is primarily synthesized from glutamate by the activity of glutamate decarboxylase (GAD).Citation22,Citation23 In plants, GABA is found in all organs at different concentrations. Hence, diverse functions have been predicted and proven for GABA ranging from it being a primary metabolite to a signaling molecule.Citation24 Rapid accumulation of GABA either through increased GAD activity or by polyamine catabolism has been reported in different plants under abiotic and biotic stresses.Citation25–29 Under drought stress conditions ABA accumulation lead to increased Ca+2 levels and Ca2+ activated GAD for GABA biosynthesis.Citation23–30
The idea of GABA as a signaling molecule in plants arises from two observations; first, it functions as a neurotransmitter inhibitor with very well-defined receptors (GABAergic) in mammals, and second, upon exposure to different abiotic and biotic stresses GABA levels sharply increase in plant tissues. Until now no clear evidence for any protein interacting solely and directly with GABA as a receptor has been found. However, aluminum tolerance associated transporters – aluminium‐activated malate transporters (ALMTs) – may be the exception. ALMTs were proposed as the first protein family identified to interact directly with GABA in plants.Citation31,Citation32 Furthermore, a new study provided evidence that GABA caused a conformational change in Triticum aestivum ALMT1 (TaALMT1) rather than competitive inhibition which reduced anion channel opening frequency.Citation33 Accordingly, Mekonnen et al.Citation34 attributed GABA to a stress-specific role as a signaling molecule for the regulation of stomatal movement by binding to ALMT proteins and interacting with H+ATPase. GABA, as a signaling molecule in plants is still under debate and several questions remains to be addressed. As indicated by Li et al.Citation35 how GABA levels are sensed and its interaction with reactive oxygen species (ROS) are unknown, while different signaling pathways of GABA might also be operating in plants. On the other hand, intercellular communication through GABA gradients affecting growth and development especially under stress conditions is also yet to be answered.Citation35,Citation36
In light of the above studies, we hypothesized that GABA may be responsible for controlling the circadian rhythm in plants as it does in animals. Perhaps this would explain its rapid accumulation under various stress conditions, as continuation of the daily rhythm is necessary to maintain cellular homeostasis. Therefore, in this study, using tobacco (Nicotiana tabacum L.), we conducted experiments to understand whether diurnal rhythm has an effect on GABA biosynthesis under ambient and drought stress conditions. This is the first report on the effect of diurnal rhythm on the cytosolic part of the GABA shunt. The differences during the day and night cycles were analyzed and compared between control and drought-stressed plants in N. tabacum.
Results
Growth and physiological parameters
Drought stress was performed using 20% Polyethylene glycol (PEG) 6000 which was equivalent to an osmotic potential of −0.73 MPa. The differences in relative water content (RWC), membrane ion leakage, photosynthetic efficiency and root dry weights were analyzed in control and drought-stressed groups on the 1st and 2nd days of the PEG treatment (). Since we were interested in the effect of day/night cycle on GABA-related responses under drought stress we analyzed growth and physiological parameters at 24 h intervals following exposure to stress. Overall, there were no significant differences between control and drought-stressed groups on both days except that of membrane ion leakage which increased in stressed plants on day 2 (). RWC, photosynthetic efficiency, and root dry weight were not significantly different between the control and PEG-treated groups ().
Differences in proline content
Proline levels differed between the control and drought-stressed group (). During the day cycle, proline levels in the control were higher than in drought-stressed plants. In the last hour of the photoperiod (16 h) proline levels were 3 fold higher in the control compared to the drought-stressed group in which levels had decreased between hours 12–16. However, within the first hour (17th hour of complete photoperiod) of the dark cycle this situation was changed. Proline levels increased (almost 2-folds) in drought-stressed plants and decreased in the control group. For the rest of the night cycle to the end of the first 24 h period, proline levels were similar in both groups (). Proline accumulated to the highest level of the experimental period in drought-stressed plants on day 2 which might be a possible indicator for the onset of its osmo-protective role.
Glutamate content and glutamate dehydrogenase (GDH)
We analyzed glutamate content and GDH activity in the first 24 hours of PEG-induced drought stress (). In drought-stressed groups glutamate level was similar to control groups during the day cycle (between 0 and 16th hours). Glutamate levels increased in both groups at the 1st hour of the night cycle however, it was decreased in the following dark-period in the control group. In drought-stressed group, glutamate levels increased following the 3rd hour of the night cycle although the values were not higher than that of neither the control group nor the first hour of the night cycle. GDH activities were changed significantly in both groups at the last hour of the day cycle and following the night cycle. The increased GDH activity was more remarkable in drought-stressed group which had a clear fluctuation during the night cycle. Even the decreased values were higher than that of its day cycle values and overall control levels. Transcript abundance of GDH was up-regulated starting from the 1st hour of drought stress treatment and highest GDH transcript abundance was found at the 3rd hr. Although GDH transcript abundance was higher in PEG-treated groups than in the control groups there was a difference between day and night cycles. Induction of GDH transcript abundance was at a steady-state level during the night cycle while it was enhanced during the day cycle ().
Figure 3. Glutamate content (µmol g FW−1) and GDH activity (µmol min mg protein−1) and relative transcript abundances (fold change) of GDH encoding gene in control and PEG-treated N. tabaccum plants at day/night cycle, 24 and 48 hours. Values presented are the mean ± SE (n = 6) of two independent experimental series. *p < .05 and **p < .01
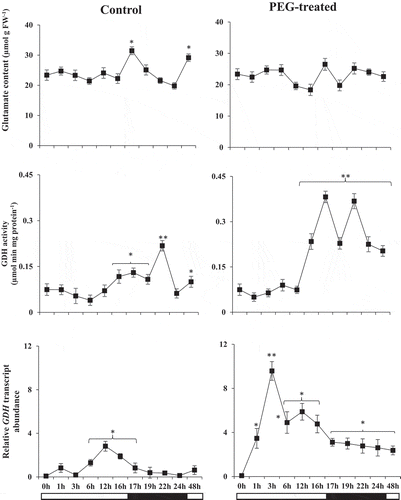
GABA content and glutamate decarboxylase (GAD)
The first step in the GABA shunt (cytosolic GABA biosynthesis) is catalyzed by GAD. We compared GABA content and GAD enzyme activity in control and PEG-treated groups (). GABA levels increased sharply after the first hour of drought stress at the day-cycle. This level was 2-fold greater than the control group and did not change until the beginning of the night-cycle. GAD activity in control and drought-stressed plants exhibited a similar trend during the day cycle which had the highest activity at the 3rd hour in both groups. GAD activity was higher in PEG-treated groups at the first hour (17th hour) of the night-cycle compared to control levels and it increased more than 3-fold by the 6th hour of the night-cycle (). Moreover, in drought-stressed group there was a steep decline in GAD activity at the end of the night cycle. GABA levels were slightly higher than control groups during the night cycle which was not correlated with differences in GAD activity. Increased GAD activity followed by GABA accumulation in the control group while it was not the case in drought-stressed group on day 2. When we compare GAD transcript abundance, rapid transcriptional activation was seen in the drought-stressed groups as compared to control groups during the day cycle (). The highest GAD transcript abundance was seen at the 12th hour in the PEG treated groups. Even the declined transcript levels in drought-stressed groups remained higher than that of control groups at the night cycle. Highest GAD transcript level was found at the 1st hour of the day cycle and last hours of the night cycle in control groups which were lower than that of drought-stressed groups ().
Figure 4. GABA content (µmol g FW−1), GAD activity (µmol min mg protein−1) and relative transcript abundances (fold change) of GAD encoding gene in control and PEG-treated N. tabaccum plants at day/night cycle, 24 and 48 hours. Values presented are the mean ± SE (n = 6) of two independent experimental series. *p < .05 and **p < .01
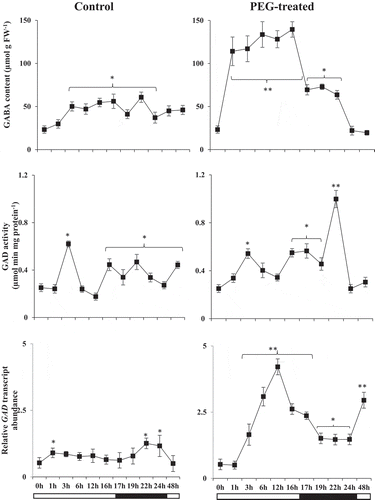
Discussion
The proof of GABA’s role as a signaling molecule is a hot topic of GABA and GABA-shunt-related research in plants. To date, besides ALMTs, no GABA receptor as a counterpart to animal homologs has been identified. There are serious questions yet to be answered such as why GABA is found in all plant tissues and how its biosynthesis and degradation are tightly controlled. For instance, disrupting GABA degradation leads to infertility and abnormal pollen tube growth and high levels also caused distorted development of female tissues.Citation37 On the other hand, maintenance of adequate GABA levels is also important for pollen tube guidance.Citation37 Recently, regulation of circadian rhythm was found to be regulated by GABA in miceCitation21 which might also be the case in plants.
Here, we focused on how GABA shunt’s first step components were affected by PEG-mediated drought stress during the diurnal rhythm (day and night cycle). We considered that elucidation of how GABA levels and its primary biosynthetic route were affected by the diurnal rhythm should be important before attributing to GABA a regulatory role in the circadian rhythm. Root growth and physiological parameters measured to determine short-term effects of drought showed no differences between control and stress groups. Therefore, the changes in GABA metabolism related biochemical processes and transcriptional activation took place long before observable changes in morphological and physiological characteristics in the first phase of PEG-induced drought stress. The effect of drought stress on plants varies according to its severity and duration. Drought responsive genes expressions varies with time of the day under mild-drought conditions in A. thaliana.Citation38 Roots are less affected by drought as compared to leaves and a steady but reduced elongation takes place within days to weeks of the stress treatment.Citation4 Leaf growth is suppressed as the first response to growth leading to long-term yield losses.Citation39 In A. thaliana inhibition of growth by drought stress was more pronounced during the day time and related to the circadian clock.Citation38
GABA shunt’s first step takes place in the cytosol where glutamate is formed by the activity of GDH and GABA is synthesized by GAD.Citation26 We found a clear discrepancy between day and night cycles with regards to GDH and GAD activities and their transcript abundances which were generally induced by drought stress. We propose that GDH and GAD-related biosynthetic route is regulated differentially through day/night cycle which was more remarkable at the first 24 h of the drought stress. Increased GABA levels in drought-stressed plants were well correlated with increased GAD transcript abundance during the day cycle which was not the case for control groups. Although GABA biosynthesis may occur by other routes (polyamine catabolism and proline conversion) in plants the main route takes place in the cytosol by the activity of GAD which takes place at the cost of glutamate.Citation23,Citation40 However, as indicated by Shelp et al., Citation23 high GABA levels under stress are related to glutamate and GAD activity as the alternative routes (diamine oxidase and 4-aminobutyraldehyde dehydrogenase) are inhibited by abiotic stress.
According to overall findings, we speculate that drought stress induces transcriptional reprogramming of GABA biosynthesis during the day cycle which might also be regulated by circadian clock genes; hence, further detailed analysis is necessary especially by the mutagenesis approach. As indicated previously, GABA accumulates to high levels when plants are exposed to stresses, such as temperature shocks (either cold or heat), mechanical wounding, hypoxia, etc., which was not always followed by increased GAD activity.Citation41 It is important to distinguish whether this high level is a consequence of induced biosynthetic routes or due to the impairment in GABA-shunt under stress conditions.Citation42 High activities and induced transcript levels of GDH did not result in glutamate accumulation in drought-stressed plants which was more remarkable during the night-cycle. GABA levels between the first and last hours of the night-cycle were lower than that of day-cycle levels however, even these decreased values were still higher than in the control. Masclaux-Daubresse et al.Citation43 reported day/night fluctuations in GABA and glutamate contents of tobacco leaf disc which were taken from four month old plants at the flowering stage. They found that oscillations in GABA content were parallel to glutamate levels which were not in accordance with GDH transcript abundance in source leaves. Their conclusion was; a buffering role for GABA which might also be the case in our study. High GABA levels at the day cycle during drought stress exposure would be necessary for keeping glutamate levels constant. However, we found increased GDH transcript levels during the day cycle was followed by increased GDH enzyme activity at the night cycle in drought-stressed groups.
Glutamate is an important amino acid and metabolite precursor hence the glutamate pool is tightly controlled.Citation34 GDH activity is not the only route for glutamate biosynthesis in plants. Glutamate is also synthesized by two other enzymes; glutamine synthetase and glutamate synthase.Citation44 In our study, these two enzymes could be the major contributors to glutamate biosynthesis rather than GDH in control plants. Stress-induced GDH activity has been reported in tobacco and grapevine.Citation44 Accordingly, similar to our findings, Forde and LeaCitation44 emphasized that the diurnal fluctuations were small in leaf glutamate levels as compared to other amino acid levels. Moreover, higher GDH activities during the night-cycle are also a common feature for both control and drought-stressed plants which are not transcriptionally regulated. Radioactive tracer studies provided evidence for glutamate-induced GABA biosynthesis in the short-term while, inhibitory effects occur as GABA levels increases in the long term under normal conditions.Citation45 This can be an explanation for our findings in relation to steady-state levels of GABA and glutamate in control plants.
Like glutamate, the essential amino acid proline is also found at high levels in plants exposed to abiotic and biotic stress. Osmoprotective function, free radical scavenging capacity, and stabilization of membranes are among the roles attributed to proline and it was proposed as a metabolic signal regulating metabolic pools and redox balance.Citation46 These protective effects of proline take place days after stress exposure; hence, here we focused on how proline levels changed diurnally in control, and drought-stressed plants. In the first day and night cycle, proline levels in drought-stressed plants were lower or similar to control values. Light activates proline biosynthesis, with local increases in proline levels related to flowering while, dark induces its catabolism.Citation46,Citation47 The drought-induced high proline level (almost 3-folds higher) was found to be sufficient to maintain RWC at control levels on day 2 since, the onset of its osmo-protective function might occurred after 24 hours. Proline accumulation in plants under drought stress is well documented.Citation48–50 In these studies along with better leaf water status, an osmoprotective role was attributed to proline. A non-enzymatic antioxidative role was also suggested as proline has hydroxyl radical (•OH) scavenger activity.Citation40,Citation51 Under oxidative stress favoring conditions •OH-attack on the N atom of proline can lead to the formation of GABA.Citation40 Accordingly, in the first day cycle of the stress treatment, this additional non-enzymatic GABA formation through proline could also contribute to GABA accumulation, since proline levels were decreased in drought-stressed groups.
Despite the vast amount of published data related to GABA and GABA-shunt there are still missing parts of the puzzle as indicated by Fromm.Citation24 Based on our findings presented in this study we suggest that the regulatory role or contribution of GABA to diurnal rhythm should also be taken into account. Yet, further studies are necessary to understand how circadian rhythm related genes, especially transcription factors are interacting with GABA and GABA shunt components.
Materials and methods
Plant growth and drought stress treatment
Nicotiana tabacum seeds were provided by Aegean Agricultural Research Institute-Menemen, Izmir (AARI). Seedlings were transferred into pots filled with perlite after germination and grown under controlled conditions (16/8h light/dark photoperiod at 25/20°C, relative humidity of 60–70%, and photosynthetic photon flux density of 350 μmol m−2 s−1) and watered regularly with ½ strength Hoagland solution. When seedlings were 45 days old they were separated into two groups (control and PEG-treated) and each group contained 40 plants. For drought stress treatment, PEG 6000 20% (w/v) was added into ½ strength Hoagland solution which was equivalent to an osmotic potential of −0.73 MPa. The fully developed 3rd leaf was taken for sampling from control and PEG treated groups at the 0, 1, 3, 6, 12 and 16th hours of the day cycle and 1, 3 and 6th hours of night cycle for all analysis except that of RWC, membrane leakage, Fv/Fm and root dry weight. Leaf samples were also taken on the 1st (24 h) and 2nd (48 h) days of drought stress treatment for all analysis. Two independent trials were conducted and per trial five biological replicates (n = 10) for RWC, membrane leakage, Fv/Fm and root dry weight analysis and three biological replicates (n = 6) for other analysis were sampled randomly. RWC, membrane leakage, Fv/Fm and root dry weight analysis were performed on the sampling day and other samples were fresh frozen in liquid nitrogen and stored at −80°C.
Root dry weight
Seedlings from each treatment were randomly sampled on days 0, 1 and 2 days (n = 10). Roots were carefully separated, excised and oven dried at 70°C for 72 h. After 72 h, they were weighed and recorded as dry weight values.
Relative water content (RWC)
RWC values of the leaves were determined according to Smart and BinghamCitation52 by the formula RWC% = [(FW−DW)/(TW−DW)]×100. Fresh weights (FW) were recorded immediately after sampling and for turgid weights (TW) leaves were submerged in dH2O for 5 h at room temperature. After measuring turgid weights leaves were dried at 70°C for 72 h and their dry weights (DW) were also recorded (n = 10).
Membrane ion leakage determination
Membrane ion leakage levels in control and drought-stressed groups were measured using a WTW Inolab Conductivity Meter 720. Leaf discs of 0.6 cm2 were excised from each group and “X0” values were measured. The same discs were then immersed in 20 ml dH2O for 2 h for the determination of “Xi” values. For “Xt” values leaf discs were kept at 80°C for 2 h (n = 10). Membrane ion leakage levels were calculated by using the ion conductivity values at each point and calculated according to the formula; [(Xi–X0)/(Xt−X0)]×100.Citation53
Measurement of chlorophyll fluorescence
Leaf chlorophyll fluorescence analysis used a Hansatech Plant Efficiency Analyzer (Norfolk, UK). Excised leaves from control and PEG-treated plants were dark adapted for 30 min (n = 10). The basal non-variable chlorophyll fluorescence level (F0), the maximal fluorescence induction (Fm), variable fluorescence (Fv) and the ratio of Fv/Fm were determined.
Determination of proline content
Proline contents in control and drought-stressed groups were determined according to the Bates et al.Citation54 Leaf samples (0.1 g) were extracted in 3% (w/v) aqueous sulphosalycylic acid and extracts were filtered through Whatman filter paper (n = 6). Glacial acetic acid and acid-ninhydrine solutions were added to filtrated extracts and the mixture was incubated at 95°C for 1 hour. Samples were cooled to room temperature before the addition of toluene. The absorbance value of the toluene aspired fraction was monitored at 520 nm. Proline contents (µmol proline g FW−1) were determined by using a calibration curve.
Glutamate dehydrogenase (GDH) and glutamate decarboxylase (GAD) activity assays
Leaf samples (1 g) were ground in liquid nitrogen and homogenized in 5 mM EDTA, 0.1 mM Tris-HCl, 10 mM DTT, 1 mM pyridoxal-5-phosphate hydrate, and 1% (w/v) PVPP (pH 7.8) homogenization buffer at +4°C. After centrifugation at 10.000g for 10 min at +4°C, supernatants were desalted using a Sephadex G-50 column. Desalted homogenates were used for protein content, GDH and GAD activity assays (n = 6). Protein contents of the samples were determined according to BradfordCitation55 using bovine serum albumin as the standard. GDH activity was determined according to Akihiro et al.Citation56 Desalted homogenate was mixed with the reaction mixture (50 mm (NH4)2SO4, 13 mM α-ketoglutarate, 0.25 mM NADPH and 1 mM CaCl2, 100 mM Tris-HCl buffer, pH 8.0) and the differences in absorbance values were determined at 340 nm for 1 min. GDH activity was expressed as μmol min−1 mg protein−1. GAD activity analysis was done according to Bartyzel et al.Citation57 with some modifications. Desalted homogenates were pre-incubated in the reaction mixture (20 μm pyridoxal phosphate, 50 mM K-phosphate, pH 5.8) at 30°C for 10 min. Decarboxylation reaction was started by the addition of 3 mM L-glutamate to the mixture which was incubated at 30°C for 1 h. After incubation reaction was stopped by the addition of 0.5 M HCl solution and mixture was centrifuged at 12.500 g for 10 min. Samples were derivatized using a ninhidrine solution of 0.35% (w/v). GABA content of the samples was determined spectrophotometrically by compared with the standard curve. GAD enzyme activity was expressed as μmol min−1 mg protein−1. All spectrophotometric analyses were conducted on a Shimadzu (UV-1600) spectrophotometer.
Glutamate and GABA content determinations
Glutamate (K-Glu 03/06) assay kit (Megazyme, Ireland) was used for the determination of glutamate content of the samples. Sample extraction was carried out by using the enzyme extraction method used above and glutamate contents (μmol g FW−1) were determined spectrophotometrically according to the manufacture’s protocol (n = 6). GABA contents were determined by HPLC (Shimadzu-VP101, Japan). GABA extraction was done according to Baum et al.Citation58 and HPLC analysis was performed according to Bor et al.Citation59 Leaf samples (0.5 g) from control and drought-stressed plants were ground in liquid nitrogen and then extracted with water: chloroform: methanol (3:5:12 v/v/v) solution. Extracts were centrifuged at 10,000g for 2 min. Supernatants were dried o/n and re-dissolved in derivatization solution (dH2O, borax buffer (pH 8.0) and 2-hydroxynaphthaldehyde (HN) 0.3% w/v in methanol). After incubation at 80°C for 20 min, samples were cooled and diluted with methanol before they were filtrated through 0.45 μm sample filter. For HPLC analysis isocratic elution was used with methanol: water (62:38 v/v) mobile phase at a flow rate of 0.5 mL min−1. Separation through Supelco LC18; 250 × 4.6 mm, 2. 5 μm column with the sample injection volume of 20 μL and detection at 330 nm (UV-detector) were performed. The retention time for GABA was 12 min in a total elution of 20 min. GABA contents (μmol g FW−1) were quantified by using a standard curve (n = 6).
Quantitative PCR analysis
Differences in the transcript abundances of GAD and GDH encoding genes were analyzed and compared by real-time PCR using Actin as the internal standard. Total RNA was extracted from the leaf samples (100 mg) of control and drought-stressed plants using the NucleoSpin RNA Plant Kit (Macherey-Nagel). Before cDNA synthesis, the quality and integrity of RNAs were controlled on EtBr gel and quantified by BioPhotometer UV/Vis Spectrophotometer (Eppendorf). Protoscript II cDNA synthesis kit (New England Biolabs) was used for reverse transcription of RNAs (10 μg). 10-fold diluted cDNA from each sample was used for real-time PCR reaction. Gene-specific primers for GAD, GDH and Actin encoding genes were designed using Primer-Blast (http://www.ncbi.nlm.nih.gov/tools/primer-blast/) and primer sequences presented in . The expressions of GAD and GDH were normalized to Actin. Real-yime q-PCR reactions were performed by Applied Biosystems (ABI) Step One Plus Real time PCR system with the SYBR Green PCR master mix (Applied Biosystems). The total volume of the reaction mixture was 20 μL (5 μL of cDNA, 15 μL PCR master mix). Reactions were initiated by the activation of the enzyme at 95°C for 10 min, followed by 40 cycles of: 95°C for 15 s, 60°C for 1 min and final extension at 72°C for 1 min. The resulting Ct values were used to calculate the relative transcript abundance according to the standard curve method by Step One Plus Software.
Table 1. List of the primer sequences used in qPCR analysis
Statistical analysis
All analyses were done on a completely randomized design and the data obtained were subjected to one-way analysis of variance (ANOVA). The mean differences were compared by the lowest standard deviations (LSD) test within the control and drought-stressed groups. Each data point was the mean of six replicates (n = 6) except that of RWC, membrane leakage, Fv/Fm and root dry weight analysis (n = 10). Comparisons with P values <.05 and <0.01 were considered significantly different. In all the figures the error bars represent the standard errors of the means.
Author contributions
MB and FO conceived the research plans and designed the experiments; AP, SY, and MB performed the experiments; MB and IT wrote the article. All authors read and approved the manuscript.
Acknowledgments
The authors thank to Prof. Steven Footitt for his critical reading and helpful contributions to the manuscript. This work was supported by grant-in-aid 14-FEN-045 from Ege University Research Foundation.
Disclosure statement
The authors declare that they have no conflicts of interest.
References
- Chaves MM, Maroco JP, Pereira JS. Understanding plant responses to drought – from genes to the whole plant. Functional Plant Biology. 2003;30(3):1–9. doi:10.1071/FP02076.
- Galle A, Florez-Sarasa I, Thameur A, De Paepe R, Flexas J, Ribas-Carbo M. Effects of drought stress and subsequent rewatering on photosynthetic and respiratory pathways in Nicotiana sylvestris wild type and the mitochondrial complex I-deficient CMSII mutant. JXB. 2010;61(3):765–775.
- Osakabe Y, Osakabe K, Shinozaki KP, Tran LSP. Response of plants to water stress. Front Plant Sci. 2014;5(86):1–8. doi:10.3389/fpls.2014.00086.
- Munns R. Comparative physiology of salt and water stress. Plant, Cell & Environment. 2002;25(2):239–250. doi:10.1046/j.0016-8025.2001.00808.x.
- Kim S-W, Lee S-K, Jeong H-J, An G, Jeon J-S, Jung K-H. Crosstalk between diurnal rhythm and water stress reveals an altered primary carbon flux into soluble sugars in drought-treated rice leaves. Sci Rep. 2017;7(1):8214. doi:10.1038/s41598-017-08473-1.
- Kuhlman SJ, Craig LM, Duffy JF. Introduction to Chronobiology. Cold Spring Harbor Perspectives in Biology. 2018;10(9):a033613. doi:10.1101/cshperspect.a033613.
- Farre EM. The brown clock: circadian rhythms in stramenopiles. Physiologia Plantarum. 2020;169(3):430–441. doi:10.1111/ppl.13104.
- De Mairan J. Observation botanique. Hist Acad Royal Sci. 1729;35–36.
- Huang H, Nusinow DA. Into the Evening: complex interactions in the Arabidopsis circadian clock. Trends in Genetics. 2016;32(10):674–686. doi:10.1016/j.tig.2016.08.002.
- Nimmo HG, Lairda J, Bindbeutel R, Nusinow DA. The evening complex is central to the difference between the circadian clocks of Arabidopsis thaliana shoots and roots. Physiologia Plantarum. 2020;169(3):442–451. doi:10.1111/ppl.13108.
- Covington MF, Maloof JN, Straume M, Kay SA, Harmer SL. Global transcriptome analysis reveals circadian regulation of key pathways in plant growth and development. Genome Biology. 2008;9(8):130. doi:10.1186/gb-2008-9-8-r130.
- Endo M. Tissue-specific circadian clocks in plants. Current Opinion in Plant Biology. 2016;29:44–49. doi:10.1016/j.pbi.2015.11.003.
- Vitaterna MH, Takahashi JS, Turek FW. Overview of circadian rhythms.. Alcohol Res Health. 2001;25:85–93.
- McHill AW, Butler MP, Shea SA, Cycle F-R. Vonk J. Cham: Shackelford T. (eds) Encyc Animal Cogn Behav. Switzerland: Springer; 2018.
- Wilkins O, Bräutigam K, Campbell MM. Time of day shapes Arabidopsis drought transcriptomes. The Plant Journal. 2010;63(5):715–727. doi:10.1111/j.1365-313X.2010.04274.x.
- Pan W, Teyker R, Jackson W, Moll RH. Nutrient elements: diurnal variation in nitrate, potassium, and phosphate uptake in maize seedlings: considerations in screening genotypes for uptake efficiency. Journal of Plant Nutrition. 1987;10(9):1819–1833. doi:10.1080/01904168709363723.
- Maruyama A, Kuwagata T. Diurnal and seasonal variation in bulk stomatal conductance of the rice canopy and its dependence on developmental stage. Agricultural and Forest Meteorology. 2008;148(6–7):1161–1173. doi:10.1016/j.agrformet.2008.03.001.
- Soni P, Kumar G, Soda N, Singla-Pareek SL, Pareek A. Salt overly sensitive pathway members are influenced by diurnal rhythm in rice. Plant Signaling & Behavior. 2013;8(7):e24738. doi:10.4161/psb.24738.
- Waters I, Armstrong W, Thompson CJ, Setter TL, Adkins S, Gibbs J, Greenway H. Diurnal changes in radial oxygen loss and ethanol metabolism in roots of submerged and non-submerged rice seedlings. New Phytol. 1989;113(4):439–451. doi:10.1111/j.1469-8137.1989.tb00355.x.
- Reppert SM, Weaver DR. Coordination of circadian timing in mammals. Nature. 2002;418(6901):935–941. doi:10.1038/nature00965.
- Ono D, Honma K, Yanagawa Y, Yamanaka A, Honma S. GABA in the suprachiasmatic nucleus refines circadian output rhythms in mice.Communications Biology. 2019;2(1):232. doi:10.1038/s42003-019-0483-6.
- Fait A, Fromm H, Walter D, Galili G, Fernie AR. Highway or byway: the metabolic role of the GABA shunt in plants. Trends in Plant Science. 2008;13(1):14–19. doi:10.1016/j.tplants.2007.10.005.
- Shelp BJ, Mullen RT, Waller JC. Compartmentation of GABA metabolism raises intriguing questions. Trends in Plant Science. 2012;17(2):57–59. doi:10.1016/j.tplants.2011.12.006.
- Gaba FH. Signaling in Plants – Targeting the Missing Pieces of the Puzzle. JXB. 2020;71:6238–6245.
- Kinnersley AM, Turano FJ. Gamma aminobutyric acid (GABA) and plant responses to stress. Crit Rev Plant Sci. 2000;19(6):479–509. doi:10.1080/07352680091139277.
- Akçay N, Bor M, Karabudak T, Özdemir F, Türkan İ. Contribution of Gamma amino butyric acid (GABA) to salt stress responses of Nicotiana sylvestris CMSII mutant and wild type plants. J Plant Physiol. 2012;169(5):452–458. doi:10.1016/j.jplph.2011.11.006.
- Dimlioglu G, Das ZA, Bor M, Özdemir F, Türkan İ. The impact of GABA in harpin-elicited biotic stress responses in Nicotiana tabaccum. Journal of Plant Physiology. 2015;188:51–57. doi:10.1016/j.jplph.2015.08.005.
- Akcan-Das Z, Dimlioglu G, Bor M, Özdemir F. Zinc induced activation of GABA-shunt in tobacco (Nicotiana tabaccum L.). Environmental and Experimental Botany. 2016;122:78–84. doi:10.1016/j.envexpbot.2015.09.006.
- Ramesh S, Tyerman SD, Gilliham M, Xu B. γ-Aminobutyric acid (GABA) signalling in plants. Cell Mol Life Sci. 2017;74:1577–1603.
- Bown AW, Shelp BJ. Plant GABA: not Just a Metabolite. Trends Plant Sci. 2016;21(10):811–813. doi:10.1016/j.tplants.2016.08.001.
- Ramesh SA, Tyerman SD, Xu B, Bose J, Kaur S, Conn V, Domingos P, Ullah S, Wege S, Shabala S, et al. GABA signalling modulates plant growth by directly regulating the activity of plant-specific anion transporters. Nature Communications. 2015;6(1):7879. doi:10.1038/ncomms8879.
- Ramesh SA, Kamran M, Sullivan W, Chirkova L, Okamoto M, Degryse F, McLaughlin M, Gilliham M, Tyerman SD. Aluminum-activated malate transporters can facilitate GABA transport. Plant Cell. 2018;30(5):1147–1164. doi:10.1105/tpc.17.00864.
- Long Y, Tyerman SD, Gilliham GM. Cytosolic GABA inhibits anion transport by wheat ALMT1. New Phytologist. 2020;225(2):671–678. doi:10.1111/nph.16238.
- Mekonnen DW, Flügge U-I, Ludewig F. Gamma-aminobutyric acid depletion affects stomata closure and drought tolerance of Arabidopsis thaliana. Plant Science. 2016;245:25–34. doi:10.1016/j.plantsci.2016.01.005.
- Li L, Dou N, Zhang H, Wu C. The versatile GABA in plants. Plant Signaling & Behavior. 2021;16(3):3, 1862565. doi:10.1080/15592324.2020.1862565.
- Zarsky V. GABA receptor found in plants. Nat Plants. 2015;15155:1–2.
- Palanivelu R, Brass L, Edlund F, Preuss D. Pollen tube growth and guidance is regulated by POP2, an Arabidopsis gene that controls GABA levels. Cell. 2003;114(1):47–59. doi:10.1016/S0092-8674(03)00479-3.
- Dubois M, Claeys H, Van Den Broeck L, Inzé D. Time of day determines Arabidopsis transcriptome and growth dynamics under mild drought. Plant, Cell & Environment. 2017;40(2):180–189. doi:10.1111/pce.12809.
- Correa-Tedesco G, Rousseaux MC, Searles PS. Plant growth and yield responses in olive (Olea europaea) to different irrigation levels in an arid region of Argentina. Agricultural Water Management. 2010;97(11):1829–1837. doi:10.1016/j.agwat.2010.06.020.
- Signorelli S, Dans PD, Coitiño EL, Borsani O, Monza J. Connecting proline and γ-aminobutyric acid in stressed plants through non-enzymatic reactions. Plos One. 2015;10(3):e0115349. doi:10.1371/journal.pone.0115349.
- Bown AW, MacGregor KB, Shelp BJ. Gamma-aminobutyrate: defense against invertebrate pests? Trends in Plant Science. 2006;11(9):424–427. doi:10.1016/j.tplants.2006.07.002.
- Bor M, Turkan I. Is there a room for GABA in ROS and RNS signalling? Environmental and Experimental Botany. 2019;161:67–73. doi:10.1016/j.envexpbot.2019.02.015.
- Masclaux-Daubresse C, Valadier M-H, Carrayol E, Reisdorf-Cren M, Hirel B. Diurnal changes in the expressionof glutamate dehydrogenase and nitrate reductase are involved in the C/N balance of tobacco source leaves. Plant, Cell & Environment. 2002;25(11):1451–1462. doi:10.1046/j.1365-3040.2002.00925.x.
- Forde BG, Lea PJ. Glutamate in plants: metabolism, regulation, and signalling. JXB. 2007;58:2339–2358.
- Scott-Taggart CP, Van Cauwenberghe OR, McLean MD, Shelp BJ. Regulation of Γ -aminobutyric acid synthesis in situ by glutamate availability. Physiol Plant. 1999;106(4):363–369. doi:10.1034/j.1399-3054.1999.106402.x.
- Szabados L, Savouré A. Proline: a multifunctional amino acid. Trends in Plant Science. 2010;15(2):89–97. doi:10.1016/j.tplants.2009.11.009.
- Samach A, nouchi H, Gold SE, Ditta GS, Schwarz-Sommer Z, Yanofsky MF, Coupland G. Distinct roles of CONSTANS target genes in reproductive development of Arabidopsis. Science. 2000;288(5471):1613–1616. doi:10.1126/science.288.5471.1613.
- Türkan İ, Bor M, Özdemir F, Koca H. Differential responses of lipid peroxidation and antioxidants in the leaves of drought-tolerant P. acutifolius Gray and drought-sensitive P. vulgaris L. subjected to polyethylene glycol mediated water stress. Plant Sci. 2005;168(1):223–231. doi:10.1016/j.plantsci.2004.07.032.
- Choudhary NL, Sairam RK, Tyagi A. Expression of delta1-pyrroline-5-carboxylate synthetase gene during drought in rice (Oryza sativa L.). Indian J Biochem Biophys. 2005;42:366–370.
- Chaves MM, Flexas J, Pinheiro C. Photosynthesis under drought and salt stress: regulation mechanisms from whole plant to cell. Annals of Botany. 2009;103(4):551–560. doi:10.1093/aob/mcn125.
- Signorelli S, Arellano JB, Melø TB, Borsani O, Monza J. Proline does not quench singlet oxygen: evidence to reconsider its protective role in plants. Plant Physiology and Biochemistry. 2013;64:80–83. doi:10.1016/j.plaphy.2012.12.017.
- Smart RE, Bingham GE. Rapid estimates of relative water content. Plant Physiology. 1974;53(2):258–260. doi:10.1104/pp.53.2.258.
- Campos PS, Pham Thi AT. Effects of an abscisic acid pretreatment on membrane leakage and lipid composition of Vigna unguiculata leaf discs subjected to osmotic stress. Plant Science. 1997;130(1):11–18. doi:10.1016/S0168-9452(97)00199-4.
- Bates LS, Waldren RP, Teare ID. Rapid determination of free proline for water-stress studies. Plant and Soil. 1973;39(1):205–207. doi:10.1007/BF00018060.
- Bradford MM. A rapid and sensitive method for the quantitation of microgram quantities of protein utilizing the principle of protein-dye binding. Analytical Biochemistry. 1976;72(1–2):248–254. doi:10.1016/0003-2697(76)90527-3.
- Akihiro T, Koike S, Tani R, Tominaga T, Watanabe S, Iijima Y, Aoki K, Shibata D, Ashihara H, Matsukura C, et al. Biochemical mechanism on GABA accumulation during fruit development in tomato. Plant Cell Physiol. 2008;49(9):1378–1389. doi:10.1093/pcp/pcn113.
- Bartyzel I, Pelczar K, Paszkowski A. Functioning of the γ-aminobutyrate pathway in wheat seedlings affected by osmotic stress. Biologia Plantarum. 2003;46(2):221–225. doi:10.1023/B:BIOP.0000022255.01125.99.
- Baum G, Lev-Yadun S, Fridmann Y, Arazi T, Katsnelson H, Zik M, Fromm H. Calmodulin binding to glutamate decarboxylase is required for regulation of glutamate and GABA metabolism and normal development in plants.. Embo J. 1996;15(12):2988–2996. doi:10.1002/j.1460-2075.1996.tb00662.x.
- Bor M, Seckin B, Ozgur R, Yılmaz O, Ozdemir F, Turkan I. Comparative effects of drought, salt, heavy metal and heat stresses on gamma-aminobutryric acid levels of sesame (Sesamum indicum L.). Acta Physiol Plant. 2009;31(3):655–659. doi:10.1007/s11738-008-0255-2.