ABSTRACT
Brassinosteroids (BRs) regulate of maize (Zea mays L.) growth, but the underlying molecular mechanism remains unclear. In this study, we used a multi-disciplinary approach to determine how BRs regulate maize morphology and physiology during development. Treatment with the BRs promoted primary root the elongation and growth during germination, and the early development of lateral roots. BRs treatment during the middle growth stage increased the levels of various stress resistance factors, and enhanced resistance to lodging, likely by protecting the plant against stem rot and sheath rot. BRs had no significant effect on plant height during late growth, but it increased leaf angle and photosynthetic efficiency, as well as yield and quality traits. Our findings increase our understanding of the regulatory effects of BR on maize root growth and development and the mechanism by which BR improves disease resistance, which could further the potential for using BR to improve maize yield.
KEYWORDS:
Introduction
Maize (Zea mays L.) is the third largest food crop in China. It is also an important source of human food, animal feed and industrial raw materials. The planting area and output of corn in China rank first in the world. Corn plays an important role in China’s agricultural production and national economy.Citation1,Citation2
Biotic and abiotic stresses affect maize yields worldwide and improving stress tolerance provides an important approach for improving crop yields. One hallmark of stress conditions is high levels of reactive oxygen species (ROS) such as superoxide and hydrogen peroxide (H2O2). These ROS typically cause membrane lipid peroxidation under stress conditionsCitation3,Citation4 such as high temperature, high salt, alkali, and strong light, and the content of malondialdehyde (MDA) reflects the degree of lipid peroxidation of plant cell membranes. High MDA content indicates a high degree of lipid peroxidation and suggests that the cell membrane is seriously damaged.Citation5–7
Plants express various enzymes that help them detoxify ROS. Superoxide dismutase (SOD)Citation8 effectively enhances the stress tolerance of plants by catalyzing the disproportionation of superoxide anion free radicals, thus limiting damage by ROS to the cell membrane system.Citation9 Peroxidase (POD) is an important protective enzyme for preventing oxygen free radical damage in plants, which is closely related to stress tolerance. H2O2 is an oxidative metabolite in cells. Higher contents of H2O2 cause greater damage to cell sand reduce the ability of plants to tolerate stress.Citation10–12 Ascorbate peroxidase (APX2) also scavenges hydrogen peroxide in plant cells.Citation13,Citation14
Plants also produce various small molecules to resist stress. For example, the content of proline in plants reflects the stress tolerance of plants to a certain extent. Stress conditions cause the accumulation of proline in plants, which is dependent on changes in the activity of 1-pyrroline-5-carboxylic acid synthase (P5CS)Citation15,Citation16 a key enzyme in proline biosynthesis. In addition, the higher the soluble sugar content of plants, the smaller the possibility of cell water loss, increasing the possibility of plant survival under stress and therefore improving stress tolerance.Citation17,Citation18
The plant root system functions in absorbing water and nutrients from the soil, storing photosynthetic products, fixing nitrogen (in legumes), and biosynthesizing plant hormones.Citation1,Citation19,Citation20 Roots also play a key role in preventing lodging, which occurs when roots detach from the soil or stems bend due to external forces such as high wind. Stem bending damages the stem transport system, affecting the upward transport of water and nutrients from roots and the transport of photosynthetic products from leaves to ears, thus hampering seed setting and resulting in severe yield losses. Lodging also destroys leaf tissue, greatly reducing photosynthetic efficiency and thus yields, and hampers mechanical harvesting of crops.Citation21 Lodging has become a major factor limiting maize yield.Citation22–24
Plants typically have tap or fibrous root systems. The roots of soybean, canola, and other dicotyledonous plants are tap root systems, while wheat, rice, maize, and other monocotyledon plants have fibrous root systems. Tap root systems consist of a principal root and branch roots; the strongly developed principal root is thick and long and has geotropism and deep rooting ability, while the branch roots generated from the principal root are relatively short and thin, growing around the principal root at an angle. By contrast, fibrous root systems develop a mass of whisker-shaped roots with a shallow distribution and a large range, along with adventitious roots growing from the stem base.Citation25
The development of branch roots involves the establishment of founder cells, development of lateral root primordia, and outgrowth of the branch root.Citation26,Citation27 Branch roots mainly originate in specific sheath cells of the maternal root that are stimulated to dedifferentiate and reestablish cell division to form lateral root primordia. Lateral roots grow from the lateral root primordia and the cells of the growing point continue to divide, enlarge, and differentiate. Root tip cells secrete enzymes to partially dissolve cortical and epidermal cells of the parent root, allowing the lateral roots to pass through the parent root epidermis into the external environment. This complex and orderly process is repeated during subsequent secondary and tertiary lateral root development.Citation28–30 Moreover, the growth and development of plant lateral roots is affected by internal factors, such as hormones, and external factors, such as light, water, and nutrients, which regulate lateral root development by influencing intrinsic factors.Citation31–33
BRs as plant hormones that regulate plant height and promote root growth. Moreover, BRs can induce plant tolerance to a variety of biological and abiotic stresses, such as heavy metal stress, salt stress, temperature stress, drought stress, pathogen infection, and oxidative stress.Citation34 Low nitrogen specifically up regulates the transcription level of BRs co-receptor BAK1 to activate BRs signal and stimulate root elongation. The role of BR signal in root elongation under low nitrogen condition was revealed. The BRs signaling kinase BSK3 allele provides a target for improving root growth of crops growing under limited nitrogen conditions.Citation35,Citation36 Given their vital function in plant growth, development, and reproduction, exogenous application of BRs can increase the yield of various plants and improve crop quality.Citation37 BRs is also nontoxic and beneficial to human health, acting as a potent antioxidant and neuroprotective agent in Parkinson’s diseaseCitation38 and may improve keratitis in mice.Citation39 BRs can increase plant yieldCitation40 with transgenic rice possessing increased BR biosynthesis levels showing yield increases of 15–44% per plant; BR can also enhance plant stress tolerance and reduce pesticide use, thus decreasing the amounts of pesticide residues.Citation41–43 In rice, data on genes controlling growth and development downstream of BRs signal transduction are mainly derived from microarray results.Citation44,Citation45 Expression of OsBLE1 increased markedly after BRs treatment, and transferring antisense OsBLE1 into rice inhibits plant growth, indicating that OsBLE1 is involved in BRs-regulated plant growth and development.Citation45Expression levels of OsMPD1 decrease in response to BRs treatment, and transgenic plants lacking OsMPD1are hypersensitive to exogenous BRs, showing that OsMPD1 plays a negative role in BRs signal transduction.Citation44 OsMADS22 and OsMADS55 are homologs of OsMPD1 and have also been shown to play a negative regulatory role in BRs signal transduction [Shinyoung Citation46].
In this study, we used a multi-disciplinary approach based on plant morphology, developmental biology, plant physiology, cell biology, and molecular biology techniques to clarify the regulatory effects of BRs on maize root growth and development and the mechanism by which BRs improves disease resistance to improve maize yield.
Materials and methods
Plant materials and treatments
Maize (Zea mays) cultivar ‘B-73’ seeds of the same size and plumpness were kept at 30°C for 1 day. Root length was measured at two developmental stages. At the first stage, seeds were soaked in distilled water containing different concentrations of 2,4-epibrassinolide and placed on filter paper; root length was measured after germination at 28°C for 36 h. At the second stage, roots from seeds treated with 0, 0.5, 1, 1.5, or 2 mg/L eBL (an active by-product of BRs biosynthesis, which has the ability to stimulate different plant metabolic processes) were measured after planting seeds in soil for 15 days. Roots of maize grown on half-strength Murashige and Skoog (1/2 MS) medium containing 0 or 1 mg/L eBL for 3 days were observed by paraffin sectioning. When maize (Zhengdan 958) seedlings grew to the big bell mouth stage (between jointing and tasseling), 0 or 1 mg/L eBL was sprayed on the aboveground parts, and physiological indexes were measured after 1 week. After 4 weeks, the aerial root growth of maize was observed.
Fusarium infection treatment
Fusarium graminearum and Fusarium proliferatum cultures showing good growth covering the entire surface of the medium were used for fungal inoculation tests. Suspensions of 106 spores/mL were prepared. F.proliferatum was sprayed onto the aboveground parts of maize plants. F.graminearum spores were injected into maize stems at the big bell stage using a syringe. After inoculation, corn seedlings were watered as usual, and samples were taken 7 days or 4 weeks later to observe fungal infection.
Proline content measurement
Fresh leaves (0.5 g) were harvested in a test tube, and 5 mL of 3% sulfosalicylic acid was added. After sealing the test tube with a glass ball, the sample was placed in boiling water for 10 min (shaking constantly to sink the crushed leaves). After cooling to room temperature, 2 mL of supernatant was mixed with 2 mL of glacial acetic acid and 3 mL of chromogenic solution in a fresh test tube (control tube: 2 mL of glacial acetic acid, 3 mL of chromogenic solution, and 2 mL of water) and placed in boiling water for 40 min. Toluene solution (5 mL) was added to each cooled test tube and shaken vigorously to extract the red substance. After standing for 10 min (to allow layers to form), the toluene layer was collected and its optical density (OD) was measured at 520 nm. The content of proline in the sample was calculated according to the formula y = (C* V)/(a * W), where C = 38.869 * OD520 + 0.0839;V = total volume of extract (mL); a = volume of toluene layer absorbed during determination (mL); and W = sample weight (g).
Soluble sugar content measurement
Fresh corn leaves (0.3 g) were harvested into a test tube and mixed thoroughly with 9 mL of water, and the tube was placed in boiling water for 20 min. After cooling, the supernatant was poured off and water was added to the pellet to a final volume of 1 mL.Anthrone reagent (5 mL) was added, and the tube was placed in boiling water for 10 min. After cooling to room temperature, the OD was measured at 600 nm. Soluble sugar content (mg/g) = (C × V × n)/(v × w × 1000), where C = 2.4525 * OD600–0.0036 (C obtained using a standard curve (mg)); V = total volume of sample extract (mL); v = amount of sample added after sample extract (mL); w = fresh weight of sample (g); and n = dilution factor of the sample.
Detection of superoxide dismutase (SOD) activity
A 50-μL aliquot of enzyme extract (5 mL phosphate buffer, pH7.8, 5 mL 0.2 M KNO3) plus 50 μL riboflavin was added to 4 mL of reaction solution (2 mL phosphate buffer, pH7.8, 0.5 mL 100 mM methionine, 1 mL 300 μM NBT, and 0.5 mL 0.8 mM EDTA) in a test tube. Two treatments contained no enzyme solution (replaced with pH7.8 phosphate buffer). Tubes were immediately placed under a 4000 LX fluorescent lamp for light reduction reaction. After 15 min, the reaction was stopped by shading with black paper. Phosphoric acid buffer was used as a zero point, and sample color was compared by measuring OD at 560 nm. SOD activity was calculated according to the formula (control OD560–sample OD560) *dilution factor/ (control OD560*0.5*w*sample dosage during determination), where w = fresh weight of sample (g).
Malondialdehyde (MDA) content measurement
Thiobarbituric acid (TBA; 0.6%) was prepared by dissolving 0.6 g TBA in a small amount of 1 M NaOH by heating and adjusting the volume to 100 mL using 10% trichloroacetic acid (TCA). Enzyme extract (1 mL) and 2 mL of 0.6% TBA were added to a test tube, which was sealed and placed in a boiling water bath for 15 min. The sample was then allowed to stand before centrifuging at 12,000 g for 10 min. The OD of the supernatant was measured at 600, 532, and 450 nm. MDA content was determined using the following formula: MDA (μmol/g fresh weight) = (6.45×(OD532–OD600)–0.56× OD450) ×volume of extract (mL)/fresh weight of plant tissue (g).
Detection of peroxidase (POD) activity
A 20 μL aliquot of enzyme extract (5 mL phosphate buffer, 5 mL 0.2 M KNO3) was mixed thoroughly with 3 mL of reaction solution (2.91 mL of phosphoric acid buffer, pH 7.0, 0.05 mL of guaiacol, 0.02 mL of 40 mM H2O2, 0.02 mL of enzyme solution) in a small tube and placed in a constant temperature water bath at 34°C for 3 min. Subsequently, 20 μL of 20% trichloroacetic acid was added to stop enzyme activity. Optical density was measured at 470 nm using pH7.0 phosphoric acid buffer as a zero point (the reaction solution was precooled in advance). POD activity was calculated according to the formula (sample OD470*total volume of enzyme solution)/(leaf fresh weight (g)*volume of enzyme solution (mL)).
Measurement of chlorophyll content
Fresh leaves (0.2 g) were harvested and mixed with small amounts of quartz sand and calcium carbonate powder and 3 mL of 80% acetone in a mortar. The homogenate was transferred into a 25 mL Brown volumetric flask, and 80% acetone was added until the total volume reached 25 mL. The sample was then mixed and centrifuged at 12,000 g for 10 min. Using 80% acetone reagent as a control, OD was measured at 663 and 645 nm. Chlorophyll content was calculated as chlorophyll concentration * extract volume * dilution factor/sample fresh weight (g).
Measurement of other physiological indexes
Other physiological indexes were measured using commercially available kits (Suzhou COMIN, China) for detection of hydrogen peroxide (Cat#H2O2-2-Y); protein (Cat# BCAP-2-W), crude fat (Cat# TFA-1-Y), and starch (Cat#DF-2-Y) content in grains; and phosphoenolpyruvate carboxylase (PEPC)(Cat#PEPC-2-Y), sucrose synthase (SS)(Cat#SSS-1-Y), and sucrose phosphate synthase (SPS)(Cat#SPS-2-Y) content in leaves.
Stems tiffness measurement
A plant stem strength tester (YYD-1B; Zhejiang Tuopu, China) was used to measure stem lodging resistance of corn following different treatments. The front-end accessories of the plant stem strength tester and half of the corn stem were fixed with a rubber strip. Corn stems were gently pushed at right angles to the ground. Pressure values were recorded when maize stalks tilted to an angle of 45° with the ground.
Reverse-transcription quantitative PCR (RT-qPCR)
Total RNA was isolated using Trizol reagent and digested using DNaseI to remove DNA contamination. cDNA was synthesized using oligo dT as the primer. TUBULIN genes were used as internal controls. Primers used for RT-qPCR are listed in Table S1. The reaction mixture (20 μL) contained 10 μL of SYBR Green Realtime PCR Master Mix (DBI, Bioscience, Germany), 0.5 μM each of forward and reverse primers, and 1 μL of cDNA template (equivalent to 50 ng of total RNA). PCR was performed on an ABI 7500 system using a SYBR Premix Ex Taq (perfect real time) kit (TaKaRa Biomedicals, Dalian, China) under the following conditions: 94°C for 30s and 40 cycles of 94°C for 5s, 58°C for 15s, 72°C for 10s, and 72°C for 7 min for plate reading. Three biological replicates were performed. Expression levels were calculated according to the delta-delta CT method.
Statistical analysis
In the experiments involving statistical analysis, 15 seedlings were measured in each treatment. Three biological replicates were set up and the average value was taken. Each experiment was repeated at least three times, and the significance was analyzed by t-test.
Results
BRs promotes maize primary root growth
Roots absorb water and nutrients from the soil and anchor the plant to prevent lodging. Maize has a fibrous root system, in which the primary root from the embryo forms additional seminal roots; in maize, adventitious roots, called crown roots, also grow from the base of the stem.Citation25 To observe the growth of maize primary roots, seeds of the same size and fullness were germinated at 28°C in distilled water containing no eBL or 1 mg/L eBL. After 2 days of BRs treatment, the primary roots of eBL-treated seeds were markedly longer than that in controls ()), showing that BR could promote the growth of primary roots remarkably. To test the optimum concentration of BRs for regulating seed germination, we performed a germination experiment at 28°C for 36 h in distilled water containing 0, 0.5, 1, or 2 mg/L eBL. Primary roots were longest in distilled water containing 1 mg/L eBL, followed by the seeds germinated in 0.5 and 2 mg/L eBL distilled water, while seeds in distilled water without eBL showed the slowest germination ()). Roots were significantly longer with 1 mg/L eBL treatment than with other treatments; however, the increasing trend of root length with eBL concentration was inhibited under 2 mg/L eBL treatment, demonstrating a typical concentration dependence of eBL treatment in which low concentration promotes and high concentration inhibits root growth. Therefore, these results indicate that BRs plays an important role in the elongation and development of maize primary roots. This is the same as the previous report (https://link.springer.com/article/10.1007/s10725-020-00626-z).
Figure 1. BR regulates the development of primary roots in maize. A, B, morphological observation of primary roots in maize grown under the indicated treatments. Control: 0 mg/L eBL; BR:1 mg/L eBL. Scale bars, 1 cm. C, statistics of primary root length of control(a) and BR(b) treatments. Data were analyzed from 15 seeds for each genotype from 3 experiments. Statistical significance was determined by student’s t-test (two-tailed) in (c). D, germination of maize seeds under 0, 0.5, 1, and 2 mg/L eBL treatments. Scale bars, 1 cm. E, statistics of primary root length after treatment with different concentrations of eBL(d). The letters indicate the significance groups at P < .05 (one-way ANOVA and Tukey test).
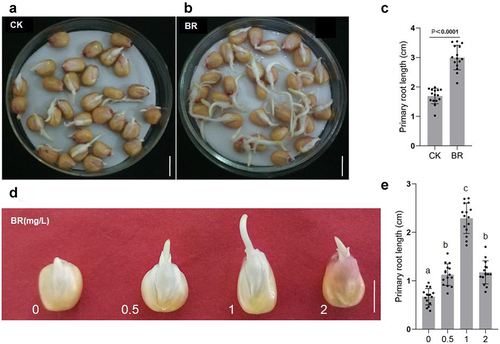
BRs promotes secondary root growth
Next, we tested the effect of BRs on secondary root length. When maize seeds were germinated for 3 days in eBL-containing distilled water, primary roots were obviously longer than those of control seeds. Furthermore, the number of secondary roots produced by eBL-treated seeds was also greater than that of the control ()). In the process of maize root development, secondary roots, which are borne on all morphological roots, form from the embryo root. Hence, we planted maize seeds treated with 0, 0.5, 1, 1.5, or 2 mg/L eBL in soil and observed the growth of secondary roots after 15 days. The number of secondary roots treated with eBL were obviously greater than those in roots not treated with eBL. Lateral roots from seeds treated with 1 mg/L eBL displayed the highest density (), indicating that the development of maize secondary roots was promoted under BRs treatment.
Figure 2. BR regulates the development of maize secondary and aerial roots. A, phenotype of maize secondary roots treated with different concentrations of eBL after 3 days. Scale bar: 1 cm. B, statistics of secondary root number treated with 0 and 1 mg/L eBL. Data were analyzed from 15 seeds for each genotype from 3 experiments. Statistical significance was determined by student’s t-test. P values are indicated. C. Phenotype of the maize secondary roots treated with 0, 0.5, 1, 1.5, and 2 mg/L eBL before planting in the soil after 15 days. Scale bar: 1 cm. D-G, phenotypes of aerial roots of the indicated treatment after 4 weeks of the big bell mouth stage. CK: 0 mg/L eBL (d, f); BR:1 mg/L eBL (e, g). Scale bars, 10 cm. H, I, phenotype of initial development of lateral roots in the indicated treatment after 3 days. CK: 0 mg/L eBL (h); BR:1 mg/L eBL (i). J-M, phenotype of lateral root transection and slitting in the indicated treatment after 3 days. CK: 0 mg/L eBL (j, l); BR:1 mg/L eBL (k, m).
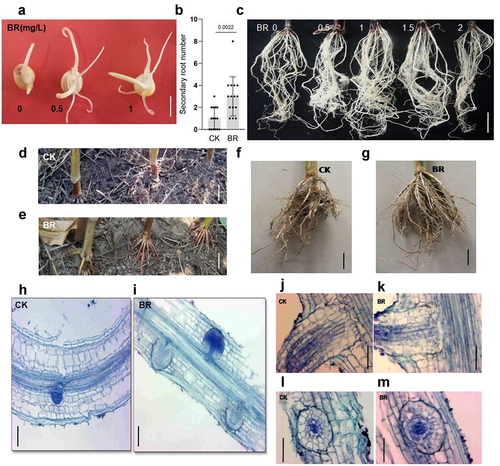
Analysis of paraffin sections of maize primary roots grown on medium containing 0 or 1 mg/L eBL for 3 days showed that roots treated with no eBL produced only one lateral root, while BRs-treated roots had three lateral roots ()), demonstrating that BRs can promote the early development of lateral roots. Further analysis of longitudinal and transverse sections of lateral roots showed that BRs treatment did not change the internal structure of lateral roots ()).The function of BRs in promoting lateral root growth occurred during different growth periods of maize (aboveground or underground). After spraying BRs on the aboveground parts of maize at the big bell mouth stage, the development of underground lateral roots was also significantly promoted ()).
The unique aerial roots of maize provide strong lodging resistance. During the late stage of maize growth, the primary and secondary roots degenerate, and nutrition and water absorption are mainly dependent on aerial roots.Citation47,Citation48 Most importantly, ensuring normal yield under flood and drought conditions is crucial. We sprayed 1 mg/L eBL onto the shoots of maize seedlings with eight leaves to observe the effects of BRs on the growth and development of aerial roots. Aerial roots were observed after 4 weeks. The aerial roots of untreated controls were just beginning to grow; however, the aerial roots of BRs-treated maize had obviously elongated, and aerial roots developed in the previous stem section ()). These results showed that BRs promotes the development of aerial roots in maize.
These results demonstrate that BRs promotes the development of maize primary, secondary, and aerial roots, with 1 mg/L eBL as the optimum concentration for promoting growth.
BRs regulates root development via auxin
To further explore the role of BRs in maize root development, we analyzed the expression patterns of BR-related genes. The BRs biosynthesis genes ZmDWF4Citation49 and ZmCPDCitation50–52 were down-regulated by eBL treatment compared with untreated controls (), which was consistent with previous results in Arabidopsis (Arabidopsis thaliana), suggesting that this experimental treatment is effective.
Figure 3. Expression analysis of key genes regulating root development. A, RT-qPCR of ZmDWF4 and ZmCPD(a); ZmWOX5, ZmBBM1, and ZmBBM2(b); and ZmPIN2, ZmPIN3a, ZmPIN3b, ZmIAA2, ZmIAA30,ZmARF7, and ZmARF19(c)under the indicated treatments. CK: 0 mg/L eBL; BR:1 mg/L eBL. Gene expression in CK was set to 1. Data represent mean ± s.d. of three biological repeats. Statistical significance was determined by two-sided student’s t-test (A, B, C); NS, not significant; P values are indicated. Tubulin was used as an internal reference gene.
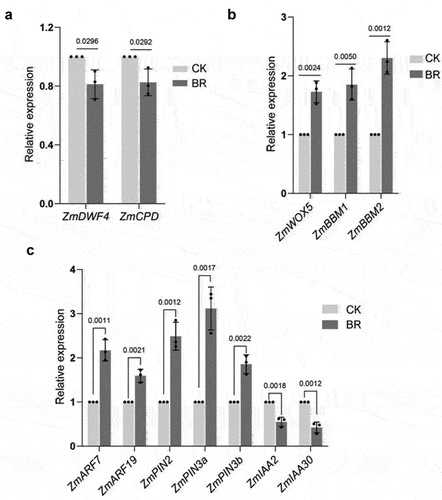
WOX5 is specifically expressed in the quiescent center (QC) cells in the Arabidopsis root meristem and maintains the activity of surrounding stem cells.Citation53,Citation54 The expression of PLT1 and PLT2 is closely related to auxin levels in the root meristem. PLT genes in Arabidopsis are necessary for the formation of the stem cell microenvironment in the root meristem. A PLT protein gradient controls stem cell division and cell differentiation.Citation55,Citation56We examined the expression of WOX5, PLT1 and PLT2 homologs ZmWOX5, ZmBBM1, and ZmBBM2 in maize roots after BRs treatment and found that the expression of ZmWOX5, ZmBBM1, and ZmBBM2 in BRs-treated roots was higher than that in untreated controls ().
Auxin participates in the ubiquitination and degradation of AUX/IAA proteins, releasing auxin response factors. Auxin response factors facilitate auxin-mediated regulation of gene expression.Citation57 Auxin shows a gradient distribution in the root apical meristem, accumulating in the static center and stem cell area and gradually decreasing from the stem cell area to the root tip elongation area. We found that expression of the auxin transport genes ZmPIN2, ZmPIN3a, and ZmPIN3b was higher under BRs treatment than with no treatment ().IAA14 and IAA28 are inhibitors of lateral root primordium initiation,Citation58–61 and their candidate maize homologs, ZmIAA2 and ZmIAA30,Citation62 were down-regulated after BRs treatment(). By contrast, some positive regulatory factors promoting the initiation of lateral root primordia, such as ZmARF7 and ZmARF19,Citation63–66 were up-regulated after BRs treatment (), suggesting that BRs regulates root growth and development through auxin.
BRs enhances the stress tolerance of maize
We detected the SOD, POD, soluble sugar, proline, H2O2, and MDA contents in BRs-treated maize leaves at the big bell stage. SOD, POD, soluble sugar and proline contents in the leaves of maize treated with BRs were higher than those in the control ()). Moreover, the H2O2 and MDA contents of BRs-treated leaves were lower than those of untreated controls ()).At the same time, we examined the expression of ZmSOD, ZmPOD, ZmAPX2, and ZmP5CS after BRs treatment. The expression levels of ZmSOD, ZmPOD, ZmAPX2, and ZmP5CSwere increased compared with those of the control (), suggesting that BRs treatment can enhance the stress tolerance of maize.
Figure 4. BR regulates physiological and biochemical indexes of maize. SOD(a), POD(b), proline(c), soluble sugar(d), H2O2(e), and MDA(f) content under the indicated treatments. CK: 0 mg/L eBL; BR:1 mg/L eBL. G, RT-qPCR of ZmSOD, ZmPOD, ZmAPX2, and ZmP5CS under the indicated treatments. CK: 0 mg/L eBL; BR:1 mg/L eBL. Gene expression in CK was set to 1. Data represent mean ± s.d. of three biological repeats. Statistical significance was determined by two-sided Student’s t-test (a-g); NS, not significant; P values are indicated. Tubulin was used as an internal reference gene.
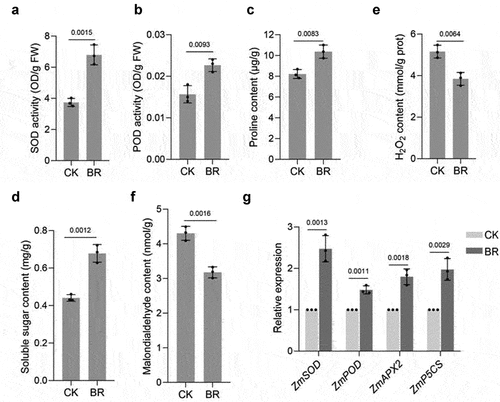
BRs improves the resistance of maize to Fusarium infection
Fusarium graminearum is one of the main pathogenic fungi causing corn stem rot.Citation67 BRs can improve the ability of plants to resist a variety of stresses.Citation68 To determine whether BRs treatment can improve the resistance of maize to F.graminearum, we inoculated maize treated with BRs and untreated maize with F.graminearum. F.graminearum spores were injected into maize stems at the big bell stage using a syringe. After inoculation, and samples were taken 4 weeks later to observe fungal infection. Treated maize stalks showed no obvious differences from the outside. When cut open, however, we found that the untreated maize stalks inoculated with F.graminearum were blackened over a large area, while the BRs-treated stalks had only a small blackened area. The incidence rate of Fusarium infection in plants treated with BRs was significantly lower than that of untreated plants ()). The lodging resistance of untreated plants inoculated with F.graminearum was lower than that of BRs-treated plants. BRs treatment could therefore improve the lodging resistance of plants after Fusarium inoculation ().
Figure 5. BR improved the resistance of maize. A-C, Phenotype of maize stalk under the indicated treatment(B, transaction; C, longitudinal section). Phenotype of maize seedling(f) and leaf(g) under the indicated treatment. Scale bars, 1 cm. The incidence rate(d,h) and lodging resistance(e), Data were analyzed from 15 seeds for each genotype from 3 experiments. Data represent mean ± s.d. of three biological repeats. Statistical significance was determined by two-sided Student’s t-test (D, E, H); NS, not significant; P values are indicated. CK: 0 mg/L eBL; BR:1 mg/L eBL.
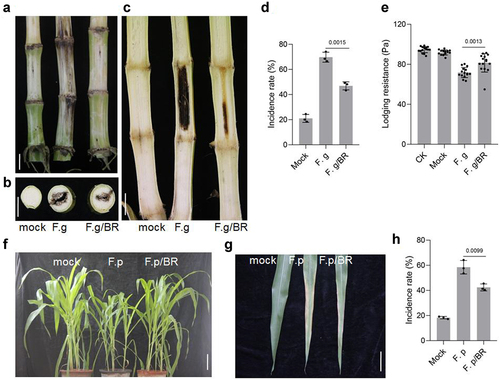
Fusarium proliferatum is one of the main pathogens causing corn sheath rot.Citation69 To determine whether BRs can prevent and control F.proliferatum, we inoculated BR-treated and untreated maize with F.proliferatum. F.proliferatum was sprayed onto the aboveground parts of maize plants, and samples were taken 7 days later to observe fungal infection. Plants infected with F.proliferatum were weaker, with reduced plant height, compared with control plants. The leaves showed large yellow patches. However, after BRs treatment, the incidence of F.proliferatum was low and plant height was similar to that of uninfected plants. Damage to the blade was less severe and plant growth was better than that in untreated plants inoculated with F.proliferatum ()).This suggested that the disease resistance of maize was weakened after being infected by Fusarium, while BRs improved the disease resistance of maize.
BRs improves the photosynthetic performance of maize
To have practical significance, the ideal maize plant must have architecture suited to withstand close planting and possess lodging resistance. Our results showed that BRs promoted an increase in the leaf angle of maize ()). Phosphoenolpyruvate carboxylase (PEPC) improves CO2 utilization and accelerates photosynthesis,Citation70–72 while sucrose phosphate synthase (SPS) is the main enzyme for sucrose production, which is beneficial for storage of organic matter. SPS activity is positively correlated with photosynthetic rate.Citation73–75 Sucrose synthase (SS) catalyzes free fructose and glucose in plants to produce sucrose.Citation76 The activities of SPS and SS are positively correlated with grain filling, total starch, and amylopect in accumulation rate.Citation77 We measured chlorophyll contentCitation78 and PEPC, SS, and SPS activities after spraying maize seedlings with BRs()). Chlorophyll content was slightly higher in BRs-treated plants than in non-BRs-treated plants. The activities of PEPC, SS, and SPS in plants sprayed with BR were significantly higher than those in control plants, indicating that BRs accelerated the photosynthetic rate of maize and the accumulation of organic matter, which promoted grain filling and played an important role in improving the yield and quality of maize.
Figure 6. BR improves the photosynthetic performance of maize. Phenotype of maize leaf angle under CK and eBL treatments(a). Scale bars, 10 cm. And statistical data in leaf angle(b). Chlorophyll(c), PEPC(d), SPS(e), and SS(f) contents. Data represent mean ± s.d. of three biological repeats. Statistical significance was determined by two-sided Student’s t-test (d-f); NS, not significant; P values are indicated. CK: 0 mg/L eBL; BR:1 mg/L eBL.
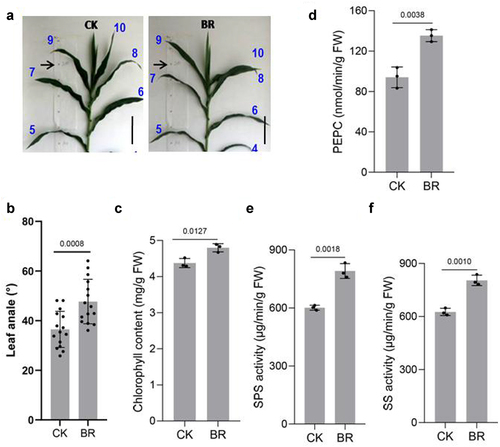
BRs increases the yield of maize
The value of crops depends on yield and quality.Citation79,Citation80 When maize seedlings grew to the big bell mouth stage (between jointing and tasseling), 0 or 1 mg/L eBL was sprayed on the aboveground parts, wait for the grain to mature. We therefore measured the yield of maize treated with BRs. The 1000-grain weight changed little after BRs treatment, but the spike length, number of grains per spike, and yield per mu all increased ()). BRs treatment also increased the contents of protein, fat, and starch in grains ()). This suggested that BR functions in improving grain yield and quality of maize.
Figure 7. BR increases the yield of maize. Phenotype of maize spike length under CK(a) and eBL(b) treatments. Scale bars, 1 cm. The 1000-grain weight(c), spike length(d), grains per spike(e), and the yield per mu(f). Protein(h), fat(g), and starch(i) contents. Data were analyzed from 15 seeds for each genotype from 3 experiments(d, e). Data represent mean ± s.d. of three biological repeats. Statistical significance was determined by two-sided Student’s t-test (c-i); NS, not significant; P values are indicated. CK: 0 mg/L eBL; BR:1 mg/L eBL.
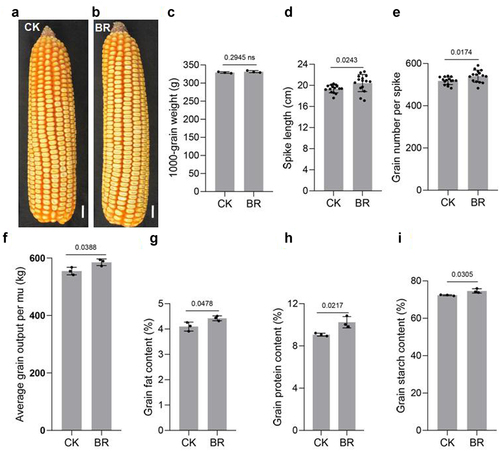
Discussion
Plant hormone treatment is an environmentally friendly, efficient method that is widely used to produce strong, stress-tolerant plants. BRs is an important plant hormone that not only affects plant growth, flowering, and photosynthesisCitation81,Citation82 but also increases the adaptability of plants to various biotic and abiotic stresses.Citation83 Exogenous 0.025 g/L eBL treatment reduces the production of ROS by activating ROS-scavenging enzymes, regulating the plant osmotic state, and improving photosynthesis and other leaf gas exchange properties, thus playing an important role in plant stress responses.Citation84 BRs treatment significantly increased the activities of antioxidant enzymes and enhanced the heat tolerance of kiwifruit seedlings, with 0.3 mg/L eBL being the most effective treatment. Selecting the optimal hormone concentration for spraying is crucial for crop growth and development under stress.Citation84–86
Various abiotic stresses seriously affect maize production. For example, high-temperature stress accelerates leaf senescence, decreases leaf photosynthetic capacity and dry matter accumulation after anthesis, and increases grain abortion, which leads to yield losses.Citation87–89 High-temperature stress before the silking stage significantly decreased the ear diameter and length, grain number per row, and 1000-grain weight of maize varieties Zhengdan 958 and Xianyu 335; it also increased the length of the bald tips of ears and decreased the yields of both varieties. High-temperature stress at the silking stage and grain completion stage significantly decreases the number of grains per ear and grain weight, thereby affecting yield.Citation90 The main reason for this decreased grain number per ear is that high temperature increases the rate of grain abortion. Photosynthesis is blocked and respiration is enhanced under high-temperature stress, resulting in the decreased transport of assimilates from photosynthetic products to grains, which affects their accumulation in grains and grain weight, ultimately decreasing grain yield.Citation91,Citation92
Grain quality in maize is determined by the proportion of starch, protein, and fat and its internal structure.Citation93 High-temperature stress not only reduces the grain yield of maize, but it also affects the grain quality of waxy maize. High-temperature stress increases starch grain size and protein content and decreases the grain/alcohol ratio.Citation94,Citation95 High-temperature stress accelerates grain filling, the degradation of starch in the pericarp, starch enrichment in endosperm cells, and starch accumulation, but the duration of continuous grain filling is shortened and cell division is reduced, resulting in a significant decrease in starch content at maturity.Citation96 High temperature at the grain filling stage decreases the photosynthetic rate, starch synthase activity, and starch content, resulting in starch loss and smaller granules. The decrease in total soluble sugar and starch contents in maize grains under high-temperature stress before and after anthesis is mainly due to the decreased activity of starch biosynthesis-related enzymes such as FBP, SPS, SS, and ADPG, which leads to the deterioration of maize quality.Citation97
Exogenous hormones play important roles in alleviating the effects of high-temperature stress on starch quality. Exogenous application of plant hormones (BRs, methyl jasmonate, and so on) enhances photosynthesis in rice under high-temperature stress, promotes the transport of carbohydrates to young spikelets, increases the seed-setting rate and grain-filling rate of spikelets, and alleviates damage to grain formation caused by high temperature.Citation98,Citation99
Stem rot disease poses a great threat to maize and has become an important factor limiting maize production. Stem rot is a typical soil-borne disease caused by diverse pathogens and is difficult to control, as there is currently no ideal method for doing so.Citation100–102 The breeding of resistant varieties, strengthening field cultivation management, the proper application of fertilizers, and the use of conventional chemicals are effective measures for limiting disease severity.
BRs regulates plant growth and development; therefore, studying the functions of BRs regulatory proteins is important for clarifying their mechanisms of action. To date, few studies have focused on the functions of BRs regulatory proteins, and the mechanism underlying the roles of BRs in regulating plant growth and development are not clear.Citation103–105 There is an urgent need for research and development of regulators that increase yields, stress resistance, and lodging to help solve current food security problems, increase food production, decrease the need for chemical fertilizers and pesticides for food production, improve crop stress resistance, and reduce the loss of production caused by natural disasters.
In this study, BRs treatment promoted the development of primary, lateral, and aerial roots and increased the root area for the absorption of nutrients and water in maize. In addition, BRs treatment enhanced the nitrogen fixation ability of the plant, improved lodging resistance, reduced MDA and hydrogen peroxide contents, and increased the contents of substances that enhance tolerance to various abiotic stresses, such as proline, SOD, and POD. Furthermore, BRs promoted plant disease resistance and ultimately increased the yield of maize (). These results highlight the potential value of BRs treatment for enhancing agricultural production.
Author contributions
J.D. and W.F. designed the experiments. H.Z., Z.T. and D.Z. contributed equally to this work. H.Z., Y.Z., D.Z. and Z.T. carried out all of the experiments with technical support from K.Z. and J.D. and F.W. analyzed data. H.Z., D.Z. and F.W. wrote the manuscript with input from J.D.
Supplemental Material
Download MS Excel (12 KB)Acknowledgments
This research was supported by a grant (ZD2018085) from Science and technology research project of colleges and universities in Hebei province, and funding from Hengshui science and technology program research support project (2021014010Z).
Disclosure statement
No potential conflict of interest was reported by the author(s).
Supplementary material
Supplemental data for this article can be accessed online at https://doi.org/10.1080/15592324.2022.2095139
Additional information
Funding
References
- Lynch J. Root architecture and plant productivity. Plant Physiol. 1995;109(1):7–14. doi:10.1104/pp.109.1.7.
- Zhang C, Xue J, Cheng D, Feng Y, Liu Y, Aly HM, Li Z. Uptake, translocation and distribution of three veterinary antibiotics in Zea mays L. In: Environmental pollution (Barking, Essex: 1987). 2019;Vol. 250:47–57. doi:10.1016/j.envpol.2019.03.110.
- Niki E, Yoshida Y, Saito Y, Noguchi N. Lipid peroxidation: mechanisms, inhibition, and biological effects. Biochemical and Biophysical Research Communications. 2005;338(1):668–676. doi:10.1016/j.bbrc.2005.08.072.
- Reilly CA, Aust SD (2001) Measurement of lipid peroxidation. Current protocols in toxicology Chapter 2:Unit 2.4. doi:10.1002/0471140856.tx0204s00
- Ayala A, Muñoz MF, Argüelles S. Lipid peroxidation: production, metabolism, and signaling mechanisms of malondialdehyde and 4-hydroxy-2-nonenal. Oxidative Medicine and Cellular Longevity. 2014;2014:360438. doi:10.1155/2014/360438.
- Del Rio D, Stewart AJ, Pellegrini N. A review of recent studies on malondialdehyde as toxic molecule and biological marker of oxidative stress. Nutrition, Metabolism, and Cardiovascular Diseases: NMCD. 2005;15(4):316–328. doi:10.1016/j.numecd.2005.05.003.
- Draper HH, Hadley M. Malondialdehyde determination as index of lipid peroxidation. Methods in Enzymology. 1990;186:421–431. doi:10.1016/0076-6879(90)86135-i.
- Miao L, St Clair DK. Regulation of superoxide dismutase genes: implications in disease. Free Radic Biol Med. 2009;47(4):344–356. doi:10.1016/j.freeradbiomed.2009.05.018.
- Warner HR. Superoxide dismutase, aging, and degenerative disease. Free Radic Biol Med. 1994;17(3):249–258. doi:10.1016/0891-5849(94)90080-9.
- Anjum NA, Sharma P, Gill SS, Hasanuzzaman M, Khan EA, Kachhap K, Mohamed AA, Thangavel P, Devi GD, Vasudhevan P, et al. Catalase and ascorbate peroxidase-representative H2O2-detoxifying heme enzymes in plants. Environmental Science and Pollution Research International. 2016;23(19):19002–19029. doi:10.1007/s11356-016-7309-6.
- Del Río LA. ROS and RNS in plant physiology: an overview. J Exp Bot. 2015;66(10):2827–2837. doi:10.1093/jxb/erv099.
- Ishikawa T, Uchimiya H, Kawai-Yamada M. The role of plant Bax inhibitor-1 in suppressing H2O2-induced cell death. Methods in Enzymology. 2013;527:239–256. doi:10.1016/b978-0-12-405882-8.00013-1.
- Kuo EY, Cai MS, Lee TM. Ascorbate peroxidase 4 plays a role in the tolerance of Chlamydomonas reinhardtii to photo-oxidative stress. Sci Rep. 2020;10(1):13287. doi:10.1038/s41598-020-70247-z.
- Li H, Liu H, Wang Y, Teng RM, Liu J, Lin S, Zhuang J. Cytosolic ascorbate peroxidase 1 modulates ascorbic acid metabolism through cooperating with nitrogen regulatory protein P-II in tea plant under nitrogen deficiency stress. Genomics. 2020;112(5):3497–3503. doi:10.1016/j.ygeno.2020.06.025.
- Funck D, Baumgarten L, Stift M, von Wirén N, Schönemann L. Differential contribution of P5CS isoforms to stress tolerance in Arabidopsis. Front Plant Sci. 2020;11:565134. doi:10.3389/fpls.2020.565134.
- Rai AN, Penna S. Molecular evolution of plant P5CS gene involved in proline biosynthesis. Molecular Biology Reports. 2013;40(11):6429–6435. doi:10.1007/s11033-013-2757-2.
- Sami F, Siddiqui H, Hayat S. Interaction of glucose and phytohormone signaling in plants. Plant Physiol Biochem: PPB. 2019;135:119–126. doi:10.1016/j.plaphy.2018.11.005.
- Sun Y, Hou M, Mur LAJ, Yang Y, Zhang T, Xu X, Huang S, Tong H. Nitrogen drives plant growth to the detriment of leaf sugar and steviol glycosides metabolisms in Stevia (Stevia rebaudiana Bertoni). Plant Physiol Biochem: PPB. 2019;141:240–249. doi:10.1016/j.plaphy.2019.06.008.
- Brunner I, Herzog C, Galiano L, Gessler A. Plasticity of fine-root traits under long-term irrigation of a water-limited scots pine forest. Front Plant Sci. 2019;10:701. doi:10.3389/fpls.2019.00701.
- Maurel C, Nacry P. Root architecture and hydraulics converge for acclimation to changing water availability. Nat Plants. 2020;6(7):744–749. doi:10.1038/s41477-020-0684-5.
- Papst C, Bohn M, Utz HF, Melchinger AE, Klein D, Eder J. QTL mapping for European corn borer resistance (Ostrinia nubilalis Hb.), agronomic and forage quality traits of testcross progenies in early-maturing European maize (Zea mays L.) germplasm. TAG Theoretical and Applied Genetics Theoretische Und Angewandte Genetik. 2004;108(8):1545–1554. doi:10.1007/s00122-003-1579-3.
- Ageeva EV, Leonova IN, Likhenko IE. Lodging in wheat: genetic and environmental factors and ways of overcoming. Vavilovskii zhurnal genetiki i selektsii. 2020;24(4):356–362. doi:10.18699/vj20.628.
- Boerjan W, Ralph J, Baucher M. Lignin biosynthesis. Annu Rev Plant Biol. 2003;54:519–546. doi:10.1146/annurev.arplant.54.031902.134938.
- Zhang W, Wu L, Wu X, Ding Y, Li G, Li J, Weng F, Liu Z, Tang S, Ding C, et al. Lodging resistance of japonica rice (Oryza Sativa L.): morphological and Anatomical traits due to top-dressing nitrogen application rates. Rice (New York, NY). 2016;9(1):31. doi:10.1186/s12284-016-0103-8.
- Osmont KS, Sibout R, Hardtke CS. Hidden branches: developments in root system architecture. Annu Rev Plant Biol. 2007;58:93–113. doi:10.1146/annurev.arplant.58.032806.104006.
- Malamy JE, Ryan KS. Environmental regulation of lateral root initiation in Arabidopsis. Plant Physiol. 2001;127:899–909.
- Nibau C, Gibbs DJ, Coates JC. Branching out in new directions: the control of root architecture by lateral root formation. The New phytologist. 2008;179(3):595–614. doi:10.1111/j.1469-8137.2008.02472.x.
- Casimiro I, Marchant A, Bhalerao RP, Beeckman T, Dhooge S, Swarup R, Graham N, Inzé D, Sandberg G, Casero PJ, et al. Auxin transport promotes Arabidopsis lateral root initiation. Plant Cell. 2001;13(4):843–852. doi:10.1105/tpc.13.4.843.
- Malamy JE. Intrinsic and environmental response pathways that regulate root system architecture. Plant Cell Environ. 2005;28(1):67–77. doi:10.1111/j.1365-3040.2005.01306.x.
- Malamy JE, Benfey PN. Organization and cell differentiation in lateral roots of Arabidopsis thaliana. Development (Cambridge England). 1997;124:33–44.
- Desnos T. Root branching responses to phosphate and nitrate. Curr Opin Plant Biol. 2008;11(1):82–87. doi:10.1016/j.pbi.2007.10.003.
- Iyer-Pascuzzi A, Simpson J, Herrera-Estrella L, Benfey PN. Functional genomics of root growth and development in Arabidopsis. Curr Opin Plant Biol. 2009;12(2):165–171. doi:10.1016/j.pbi.2008.11.002.
- Péret B, De Rybel B, Casimiro I, Benková E, Swarup R, Laplaze L, Beeckman T, Bennett MJ. Arabidopsis lateral root development: an emerging story. Trends in Plant Science. 2009;14(7):399–408. doi:10.1016/j.tplants.2009.05.002.
- Castorina G, Consonni G. The role of brassinosteroids in controlling plant height in Poaceae: a genetic perspective. Int J Mol Sci. 2020;21(4):1191. doi:10.3390/ijms21041191.
- Devi LL, Pandey A, Gupta S, Singh AP. The interplay of auxin and brassinosteroid signaling tunes root growth under low and different nitrogen forms. Plant Physiol. 2022. doi:10.1093/plphys/kiac157.
- Jia Z, Giehl RFH, Meyer RC, Altmann T, von Wirén N. Natural variation of BSK3 tunes brassinosteroid signaling to regulate root foraging under low nitrogen. Nat Commun. 2019;10(1):2378. doi:10.1038/s41467-019-10331-9.
- Divi UK, Krishna P. Brassinosteroid: a biotechnological target for enhancing crop yield and stress tolerance. N Biotechnol. 2009;26(3–4):131–136. doi:10.1016/j.nbt.2009.07.006.
- Carange J, Longpré F, Daoust B, Martinoli MG. 24-Epibrassinolide, a phytosterol from the brassinosteroid family, protects dopaminergic cells against MPP-Induced oxidative stress and apoptosis. J Toxicol. 2011;2011:392859. doi:10.1155/2011/392859.
- Michelini FM, Berra A, Alché LE. The in vitro immunomodulatory activity of a synthetic brassinosteroid analogue would account for the improvement of herpetic stromal keratitis in mice. The Journal of Steroid Biochemistry and Molecular Biology. 2008;108(1–2):164–170. doi:10.1016/j.jsbmb.2007.10.002.
- Wu CY, Trieu A, Radhakrishnan P, Kwok SF, Harris S, Zhang K, Wang J, Wan J, Zhai H, Takatsuto S, et al. Brassinosteroids regulate grain filling in rice. Plant Cell. 2008;20(8):2130–2145. doi:10.1105/tpc.107.055087.
- Deng XG, Zhu T, Peng XJ, Xi DH, Guo H, Yin Y, Zhang DW, Lin HH. Role of brassinosteroid signaling in modulating Tobacco mosaic virus resistance in Nicotiana benthamiana. Sci Rep. 2016;6:20579. doi:10.1038/srep20579.
- Deng XG, Zhu T, Zhang DW, Lin HH. The alternative respiratory pathway is involved in brassinosteroid-induced environmental stress tolerance in Nicotiana benthamiana. J Exp Bot. 2015;66(20):6219–6232. doi:10.1093/jxb/erv328.
- Wei LJ, Deng XG, Zhu T, Zheng T, Li PX, Wu JQ, Zhang DW, Lin HH. Ethylene is involved in brassinosteroids induced alternative respiratory pathway in cucumber (Cucumis sativus l.) seedlings response to abiotic stress. Front Plant Sci. 2015;6:982. doi:10.3389/fpls.2015.00982.
- Duan K, Li L, Hu P, Xu SP, Xu ZH, Xue HW. A brassinolide-suppressed rice MADS-box transcription factor, OsMDP1, has a negative regulatory role in BR signaling. Plant Journal. 2006;47:519–531.
- Yang GX, Komatsu S. Molecular cloning and characterization of a novel brassinolide enhanced gene OsBLE1 in Oryza sativa seedlings. Plant Physiology and Biochemistry. 2004;42:1–6.
- Lee S, Chul Choi S, Gynheung A. Rice SVP-group MADS-box proteins, OsMADS22 and OsMADS55, are negative regulators of brassinosteroid responses. The Plant Journal. 2008;54:93–105.
- Reneau JW, Khangura RS, Stager A, Erndwein L, Weldekidan T, Cook DD, Dilkes BP, Sparks EE. Maize brace roots provide stalk Anchorage. Plant Direct. 2020;4(11):e00284. doi:10.1002/pld3.284.
- Zhang Z, Zhang X, Lin Z, Wang J, Xu M, Lai J, Yu J, Lin Z. The genetic architecture of nodal root number in maize. The Plant Journal: for Cell and Molecular Biology. 2018;93(6):1032–1044. doi:10.1111/tpj.13828.
- Sakaguchi J, Watanabe Y. Light perception in aerial tissues enhances DWF4 accumulation in root tips and induces root growth. Sci Rep. 2017;7(1):1808. doi:10.1038/s41598-017-01872-4.
- Corvalán C, Choe S. Identification of brassinosteroid genes in Brachypodium distachyon. BMC Plant Biology. 2017;17(1):5. doi:10.1186/s12870-016-0965-3.
- Müssig C, Fischer S, Altmann T. Brassinosteroid-regulated gene expression. Plant Physiol. 2002;129(3):1241–1251. doi:10.1104/pp.011003.
- Si J, Sun Y, Wang LU, Qin Y, Wang C, Wang X. Functional analyses of Populus euphratica brassinosteroid biosynthesis enzyme genes DWF4 (PeDWF4) and CPD (PeCPD) in the regulation of growth and development of Arabidopsis thaliana. Journal of Biosciences. 2016;41(4):727–742. doi:10.1007/s12038-016-9635-8.
- Kong X, Lu S, Tian H, Ding Z. WOX5 is Shining in the Root Stem Cell Niche. Trends in Plant Science. 2015;20(10):601–603. doi:10.1016/j.tplants.2015.08.009.
- Stahl Y, Simon R. Is the Arabidopsis root niche protected by sequestration of the CLE40 signal by its putative receptor ACR4? Plant Signaling & Behavior. 2009;4(7):634–635. doi:10.4161/psb.4.7.8970.
- Aida M, Beis D, Heidstra R, Willemsen V, Blilou I, Galinha C, Nussaume L, Noh YS, Amasino R, Scheres B. The PLETHORA genes mediate patterning of the Arabidopsis root stem cell niche. Cell. 2004;119(1):109–120. doi:10.1016/j.cell.2004.09.018.
- Galinha C, Hofhuis H, Luijten M, Willemsen V, Blilou I, Heidstra R, Scheres B. PLETHORA proteins as dose-dependent master regulators of Arabidopsis root development. Nature. 2007;449(7165):1053–1057. doi:10.1038/nature06206.
- Sabatini S, Beis D, Wolkenfelt H, Murfett J, Guilfoyle T, Malamy J, Benfey P, Leyser O, Bechtold N, Weisbeek P, et al. An auxin-dependent distal organizer of pattern and polarity in the Arabidopsis root. Cell. 1999;99(5):463–472. doi:10.1016/s0092-8674(00)81535-4.
- Goh T, Kasahara H, Mimura T, Kamiya Y, Fukaki H. Multiple AUX/IAA-ARF modules regulate lateral root formation: the role of Arabidopsis SHY2/IAA3-mediated auxin signalling. Philosophical Transactions of the Royal Society of London Series B, Biological Sciences. 2012;367(1595):1461–1468. doi:10.1098/rstb.2011.0232.
- Kim H, Park PJ, Hwang HJ, Lee SY, Oh MH, Kim SG. Brassinosteroid signals control expression of the AXR3/IAA17 gene in the cross-talk point with auxin in root development. Bioscience, Biotechnology, and Biochemistry. 2006;70(4):768–773. doi:10.1271/bbb.70.768.
- Olmo R, Cabrera J, Díaz-Manzano FE, Ruiz-Ferrer V, Barcala M, Ishida T, García A, Andrés MF, Ruiz-Lara S, Verdugo I, et al. Root-knot nematodes induce gall formation by recruiting developmental pathways of post-embryonic organogenesis and regeneration to promote transient pluripotency. New Phytol. 2020;227(1):200–215. doi:10.1111/nph.16521.
- Rinaldi MA, Liu J, Enders TA, Bartel B, Strader LC. A gain-of-function mutation in IAA16 confers reduced responses to auxin and abscisic acid and impedes plant growth and fertility. Plant Molecular Biology. 2012;79(4–5):359–373. doi:10.1007/s11103-012-9917-y.
- Ludwig Y, Berendzen KW, Xu C, Piepho HP, Hochholdinger F. Diversity of stability, localization, interaction and control of downstream gene activity in the Maize Aux/IAA protein family. PloS one. 2014;9(9):e107346. doi:10.1371/journal.pone.0107346.
- Lee HW, Cho C, Pandey SK, Park Y, Kim MJ, Kim J. LBD16 and LBD18 acting downstream of ARF7 and ARF19 are involved in adventitious root formation in Arabidopsis. BMC Plant Biology. 2019;19(1):46. doi:10.1186/s12870-019-1659-4.
- Lee HW, Kim NY, Lee DJ, Kim J. LBD18/ASL20 regulates lateral root formation in combination with LBD16/ASL18 downstream of ARF7 and ARF19 in Arabidopsis. Plant Physiol. 2009;151(3):1377–1389. doi:10.1104/pp.109.143685.
- Okushima Y, Fukaki H, Onoda M, Theologis A, Tasaka M. ARF7 and ARF19 regulate lateral root formation via direct activation of LBD/ASL genes in Arabidopsis. Plant Cell. 2007;19(1):118–130. doi:10.1105/tpc.106.047761.
- Okushima Y, Overvoorde PJ, Arima K, Alonso JM, Chan A, Chang C, Ecker JR, Hughes B, Lui A, Nguyen D, et al. Functional genomic analysis of the AUXIN RESPONSE FACTOR gene family members in Arabidopsis thaliana: unique and overlapping functions of ARF7 and ARF19. Plant Cell. 2005;17(2):444–463. doi:10.1105/tpc.104.028316.
- Cao Y, Zhang J, Han S, Xia L, Ma J, Wang L, Li H, Yang L, Sun S, Zhu Z, et al. First report of maize stalk rot caused by Fusarium kyushuense in China. Plant Dis. 2021;105(11):3759. doi:10.1094/pdis-11-20-2342-pdn.
- Jager CE, Symons GM, Ross JJ, Reid JB. Do brassinosteroids mediate the water stress response? Physiologia Plantarum. 2008;133(2):417–425. doi:10.1111/j.1399-3054.2008.01057.x.
- Gaige AR, Todd T, Stack JP. Interspecific competition for colonization of maize plants between Fusarium proliferatum and Fusarium verticillioides. Plant Dis. 2020;104(8):2102–2110. doi:10.1094/pdis-09-19-1964-re.
- Cheng G, Wang L, Lan H. Cloning of PEPC-1 from a C4 halophyte Suaeda aralocaspica without Kranz anatomy and its recombinant enzymatic activity in responses to abiotic stresses. Enzyme and Microbial Technology. 2016;83:57–67. doi:10.1016/j.enzmictec.2015.11.006.
- Jeanneau M, Vidal J, Gousset-DuPont A, Lebouteiller B, Hodges M, Gerentes D, Perez P. Manipulating PEPC levels in plants. J Exp Bot. 2002;53(376):1837–1845. doi:10.1093/jxb/erf061.
- Ueno Y, Yoshizawa-Kumagaye K, Emura J, Urabe T, Yoshiya T, Furumoto T, Izui K. Vivo phosphorylation: development of specific antibodies to detect the phosphorylated PEPC isoform for the C4 photosynthesis in zea mays. Methods in Mol Biol (Clifton, NJ). 2020;2072:217–240. doi:10.1007/978-1-4939-9865-4_18.
- Anur RM, Mufithah N, Sawitri WD, Sakakibara H, Sugiharto B. Overexpression of sucrose phosphate synthase enhanced sucrose content and biomass production in transgenic sugarcane. Plants (Basel, Switzerland). 2020;9(2). doi:10.3390/plants9020200.
- Bilska-Kos A, Mytych J, Suski S, Magoń J, Ochodzki P, Zebrowski J. Sucrose phosphate synthase (SPS), sucrose synthase (SUS) and their products in the leaves of Miscanthus × giganteus and Zea mays at low temperature. Planta. 2020;252(2):23. doi:10.1007/s00425-020-03421-2.
- Huang T, Luo X, Wei M, Shan Z, Zhu Y, Yang Y, Fan Z. Molecular cloning and expression analysis of sucrose phosphate synthase genes in cassava (Manihot esculenta Crantz). Sci Rep. 2020;10(1):20707. doi:10.1038/s41598-020-77669-9.
- Schmölzer K, Gutmann A, Diricks M, Desmet T, Nidetzky B. Sucrose synthase: a unique glycosyltransferase for biocatalytic glycosylation process development. Biotechnol Adv. 2016;34(2):88–111. doi:10.1016/j.biotechadv.2015.11.003.
- Verma AK, Upadhyay SK, Verma PC, Solomon S, Singh SB. Functional analysis of sucrose phosphate synthase (SPS) and sucrose synthase (SS) in sugarcane (Saccharum) cultivars. Plant Biol (Stuttgart, Germany). 2011;13(2):325–332. doi:10.1111/j.1438-8677.2010.00379.x.
- Hörtensteiner S, Kräutler B. Chlorophyll breakdown in higher plants. Biochimica et biophysica acta 1807. 2011;8:977–988. doi:10.1016/j.bbabio.2010.12.007.
- Milenković L, Mastilović J, Kevrešan Ž, Bajić A, Gledić A, Stanojević L, Cvetković D, Šunić L, Ilić ZS. Effect of shading and grafting on yield and quality of tomato. Journal of the Science of Food and Agriculture. 2020;100(2):623–633. doi:10.1002/jsfa.10057.
- Smith MR, Rao IM, Merchant A. Source-sink relationships in crop plants and their influence on yield development and nutritional quality. Front Plant Sci. 2018;9:1889. doi:10.3389/fpls.2018.01889.
- Fridman Y, Savaldi-Goldstein S. Brassinosteroids in growth control: how, when and where. Plant Science: an International Journal of Experimental Plant Biology. 2013;209:24–31. doi:10.1016/j.plantsci.2013.04.002.
- Jaillais Y, Vert G. Brassinosteroids, gibberellins and light-mediated signalling are the three-way controls of plant sprouting. Nature Cell Biology. 2012;14(8):788–790. doi:10.1038/ncb2551.
- Cheng L, Sun RR, Wang FY, Peng Z, Kong FL, Wu J, Cao JS, Lu G. Spermidine affects the transcriptome responses to high temperature stress in ripening tomato fruit. J Zhejiang Univ Sci B. 2012;13(4):283–297. doi:10.1631/jzus.B1100060.
- Tanveer M, Shahzad B, Sharma A, Biju S, Bhardwaj R. 24-Epibrassinolide; an active brassinolide and its role in salt stress tolerance in plants: a review. Plant Physiol Biochem: PPB. 2018;130:69–79. doi:10.1016/j.plaphy.2018.06.035.
- Krishna P, Prasad BD, Rahman T. Brassinosteroid action in plant abiotic stress tolerance. Methods in Mol Biol (Clifton, NJ). 2017;1564:193–202. doi:10.1007/978-1-4939-6813-8_16.
- Li B, Zhang C, Cao B, Qin G, Wang W, Tian S. Brassinolide enhances cold stress tolerance of fruit by regulating plasma membrane proteins and lipids. Amino Acids. 2012;43(6):2469–2480. doi:10.1007/s00726-012-1327-6.
- Cairns JE, Sonder K, Zaidi PH, Verhulst N, Prasanna BM. Maize production in a changing climate: impacts, adaptation, and mitigation strategies. Adv Agron. 2012;114.
- Cicchino M, Edreira JIR, Uribelarrea M, Otegui ME. Heat stress in field-grown maize: response of physiological determinants of grain yield. Crop Science. 2010;50(4):1438–1448. doi:10.2135/cropsci2009.10.0574.
- Edreira JIR, Otegui ME. Heat stress in temperate and tropical maize hybrids: differences in crop growth, biomass partitioning and reserves use. Field Crops Res. 2012;130:87–98. doi:10.1016/j.fcr.2012.02.009.
- Wang Y, Tao H, Tian B, Sheng D, Pu W. Flowering dynamics, pollen, and pistil contribution to grain yield in response to high temperature during maize flowering. Environmental and Experimental Botany. 2018;158.
- Arshad MS, Farooq M, Asch F, Krishna JSV, Prasad PVV, Siddique KHM. Thermal stress impacts reproductive development and grain yield in rice. Plant Physiol Biochem: PPB. 2017;115:57–72. doi:10.1016/j.plaphy.2017.03.011.
- Fang J, Zhu W, Tong Y. Knock-down the expression of brassinosteroid receptor tabri1 reduces photosynthesis, tolerance to high light and high temperature stresses and grain yield in wheat. Plants (Basel, Switzerland). 2020;9(7). doi:10.3390/plants9070840.
- Bibb JL, Cook D, Catchot A, Musser F, Stewart SD, Leonard BR, Buntin GD, Kerns D, Allen TW, Gore J. Impact of corn earworm (Lepidoptera: Noctuidae) on field corn (poales: Poaceae) yield and grain quality. J Econ Entomol. 2018;111(3):1249–1255. doi:10.1093/jee/toy082.
- Lu D, Sun X, Yan F, Xin W, Lu W. Effects of heat stress at different grain-filling phases on the grain yield and quality of waxy maize. Cereal Chemistry. 2014;91:189–194.
- Starch composition and its granules distribution in wheat grains in relation to post-anthesis high temperature and drought stress treatments. Starch - Starke. 2014;66(5–6):419–428.
- Wang H, Huang Y, Xiao Q, Huang X, Li C, Gao X, Wang Q, Xiang X, Zhu Y, Wang J, et al. Carotenoids modulate kernel texture in maize by influencing amyloplast envelope integrity. Nat Commun. 2020;11(1):5346. doi:10.1038/s41467-020-19196-9.
- Kumari A, Kumar RR, Singh JP, Verma P, Singh GP, Chinnusamy V, Praveen S, Goswami S. Characterization of the starch synthase under terminal heat stress and its effect on grain quality of wheat. 3 Biotech. 2020;10(12):531. doi:10.1007/s13205-020-02527-4.
- You C, Zhu H, Xu B, Huang W, Wang S, Ding Y, Liu Z, Li G, Chen L, Ding C, et al. Effect of removing superior spikelets on grain filling of inferior spikelets in rice. Front Plant Sci. 2016;7:1161. doi:10.3389/fpls.2016.01161.
- Zhai L, Wang F, Yan A, Liang C, Wang S, Wang Y, Xu J. Pleiotropic effect of GNP1 underlying grain number per panicle on sink, source and flow in rice. Front Plant Sci. 2020;11:933. doi:10.3389/fpls.2020.00933.
- Ochoa J, Fonseca G, Ellis MA. First report of Fusarium wilt of babaco (Carica × heilbornii var. pentagona) in Ecuador. Plant Dis. 2000;84(2):199. doi:10.1094/pdis.2000.84.2.199a.
- Xia B, Liang Y, Hu J, Yan X, Yin L, Chen Y, Hu J, Zhang D, Wu Y. First report of sea buckthorn stem wilt caused by Fusarium sporotrichioides in Gansu, China. Plant Dis. 2021. doi:10.1094/pdis-03-21-0627-pdn.
- Yang G, Komatsu S. Molecular cloning and characterization of a novel brassinolide enhanced gene OsBLE1 in Oryza sativa seedlings. Plant Physiol Biochem: PPB. 2004;42(1):1–6. doi:10.1016/j.plaphy.2003.10.001.
- Müssig C. Brassinosteroid-promoted growth. Plant biology (Stuttgart, Germany). 2005;7(2):110–117. doi:10.1055/s-2005-837493.
- Ye H, Li L, Yin Y. Recent advances in the regulation of brassinosteroid signaling and biosynthesis pathways. Journal of Integrative Plant Biology. 2011;53(6):455–468. doi:10.1111/j.1744-7909.2011.01046.x.
- Zhao B, Li J. Regulation of brassinosteroid biosynthesis and inactivation. Journal of Integrative Plant Biology. 2012;54(10):746–759. doi:10.1111/j.1744-7909.2012.01168.x.