ABSTRACT
Bacterial leaf blight caused by Xanthomonas oryzae pv. oryzae (Xoo) has always been considered to be one of the most severe worldwide diseases in rice. Xoo strains usually use the highly conserved type III secretion system (T3SS) to deliver virulence effectors into rice cells and further suppress the host’s immunity. Previous studies reported that different Xanthomonas outer protein (Xop) effectors include XopZ from one strain appear to share functional redundancies on suppressing rice PAMP-triggered immunity (PTI). But only xopZ, except other xop genes, could significantly impaire Xoo virulence when individually deleting in PXO99 strains. Thus, the XopZ effector should not only suppress rice PTI pathway, but also has other unknown indispensable pathological functions in PXO99–rice interactions. Here, we also found that ∆xopZ mutant strains displayed lower virulence on Nipponbare leaves compared with PXO99 strains. We identified an oxysterol-binding related protein, ORP1C, as a XopZ-interacting protein in rice. Further studies found that rice ORP1C preliminarily played a positive role in regulating the resistance to PXO99 strains, and XopZ–ORP1C interactions cooperated to regulate the compatible interactions of PXO99-Nipponbare rice. The reactive oxygen species (ROS) burst and PTI marker gene expression data indicated that ORP1C were not directly relevant to the PTI pathway in rice. The deeper mechanisms underlying XopZ–ORP1C interaction and how XopZ and ORP1C cooperate for regulating the PXO99–rice interactions require further exploration.
To defend against invasion by numerous potential pathogenic microbes, plants have gradually evolved a complex, multilayered immune system. Plant immune systems usually include two defensive layers.Citation1 In the first layer, plants recognize the pathogen-associated molecular patterns (PAMPs) of invasive pathogens through pattern recognition receptors (PRRs) that mediate basal PAMP-triggered immunity (PTI). PTI responses usually include reactive oxygen species (ROS) burst, mitogen-activated protein (MAP) kinases activation, callose deposition, and PTI marker genes expression.Citation2,Citation3 In the second layer, plants have achieved the ability to recognize attacks of numerous virulence effectors through resistance (R) proteins that activated effector-triggered immunity (ETI).Citation1,Citation4 ETI is usually associated with the hypersensitive response (HR), a rapid and restricted cell death at infection sites.Citation5 The co-evolution between the virulence effectors of pathogens and R proteins of plants together led to the so-called “zigzag model”.Citation1 Traditionally, the PTI and ETI pathways are separated, but recent studies have suggested that ETI signaling depends on PTI, PTI and ETI cooperate to regulate plant immunity.Citation6,Citation7
To infect a host, pathogenic bacteria usually translocate effectors into the host cell through a type III secretion system (T3SS) and further suppress the plant innate immunity. T3SS effectors usually act kinds of biochemical roles, including protein kinases, phosphatases, proteases, acetyltransferases, and E3-ubiquitin ligases, among others.Citation8,Citation9 Mechanistically, T3SS effectors have been found to interfere with various different host defense pathways, usually including MAPK signaling, phytohormone signaling, cytoskeleton formation, proteasome- or autophagy-dependent protein degradation, and host gene expression, among others.Citation8,Citation9 To understand the biological functions of T3SS effectors in host cells, it is key to identify plant targets and further explore the mechanism of effector-triggered modifications in different plant cellular processes.
Bacterial leaf blight caused by Xanthomonas oryzae pv. oryzae (Xoo) has always been considered to be one of the most severe worldwide diseases in rice.Citation10 Similar to many other gram-negative pathogenic bacteria, Xoo strains also use the highly conserved T3SS to deliver numerous T3SS effectors into rice cells.Citation11 However, unlike with other pathogenic bacteria, Xanthomonas T3SS effectors are categorized into two groups: transcription activator-like (TAL) effectors and Xanthomonas outer protein (Xop) effectors, which are also called non-TAL effectors.Citation12,Citation13 Most TAL effectors have been identified as eukaryotic transcription activators and further transcriptionally activate the corresponding target genes for host disease resistance or susceptibility.Citation14,Citation15 For example, PXO99 ∆pthXo1 mutant strains have completely lost their virulence toward Nipponbare and other susceptible rice cultivars. Further studies found that the TAL effector PthXo1 could specifically bind the promoter region of the OsSWEET11 gene and up-regulate its expression in rice cells. The SWEET protein coded by the OsSWEET11 gene belongs to the membrane sugar transporter family, which provides nutrients for pathogenic bacteria growth through transporting the intracellular sugar to the apoplasts.Citation16 Tian et al. identified an Xoo resistance gene Xa10 which stimulated by the TAL effector AvrXa10 in IRBB10 rice. Mechanistically, Xa10 is located on the endoplasmic reticulum membrane and induces programmed cell death (PCD) by destroying the intracellular Ca2+ balance, and further limits the expansion of Xoo lesions.Citation17
To date, 22, 20, and 16 Xop effectors have been identified in different Xoo strains PXO99, MAFF311018 and KACC10311, respectively. The sequence of Xop effectors in different Xoo strains is highly conservative (http://www.xanthomonas.org/t3e.html). Unlike the definite roles that TAL effectors play in Xoo-rice interactions, the pathogenic functions of numerous Xop effectors remain unclear or redundant.Citation18 Recently, we reported that the XopI effector of the African Xoo BAI3 strain could strongly suppress SAR immunity through impersonating and hijacking the host ubiquitin proteasome system (UPS) in rice.Citation19 Additionally, other studies found that several Xops have recently been identified to target and suppress the host PTI. XopR from the Xoo strains MAFF311018 or PXO99 has been shown to target the receptor-like kinase BIK1 and further block rice PTI.Citation20,Citation21 XopAA, XopP, and XopY from the Xoo MAFF311018 strain suppress rice PTI through interacting with the host PRR receptor OsBAK1, E3 ubiquitin-ligase OsPUB44, and receptor-like kinase OsRLCK185, respectively.Citation22–24 In addition, the XopN, XopQ, XopV, XopX, and XopZ effectors have all been proven to suppress rice PTI pathway, suggesting that numerous Xops effectors from one strain appear to share functional redundancies in suppressing rice PTI, but their specific targets on host PTI pathway are diverse and unknown.Citation18,Citation25–27 Interestingly, Song and Yang found that only xopZ, except other xop genes, could significantly impair Xoo virulence on the leaves of IR24 rice when deleted in PXO99 strain.Citation18 In summary, we presume that other Xop effectors could also complete the suppression for host PTI in PXO99∆xopZ-rice interactions, XopZ effector should not only suppress host PTI, but also has other unknown indispensable pathological functions in PXO99-rice interactions.
Two equivalent xopZ effector genes (PXO_01041 and PXO_06125) were identified in PXO99 genome due to a 212-kb sequence duplication.Citation18,Citation28 In a previous study, Song and Yang constructed the PXO99 mutants ∆xopZ strain by using traditional homologous recombination methods that introduced kanamycin resistance cassettes at two xopZ sites in PXO99 genome.Citation18 However, in this study, we constructed the PXO99 mutants ∆xopZ (both PXO_01041 and PXO_06125 deletions) by using a marker-free deletion method. We then evaluated the virulence of xopZ-related strains on Nipponbare rice leaves. The inoculation data showed that the xopZ deletion significantly impaired the Xoo virulence, the average lesion lengths on Nipponbare leaves inoculated with ∆xopZ and ∆xopZ/pHMI strains were significantly shorter than those on rice leaves inoculated with PXO99 and ∆xopZ/xopZ strains (), and the populations of ∆xopZ and ∆xopZ/pHMI strains in Nipponbare leaves were substantially lower than PXO99 and ∆xopZ/xopZ strains (). In summary, consistent with the important role of the XopZ effector played in the PXO99-IR24 rice,Citation18 our experiments also indicated that XopZ is indispensable for compatible interaction of PXO99-Nipponbare rice (). However, when the same mutant strain PXO99∆xopZ was inoculated on Kitaake rice leaves, no obvious virulence reduction was observed.Citation27 This might be due to the genetic background of the host rice or the specialized interaction of host-pathogen race.
Figure 1. XopZ effector contributes to virulence of PXO99 strain on Nipponbare. (a) Pathogenic phenotypes of xopZ-related strains on Nipponbare rice leaves photographed at 14 days post-inoculation (dpi). (b) Length measurement of the blight lesions at 14 dpi. Each bar represents the mean and standard deviation (SD) of 10 rice leaves. (c) Statistics of Xoo populations in Nipponbare leaves at 14 dpi. Each bar represents the mean and SD of 5 rice leaves. In (b) and (c), different letters indicate significant differences based on Duncan’s multiple range analysis (P < 0.05). These experiments were repeated three times and similar results were obtained.
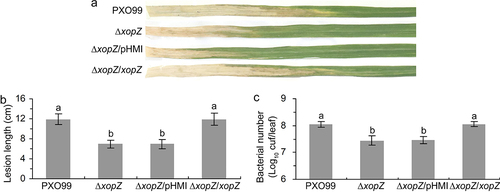
To date, the specific host targets of XopZ have not been characterized. We further screened for rice proteins that interact with XopZ by yeast two-hybrid (Y2H) assay. First, yeast strains transformed with bait plasmids and Nipponbare cDNA libraries were mixed for conjugation growth on synthetic dextrose (SD) medium, and ORP1C (accession number: LOC_Os03g49770) was identified as a candidate target to interact with XopZ according to sequencing of numerous positive clones. ORP1C belongs to the oxysterol-binding related protein family (ORPs).Citation29 The interaction between XopZ and the full-length ORP1C protein was further confirmed according to the phenotype of yeast growth phenotype and X-gal visualization of the paired Y2H assay (). Next, we explored whether XopZ could bind to ORP1C in rice cells. The XopZ-HA plasmid was transiently expressed together with ORP1C-Flag in Nipponbare rice protoplasts, and subsequent co-immunoprecipitation (Co-IP) tests revealed that XopZ could specifically bind to ORP1C in rice cells ().
Figure 2. XopZ specifically bind to ORP1C in yeast and rice cells. (a) Y2H assay identifying the interaction between XopZ and ORP1C in yeast cells. The transformant expressing BD-53 and AD-T was used as a positive control. The transformants expressing BD-Lam and AD-T, BD-XopZ and empty AD, empty BD and AD-ORP1C were used as negative controls. (b) Co-IP assays identifying the interaction between XopZ and ORP1C in rice protoplasts. Whole-cell lysates (WCL) of rice protoplasts transformed with different plasmid combinations were prepared and analyzed by immunoblotting with marked antibodies, Co-IP was conducted with the Flag antibody (IP Flag) and the interaction was further analyzed by immunoblotting with Flag or HA antibodies.
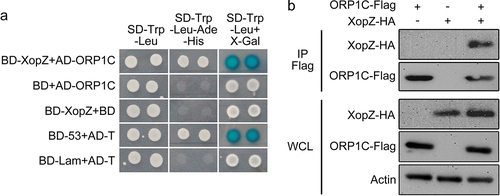
To explore the biological functions of ORP1C in rice cells, we cultivated ORP1C gene mutant rice with the CRISPR-Cas9 gene editing system. First, we calculated and constructed the specific sgRNA to target the nucleotides of the ORP1C gene from 283 to 305 (). The plasmids containing the specific sgRNA and Cas9 expression cassette were introduced into Nipponbare rice calluses. In total, two homozygous mutant rice lines (ORP1C#2 and ORP1C#9) were identified according to the sequencing results in transgenic progenies. ORP1C#2 had a homozygous allelic mutation with 2-bp deletion, ORP1C#9 had a 1-bp insertion in the target gene sites (). These two deletions or insertions both caused frameshift mutations and early stop codons.
Figure 3. Identification of ORP1C mutant rice generated by the CRISPR-Cas9 gene editing system. (a) Calculation of the sgRNA target sites based on ORP1C sequence analysis. (b) Sequencing identification of ORP1C mutant rice lines. (c) Growth phenotypes of 100-day-old Nipponbare WT and ORP1C mutant rice. (d) Days to heading of different rice lines. (e) Seed setting rates of different rice lines. The seed setting rate was calculated as the ratio of fully filled seeds to the total number of grains. In (d) and (e), each bar represents the mean and SD of 5 rice plants. Different letters indicate significant differences based on Duncan’s multiple range analysis (P < 0.05). These experiments were repeated three times with similar results obtained.
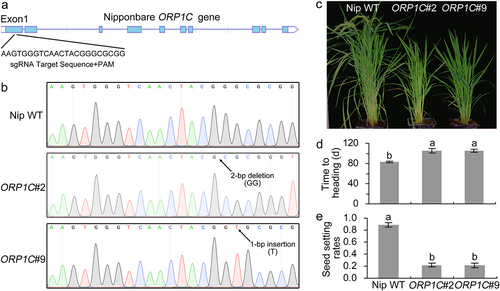
Most eukaryotic genomes encode oxysterol-binding proteins (OSBPs) and OSBP-related proteins (ORPs). These OSBPs/ORPs have been widely studied in humans and fungi,Citation30,Citation31 and are indispensable for diverse cellular functions, including signaling cascades, endosomal movements, membrane trafficking, tumor metastasis, cell cycle progression, and so on.Citation31 Mechanistically, OSBPs/ORPs act as a classical non-vesicular lipid transporter that sense and transfer kinds of lipids, especially kinds of sterols and phospholipids, and further regulate the lipid distribution at the different cell membranes.Citation31 However, the unique structural basis of numerous OSBPs/ORPs for sensing and transferring different kinds of specific lipids are not fully understood.Citation32,Citation33 Currently, 12 and 6 OSBP/ORP genes have been identified in the Arabidopsis and rice genomes, respectively.Citation29 The TAIR database demonstrates that different OSBP/ORP genes are expressed at different levels under numerous stress conditions in Arabidopsis.Citation29 However, the definite physiological and developmental functions of these OSBPs/ORPs in plants remain unknown. In particular, whether or how OSBPs/ORPs are involved in regulating lipids trafficking remains to be determined in plants.
During this study, we observed some significant changes in the morphological and agronomic phenotypes in the ORP1C mutant rice as compared to the Nipponbare WT rice under identical natural conditions. The ORP1C mutant rice exhibited a slight dwarf and late-heading phenotype in the adult stages (). In addition, ORP1C mutant rice also exhibited a mild sterile phenotype in the harvest period, and the seed setting rates of the ORP1C mutant rice were all highly lower compared with Nipponbare WT rice (). These data indicate that the ORP1C may play an important role in regulating the growth and yield of rice.
We further explored the biological functions of the ORP1C gene in PXO99–rice interactions. The statistical data show that the average lesion lengths on the leaves of the ORP1C#2 and #9 mutants were apparently longer than those on the leaves of WT rice (). Above results preliminarily indicate that ORP1C positively regulates the resistance to PXO99 strains in rice. In addition, according to the statistical data of lesion length, we found that ∆xopZ strains displayed impaired virulence on Nipponbare WT leaves compared with PXO99 or ∆xopZ/xopZ strains, but these three strains (PXO99, ∆xopZ and ∆xopZ/xopZ) displayed a nearly equivalent severe virulence on ORP1C mutant rice (ORP1C#2 and ORP1C#9) (). These results preliminarily suggest that the virulence function of XopZ is dependent on ORP1C, ORP1C should be the specific host targets of the XopZ effector and further cooperate to regulate the compatible interaction of PXO99-Nipponbare rice.
Figure 4. XopZ and ORP1C cooperate to regulate the virulence of PXO99 strain on Nipponbare not through the host PTI pathway. (a) Pathogenic phenotypes of xopZ-related strains on different rice leaves photographed at 14 dpi. (b) Length measurements of blight lesion at 14 dpi. Each bar represents the mean and SD of 10 rice leaves. These experiments were repeated three times with similar results obtained. (c) Measurement of ROS bursts in rice leaves after treatment with different elicitors. (d) Expression analyses of PAL1 and PBZ1 gene in different rice leaves after treatment with different elicitors. The average expression levels of PAL1 and PBZ1 genes in Nipponbare WT leaves treated with control water solution are defined as “1”. In (c) and (d), each bar represents the mean and SD of three biological replicates, five independent experiments were repeated with similar results obtained. In (b) to (d), different letters indicate significant differences based on Duncan’s multiple range analysis (P < 0.05).
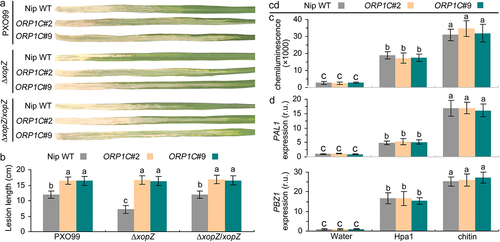
For the benefit of survival and proliferation, recent advances have revealed that numerous intracellular and cell-associated animal pathogenic bacteria have evolved a series of efficient strategies to gain advantages by hijacking host lipids metabolism.Citation34–38 However, to date, relevant studies on the hijacking of lipid metabolism by plant pathogenic bacteria remain scarce.Citation39,Citation40 In this study, we have found that the XopZ effector of PXO99 could specifically bind to the rice OSBP-related protein ORP1C (), and interfere with the resistance function of ORP1C in PXO99–rice interactions (). However, the mechanisms underlying how ORP1C positively regulates the resistance of rice to PXO99 remain unknown.
In previous studies, XopZ was proved to suppress the host basal PTI defense in Nicotiana benthamiana leaves or rice protoplasts,Citation18,Citation27 however, this PTI suppression effect of XopZ have not been proven to have a direct relationship with the virulent role of XopZ played in compatible interaction of PXO99-rice.Citation18 Hpa1 proteins from Xoo strains have been recognized as PAMPs and further activate the PTI response on different plants, similar to other PAMPs.Citation41 Herein, we found that the Hpa1 protein and fungal classical PAMP chitin could both activate the rice PTI response by inducing a ROS burst () and up-regulating the expression of PTI marker PAL1 and PBZ1 genes in different rice leaves (Nipponbare WT, ORP1C#2, or #9 mutants leaves), equivalently (). These data preliminarily indicate that ORP1C plays no role in regulating the rice PTI pathway, and XopZ–ORP1C interaction cooperates to regulate the compatible interaction of PXO99-Nipponbare rice not through the rice PTI pathway. In summary, the deeper mechanisms underlying XopZ–ORP1C interactions and how XopZ and ORP1C cooperate for regulating the PXO99–rice interaction require further exploration.
Materials and methods
Plant materials
Nipponbare WT or ORP1C mutant rice were used, and were grown in growth chambers, greenhouses, or fields as described previously.Citation19 For seed breeding and determination of the heading time, rice plants were grown in Xuzhou City of Jiangsu Province in China under natural filed conditions from June to October. The ORP1C mutant rice lines were generated with the CRISPR/Cas9 gene editing system.Citation19 Briefly, the oligonucleotides targeting the ORP1C gene were calculated on Cas-Designer website (http://www.rgenome.net/cas-designer/). The synthesized ORP1C oligonucleotides were linked into the plant CRISPR/Cas9 plasmids pRGEB32, and this pRGEB32-ORP1C plasmid was further introduced into Nipponbare calli by an Agrobacterium-mediated transformation assay as previous methods.Citation42 For mutant identification, the target and flanking ORP1C gene fragments amplified from rice genomic DNA were further sequenced using an corresponding primer.
Microbial strains
Different microbial strains including Escherichia coli, Agrobacterium tumefacien, Yeast and Xoo strains were used and cultured as described previously.Citation19 Two equivalent xopZ effector genes (PXO_01041 and PXO_06125) were found in the PXO99 genome due to a 212-kb sequence duplication,Citation18,Citation28 the xopZ genes (both PXO_01041 and PXO_06152) were both deleted following an marker-free deletion method with two cycles by the pK18mobsacB plasmid as described previously,Citation19 and the ∆xopZ (both PXO_01041 and PXO_06125 deletions) mutants were screened with sucrose-positive and kanamycin-negative phenotypes on nutrient agar (NA) plates, and eventually identified by the PCR assay. The ∆xopZ/xopZ complementation and ∆xopZ/pHM1 control strains were also constructed with the assistance of the pHM1 plasmid as described previously.Citation19
Xoo virulence assay
XopZ-related strains were cultured in nutrient broth (NB) medium overnight. Inoculated strains were resuspended in dH2O at OD600 = 0.6, and inoculated on 45-day-old rice by the leaf clipping assay.Citation19 The pathogenic phenotypes of different Xoo strains were identified by photographing or surveying the leaf blight lesion at 14 dpi. The populations of different xopZ-related strains in different rice leaves were calculated as described previously.Citation19
Protein interaction assays
The Y2H experiments were performed following previous studies.Citation19 The xopZ gene was linked into the bait plasmids pGBKT7. The yeasts transformed with bait plasmids were then co-cultured with yeasts-transformed cDNA libraries of Nipponbare rice leaves (GeneCreate Biotech). Conjugated yeasts were screened on SD-WL (without Trp and Leu) and SD-WLAH (without Trp, Leu, Ade, and His) plates. Rice library plasmids from positive yeast cells of SD-WLAH were introduced into E. coli and further sequenced. The full-length XopZ-ORP1C protein interactions were identified by yeast growth on SD-WLAH plates that were co-transformed with pGBKT7-xopZ and pGADT7-ORP1C. X-Gal agarose mixtures for detecting the lacZ activity were overlaid on yeast SD-WL plates to further confirm their interactions.
For the Co-IP assay, the plant transient expression plasmids pUC35s-XopZ-HA and pUC35s-ORP1C-Flag were transiently expressed in Nipponbare rice protoplasts alone or together. Whole-cell lysates (WCL) of rice protoplasts were prepared and analyzed by immunoblotting with Flag, HA or Actin antibodies (Beyotime) at 12 h after transient expression. Meanwhile, WCL was incubated with Flag antibody for 16 h at 4°C. The mixture was then incubated with Dynabeads protein A (Beyotime) for 5 h at 4°C. Immunoprecipitated proteins were separated in 8% SDS-PAGE gels and immunoblotted by Flag or HA antibodies.Citation19
ROS burst measurement
The ROS bursts were measured with the luminal-dependent chemiluminescence assay.Citation43 Leaf disks from 21-day-old rice were collected and incubated in dH2O for 12 h. Five leaf disks per sample were placed in a microcentrifuge tube containing 100 uL of luminol (Bio-Rad), 1 uL of horseradish peroxidase (Beyotime), and elicitors (50 nM chitin, 5 μg/mL Hpa1 protein solutions or water as control) to test the ROS bursts. The luminescence was measured using Varioskan LUX (Thermo Scientific) at 1 h after treatment.
Gene expression analysis
To analyze the expression levels of PAL1 and PBZ1 genes, rice leaves treated with the Hpa1 protein or chitin solutions were collected and ground together at 9 h. Rice RNA was extracted with an RNAprep pure Plant Kit (Tiangen), and reverse transcribed into cDNA with HiScript II Reverse Transcriptase (Vazyme). qRT-PCR was conducted using the ChamQ SYBR qPCR Master Mix (Vazyme) on an StepOnePlus Real-Time PCR system (Applied Biosystems). The constitutively expressed Ubi1 (rice) genes were selected as references.
Disclosure statement
No potential conflict of interest was reported by the author(s).
Correction Statement
This article has been republished with minor changes. These changes do not impact the academic content of the article.
Additional information
Funding
References
- Jones JD, Dangl JL. The plant immune system. Nature. 2006;444(7117):323–7. PMID:17108957. doi:10.1038/nature05286
- Boller T, Felix G. A renaissance of elicitors: perception of microbe-associated molecular patterns and danger signals by pattern-recognition receptors. Annu Rev Plant Biol. 2009;60:379–406. PMID:19400727. doi:10.1146/annurev.arplant.57.032905.105346.
- Zipfel C. Early molecular events in PAMP-triggered immunity. Curr Opin Plant Biol. 2009;12:414–420. PMID:19608450. doi:10.1016/j.pbi.2009.06.003.
- Martin GB, Bogdanove AJ, Sessa G. Understanding the functions of plant disease resistance proteins. Annu Rev Plant Biol. 2003;54:23–61. PMID:14502984. doi:10.1146/annurev.arplant.54.031902.135035.
- Mur LA, Kenton P, Lloyd AJ, Ougham H, Prats E. The hypersensitive response; the centenary is upon us but how much do we know? J Exp Bot. 2008;59:501–520. PMID:18079135. doi:10.1093/jxb/erm239.
- Yuan M, Jiang Z, Bi G, Nomura K, Liu M, Wang Y, Cai B, Zhou JM, He SY, Xin XF. Pattern-recognition receptors are required for NLR-mediated plant immunity. Nature. 2021;592:105–109. PMID:33692546. doi:10.1038/s41586-021-03316-6.
- Ngou BPM, Ahn HK, Ding P, Jones JDG. Mutual potentiation of plant immunity by cell-surface and intracellular receptors. Nature. 2021;592:110–115. PMID:33692545. doi:10.1038/s41586-021-03315-7.
- Büttner D. Behind the lines-actions of bacterial type III effector proteins in plant cells. FEMS Microbiol Rev. 2016;40:894–937. PMID:28201715. doi:10.1093/femsre/fuw026.
- Khan M, Seto D, Subramaniam R, Desveaux D. Oh, the places they’ll go! A survey of phytopathogen effectors and their host targets. Plant J. 2018;93:651–663. PMID:29160935. doi:10.1111/tpj.13780.
- Niño-Liu DO, Ronald PC, Bogdanove AJ. Xanthomonas oryzae pathovars: model pathogens of a model crop. Mol Plant Pathol. 2006;7(5):303–324. PMID:20507449. doi:10.1111/j.1364-3703.2006.00344.x
- Ji H, Dong H. Key steps in type III secretion system (T3SS) towards translocon assembly with potential sensor at plant plasma membrane. Mol Plant Pathol. 2015;16(7):762–773. PMID:25469869. doi:10.1111/mpp.12223
- Furutani A, Takaoka M, Sanada H, Noguchi Y, Oku T, Tsuno K, Ochiai H, and Tsuge S. Identification of novel type III secretion effectors in Xanthomonas oryzae pv.oryzae. Mol Plant Microbe Interact. 2009;22:96–106. PMID:19061406. doi:10.1094/MPMI-22-1-0096.
- White FF, Potnis N, Jones JB, Koebnik R. The type III effectors of Xanthomonas. Mol Plant Pathol. 2009;10:749–766. PMID:19849782. doi:10.1111/j.1364-3703.2009.00590.x.
- Kay S, Hahn S, Marois E, Hause G, Bonas U. A bacterial effector acts as a plant transcription factor and induces a cell size regulator. Science. 2007;318:648–651. PMID:17962565. doi:10.1126/science.1144956.
- White FF, Yang B. Host and pathogen factors controlling the rice-Xanthomonas oryzae interaction. Plant Physiol. 2009;150:1677–1686. PMID:19458115. doi:10.1104/pp.109.139360.
- Yang B, Sugio A, White FF. Os8N3 is a host disease-susceptibility gene for bacterial blight of rice. Proc Natl Acad Sci USA. 2006;103:10503–10508. PMID:16798873. doi:10.1073/pnas.0604088103.
- Tian D, Wang J, Zeng X, Gu K, Qiu C, Yang X, Zhou Z, Goh M, Luo Y, Murata-Hori M, et al. The rice TAL effector-dependent resistance protein XA10 triggers cell death and calcium depletion in the endoplasmic reticulum. Plant Cell. 2014;26:497–515. PMID:24488961. doi:10.1105/tpc.113.119255.
- Song C, and Yang B. Mutagenesis of 18 type III effectors reveals virulence function of XopZ(PXO99) in Xanthomonas oryzae pv.oryzae. Mol Plant Microbe Interact. 2010;23:893–902. PMID:20521952. doi:10.1094/MPMI-23-7-0893.
- Ji H, Liu D, Zhang Z, Sun J, Han B, Li Z. A bacterial F-box effector suppresses SAR immunity through mediating the proteasomal degradation of OsTrxh2 in rice. Plant J. 2020;104:1054–1072. PMID:32881160. doi:10.1111/tpj.14980.
- Akimoto-Tomiyama C, Furutani A, Tsuge S, Washington EJ, Nishizawa Y, Minami E, Ochiai H. XopR, a type III effector secreted by Xanthomonas oryzae pv. oryzae, suppresses microbe-associated molecular pattern-triggered immunity in Arabidopsis thaliana. Mol Plant Microbe Interact. 2012;25:505–514. PMID:22204644. doi:10.1094/MPMI-06-11-0167.
- Wang S, Sun J, Fan F, Tan Z, Zou Y, Lu D. A Xanthomonas oryzae pv. oryzae effector, XopR, associates with receptor-like cytoplasmic kinases and suppresses PAMP-triggered stomatal closure. Sci China Life Sci. 2016;59:897–905. PMID:27520828. doi:10.1007/s11427-016-5106-6.
- Yamaguchi K, Nakamura Y, Ishikawa K, Yoshimura Y, Tsuge S, Kawasaki T. Suppression of rice immunity by Xanthomonas oryzae type III effector Xoo2875. Biosci Biotechnol Biochem. 2013;77:796–801. PMID:23563550. doi:10.1271/bbb.120929.
- Yamaguchi K, Yamada K, Ishikawa K, Yoshimura S, Hayashi N, Uchihashi K, Ishihama N, Kishi-Kaboshi M, Takahashi A, Tsuge S, et al. A receptor-like cytoplasmic kinase targeted by a plant pathogen effector is directly phosphorylated by the chitin receptor and mediates rice immunity. Cell Host Microbe. 2013;13:347–357. PMID:23498959. doi:10.1016/j.chom.2013.02.007.
- Ishikawa K, Yamaguchi K, Sakamoto K, Yoshimura S, Inoue K, Tsuge S, Kojima C, Kawasaki T. Bacterial effector modulation of host E3 ligase activity suppresses PAMP-triggered immunity in rice. Nat Commun. 2014;5:5430. PMID:25388636. doi:10.1038/ncomms6430.
- Cheong H, Kim CY, Jeon JS, Lee BM, Sun Moon J, Hwang I. Xanthomonas oryzae pv. oryzae type III effector XopN targets OsVOZ2 and a putative thiamine synthase as a virulence factor in rice. PLoS One. 2013;8:e73346. PMID:24019919. doi:10.1371/journal.pone.0073346.
- Sinha D, Gupta MK, Patel HK, Ranjan A, and Sonti RV. Cell wall degrading enzyme induced rice innate immune responses are suppressed by the type 3 secretion system effectors XopN, XopQ, XopX and XopZ of Xanthomonas oryzae pv.oryzae. PLoS One. 2013;8:e75867. PMID:24086651. doi:10.1371/journal.pone.0075867.
- Long J, Song C, Yan F, Zhou J, Zhou H, Yang B. Non-TAL effectors from xanthomonas oryzae pv. oryzae suppress peptidoglycan-triggered MAPK activation in rice. Front Plant Sci. 2018;9:1857. PMID:30631333. doi:10.3389/fpls.2018.01857.
- Salzberg SL, Sommer DD, Schatz MC, Phillippy AM, Rabinowicz PD, Tsuge S, Furutani A, Ochiai H, Delcher AL, Kelley D, et al. Genome sequence and rapid evolution of the rice pathogen Xanthomonas oryzae pv. oryzae PXO99A. BMC Genomics. 2008;9:204. PMID:18452608. doi:10.1186/1471-2164-9-204.
- Umate P. Oxysterol binding proteins (OSBPs) and their encoding genes in Arabidopsis and rice. Steroids. 2011;76:524–529. PMID:21281657. doi:10.1016/j.steroids.2011.01.007.
- Ridgway ND. Oxysterol-binding proteins. Subcell Biochem. 2010;51:159–182. PMID:20213544. doi:10.1007/978-90-481-8622-8_6.
- Olkkonen VM, Li S. Oxysterol-binding proteins: sterol and phosphoinositide sensors coordinating transport, signaling and metabolism. Prog Lipid Res. 2013;52:529–538. PMID:23830809. doi:10.1016/j.plipres.2013.06.004.
- Raychaudhuri S, Prinz WA. The diverse functions of oxysterol-binding proteins. Annu Rev Cell Dev Biol. 2010;26:157–177. PMID:19575662. doi:10.1146/annurev.cellbio.042308.113334.
- Tong J, Manik MK, Yang H, Im YJ. Structural insights into nonvesicular lipid transport by the oxysterol binding protein homologue family. Biochim Biophys Acta. 2016;1861:928–939. PMID:26784528. doi:10.1016/j.bbalip.2016.01.008.
- Pizarro-Cerdá J, Kühbacher A, Cossart P. Phosphoinositides and host-pathogen interactions. Biochim Biophys Acta. 2015;1851:911–918. PMID:25241942. doi:10.1016/j.bbalip.2014.09.011.
- Toledo A, Benach JL. Hijacking and use of host lipids by intracellular pathogens. Microbiol Spectr. 2015;3 PMID:27337282. doi:10.1128/microbiolspec.VMBF-0001-2014.
- Lang R, Mattner J. The role of lipids in host microbe interactions. Front Biosci. 2017;22:1581–1598. PMID:28410133. doi:10.2741/4559.
- Walpole GFW, Grinstein S, Westman J. The role of lipids in host-pathogen interactions. IUBMB Life. 2018;70:384–392. PMID:29573124. doi:10.1002/iub.1737.
- Allen PE, Martinez JJ. Modulation of host lipid pathways by pathogenic intracellular bacteria. Pathogens. 2020;9:614. PMID:32731350. doi:10.3390/pathogens9080614.
- Shah J. Lipids, lipases, and lipid-modifying enzymes in plant disease resistance. Annu Rev Phytopathol. 2005;43:229–260. PMID:16078884. doi:10.1146/annurev.phyto.43.040204.135951.
- Siebers M, Brands M, Wewer V, Duan Y, Hölzl G, Dörmann P. Lipids in plant-microbe interactions. Biochim Biophys Acta. 2016;1861:1379–1395. PMID:26928590. doi:10.1016/j.bbalip.2016.02.021.
- Choi MS, Kim W, Lee C, Oh CS. Harpins, multifunctional proteins secreted by gram-negative plant-pathogenic bacteria. Mol Plant Microbe Interact. 2013;26:1115–1122. PMID:23745678. doi:10.1094/MPMI-02-13-0050-CR.
- Hiei Y, Ohta S, Komari T, Kumashiro T. Efficient transformation of rice (Oryza sativa L.) mediated by Agrobacterium and sequence analysis of the boundaries of the T-DNA. Plant J. 1994;6:271–282. PMID:7920717. doi:10.1046/j.1365-313x.1994.6020271.x.
- Park CH, Chen S, Shirsekar G, Zhou B, Khang CH, Songkumarn P, Afzal AJ, Ning Y, Wang R, Bellizzi M, et al. The Magnaporthe oryzae effector AvrPiz-t targets the RING E3 ubiquitin ligase APIP6 to suppress pathogen-associated molecular pattern-triggered immunity in rice. Plant Cell. 2012;24(11):4748–4762. PMID:23204406. doi:10.1105/tpc.112.105429.