ABSTRACT
Green fluorescent protein (GFP) and its derivatives are extensively used for labeling cells, monitoring gene expression and/or tracking the localization or interactions of proteins. Previous reports of detrimental effects of fluorescent protein (FP) expression include cytotoxicity and interference with fusion protein function or localization. Only a few studies have documented the fluorescent tag-specific effects in plants. Here, we show that placing an enhanced yellow FP (EYFP) tag on the amino-terminus of GLABRA2 (GL2) and PROTODERMAL FACTOR2 (PDF2), two developmentally important HD-Zip IV transcription factors from Arabidopsis, enhances their protein stability. Additionally, expression of EYFP:GL2 not only rescued the gl2 null mutant but also resulted in the abnormal development of abaxially curled leaves associated with EYFP-tag induced GL2 overexpression. Our study raises concerns on the use of FPs regarding their effects on the native properties of target proteins as well as biological consequences of fusion protein expression on morphology.
Introduction
First observed in the jellyfish Aequorea victoria in 1962,Citation1,Citation2 green fluorescent protein (GFP) and its derivatives have revolutionized the molecular biosciences. Using fluorescent proteins (FPs) as tags, it is possible to visualize proteins and to mark organelles, intact cells or whole organisms. FPs are used extensively for analyzing gene expression, reporting promoter activity, monitoring protein subcellular localization, studying protein-protein interactions, protein purification, designing biosensors or cell markers, and lineage tracing.Citation3–6 Their widespread popularity is based on fast and spontaneous formation of chromophores by posttranslational folding in the presence of oxygen without the need of any additional cofactors, substrates, or enzymes.Citation7 FPs are generally compatible in fusion proteins, resistant to proteolytic enzymes and can be easily visualized with standard filters.Citation8,Citation9
Despite their above-mentioned useful properties, limitations of FPs have been increasingly recognized. GFP and its homologs are ~28 kDa proteins that form an eleven stranded beta-barrel structure with a length of ~4.2 nm and a diameter of ~2.4 nm.Citation1,Citation10,Citation11 Addition of these relatively bulky tags can potentially interfere with protein folding and function as well as trafficking of proteins. However, limited evidence is available for such effects of FPs on fusion proteins. Some undesirable properties of FPs include their propensity to oligomerize, pH sensitivity, photobleaching, and overlap of their spectral signals with autofluorescence of cellular components.Citation12–17 Their expression can also have deleterious effects on cells, as exemplified by the formation of cytotoxic aggregates,Citation6,Citation12,Citation18 induction of apoptosis,Citation19,Citation20 and organ pathology in mice.Citation21,Citation22 One report showed that GFP expression in endothelial cells causes transcriptional upregulation of a heat shock protein, resulting in induction of cyclooxygenase-2, prostaglandin production and an increase in regional blood flow.Citation23 In Arabidopsis, the presence of GFP at the amino- or carboxyl-terminus of a virus movement protein sterically hindered its ordered aggregation into tubules required for virus spread.Citation24 Similarly, overexpression of GFP fused to the actin-binding domain of mouse talin (GFP:mTalin), which is typically used to study the actin cytoskeleton, attenuated blue-light-induced chloroplast movements in Arabidopsis leaves.Citation25 In a separate study, overexpression of the same fusion protein caused a reduction in actin depolymerization, resulting in severe defects in root hair expansion.Citation26
Here, we report the effects of enhanced yellow fluorescent protein (EYFP) tags on class IV homeodomain leucine-zipper (HD-Zip IV) transcription factors from Arabidopsis. These regulatory proteins are specific to epidermal or sub-epidermal cells of plants and play an important role in differentiation of these cell layers, anthocyanin accumulation, lipid transport, cuticle biosynthesis and responses to stress (Citation27–30 reviewed inCitation31). GLABRA2 (GL2), the founding member of this family from Arabidopsis, is expressed in developing trichomes and neighboring epidermal cells in leaves during early stages of tricome development.Citation32,Citation33 Later, GL2 is mainly expressed in trichomes and is required for the subsequent stages of their morphogenesis including cell expansion, branching and maturation of the trichome cell wall.Citation33 GL2 is also preferentially expressed in the differentiating hairless cells in the root and hypocotyl epidermisCitation34–36 and is involved in the inhibition of root hair initiation in non-hair cell files.Citation34,Citation36,Citation37 It also regulates mucilage biosynthesis in the seed coat.Citation38,Citation39 Another HD-Zip IV member, PROTODERMAL FACTOR2 (PDF2), functions redundantly with Arabidopsis thaliana MERISTEM LAYER1 (ATML1) in shoot epidermal differentiation during embryonic development.Citation40,Citation41 Both genes positively regulate the expression of a subset of genes specific to the outermost layer (L1) of the shoot apical meristem.Citation28,Citation40,Citation42,Citation43
In numerous previous studies, FP tags were translationally fused to HD-Zip IV TFs to study their tissue-specific gene expression patterns, determine protein subcellular localization, and analyze mutant variants.Citation30, Citation44–48 In particular, our previous work demonstrated that an EYFP-tagged GL2 protein rescues the null mutant phenotype of gl2 plants, including rescue of defects in three different cell types: trichomes, non-hair root epidermal cells, and seed coat cells.Citation45,Citation49 However, tag-induced side effects have not been described to date. In this study, we report the effect of amino-terminal EYFP fusions on the stability of two HD-Zip IV transcription factors, GL2 and PDF2, along with an abnormal curly leaf phenotype associated with elevated expression of EYFP-tagged GL2.
Results
EYFP fusions to HD-Zip IV TFs enhance their protein stability
EYFP is a GFP variant comprising of S65G, V68L, S72A, and T203Y substitutions relative to wild-type GFP.Citation10,Citation50 Its excitation and emission peaks at 514 nm and 527 nm, respectively, are associated with stacking of Tyr66 with Tyr203.Citation10,Citation51 Here, we used transgenic Arabidopsis lines expressing amino-terminal fusions of EYFP to the HD-Zip IV transcription factors GL2 and PDF2 in comparison to amino-terminal fusions using 3xFLAG or HA tags (). The FLAG tag, also known as the DYKDDDDK-tag, is a short, hydrophilic epitope tag of ~1.013 kDaCitation52 that is commonly implemented in a tandem configuration as the 3xFLAG peptide.Citation53 Similarly, the HA tag is a ~ 1.102 kDa peptide corresponding to residues 98–106 of human influenza virus hemagglutinin (HA).Citation54 For the EYFP and 3xFLAG tags, the fusion proteins were expressed under the GL2 native promoter, whereas the cauliflower mosaic virus (CaMV) 35S promoter was used to drive the HA-tag fusions.
Figure 1. EYFP fusions to HD-Zip IV TFs enhance their protein stability. (a) Schematic of constructs (drawn to scale) used in this study including EYFP-, FLAG- and HA-tagged GL2 and EYFP- and HA-tagged PDF2. EYFP- and FLAG-tagged proteins were expressed under the GL2 native promoter while HA-tagged proteins were expressed under the CaMV 35S promoter. (b) Flow diagram of the in vivo CHX chase experiment used to monitor protein turnover. (c-f) Blot images and corresponding data quantification from CHX experiments for GL2 (c, d) and PDF2 (e, f) proteins. M0, M10 and M24, Mock treatments for 0, 10 and 24 h; Loading, RuBisCo bands from Coomassie Blue stained total protein. Means are shown for n = 3 biological replicates, and error bars signify standard deviations (SDs). Unpaired t-test or ordinary two-way ANOVA using Tukey’s multiple comparisons test was applied to test the significance of the difference between protein levels (*p < .05, **p < .005).
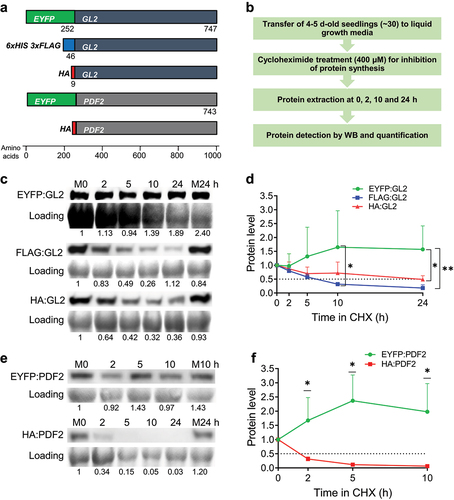
To determine the effect of EYFP fusions on protein stability, we performed Western blot (WB) analysis of protein samples taken at various time points after treatment of Arabidopsis seedlings with cycloheximide (CHX) (). CHX is an inhibitor of eukaryotic translational elongation that is routinely used to study protein turnover in the absence of translation. Quantification of blot images showed that while the protein levels of GL2 fused to the smaller tags, 3xFLAG and HA, dropped over time with half-lives of ~6.5 and ~22 h respectively, EYFP:GL2 remained stable throughout the 24 h time course (). A more dramatic difference was observed between the half-lives of EYFP- and HA-tagged PDF2. The EYFP:PDF2 protein remained significantly more stable compared to HA:PDF2, which exhibited hardly detectable protein levels after 2 h (). These data indicate that the amino-terminal fusion to EYFP confers stability to HD-Zip IV TFs in plants.
Expression of EYFP:GL2 results in downward leaf curling in Arabidopsis
Previous studies revealed the role of GL2 in the differentiation of leaf epidermal cells, especially in the formation of trichomes,Citation32,Citation33 but GL2 has not been implicated in leaf polarity establishment to date. In our study, screening T1 transformants for the rescue of null phenotypes indicated complete rescue of trichome defects in 30.95% (n = 42) and 54.05% (n = 37) of EYFP:GL2 and FLAG:GL2 transformants, respectively (). The EYFP:GL2 lines exhibited a range of EYFP expression including strong (17.07%), medium (31.71%), weak (21.95%) and no (29.27%) expression (). Strikingly, 9.76% of EYFP:GL2 plants displayed abnormal curling of leaves, a phenotype that was absent in FLAG:GL2 lines (). Confocal laser scanning microscopy confirmed the nuclear localization of the EYFP:GL2 protein in the primary root of Arabidopsis seedlings () indicating that fusing EYFP at the amino-terminus of GL2 does not alter its subcellular localization, consistent with previous reports.Citation45,Citation49 Taken together, our observations led us to hypothesize that the elevated protein stability of the EYFP:GL2 protein causes abnormal leaf curling during development.
Figure 2. Expression of EYFP:GL2 leads to development of abaxially curled leaves in Arabidopsis. Graphs showing (a) rescue of trichome phenotype, (b) EYFP expression and (c) occurrence of curly leaves in T1 progeny of plants stably transformed with the indicated construct. (d) Confocal laser scanning microscopy indicating the nuclear localization of EYFP:GL2 in the primary root of a 4-d-old seedling stained with propidium iodide. Size bar = 20 μm. (e) Rosettes of 15-d-old wild type (Col), gl2-5, EYFP:GL2 and FLAG:GL2 plants. Size bar = 2 mm. (f) 25-d-old EYFP:GL2 plants with two types of leaves. Size bar = 2 cm. (g) Expression of EYFP:GL2 in rosettes and leaf trichomes of 15-d-old plants. Size bar = 500 µm. (h) Blot images and (i) corresponding quantification data of EYFP:GL2 expression in the leaves of 25-d-old plants. Numerical values indicating EYFP:GL2 protein levels are represented as mean ± SD (n = 6 biological replicates). Unpaired t-test (two-tailed) was applied to test the significance of the difference between protein levels (****p < .0001).
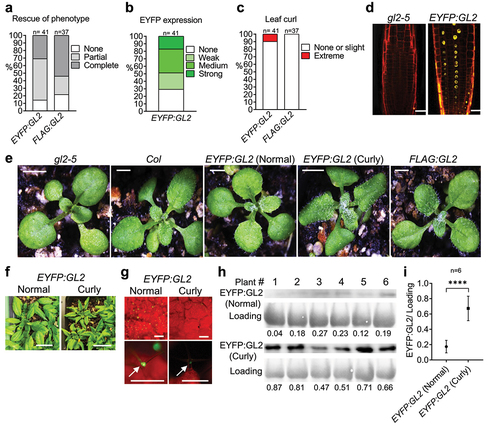
The curly leaves in EYFP:GL2 plants display a narrow and pointed morphology associated with downward rolling and altered adaxial-abaxial leaf polarity (). The leaf curling phenotype largely contributed to the distinct appearance of EYFP:GL2 rosettes in comparison to FLAG:GL2, gl2-5 null mutant or Col wild-type lines (). The onset of this phenotype occurred at around day 12–13 after germination, with rolling occurring in either the longitudinal or transverse direction (). However, longitudinal curling became predominant later in development and the intensity of leaf curling increased at the stages leading up to flowering (). We next asked whether the EYFP:GL2 protein expression level is altered in curly leaf plants in comparison to normal leaf plants. Under an epifluorescence stereomicroscope, fluorescent signals in leaf trichome nuclei of curly leaf plants appeared much stronger compared to those of normal leaf plants (). To confirm this, WB analysis was performed with leaves from 25-d-old curly leaf and normal leaf plants expressing EYFP:GL2 (). Quantification of protein bands revealed significantly higher expression of EYFP:GL2 in curly leaves compared to normal/flat leaves ().
Figure 3. Quantitative characterization of curly leaf plants in comparison to normal/flat leaf plants. (a) The phenotypes of adaxial (left) and abaxial (right) faces, and (b) leaf blade length and width statistics of the 6th leaves of 25-d-old plants. (c) Degree of leaf 6 epinasty in 25-d-old plants of various genotypes. (d) Images of 45-d-old EYFP:GL2 plants with two types of leaves and (e) corresponding graph indicating their heights. (f) Time of onset of flowering in EYFP:GL2 plants bearing normal and curly leaves. (g) Images of 4-d-old seedlings of various genotypes and (h) graph showing their lengths. Size bar = 5 mm. For all measurements, means are shown for n = 15 plants, and error bars indicate SDs. Depending upon the number of variables, ordinary one- or two-way ANOVA using Tukey’s multiple comparisons test was used to establish significant differences between genotypes (*p < .05, ****p < .0001).
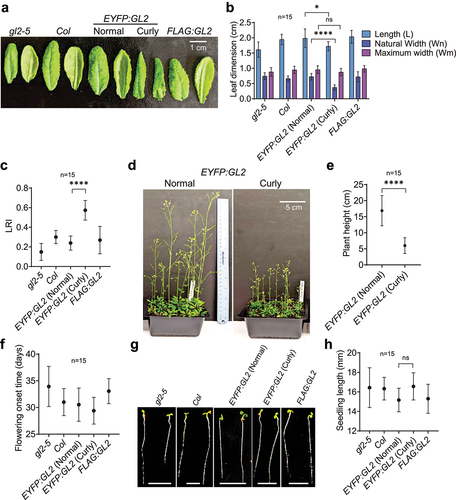
Characterization of the curly leaf trait associated with EYFP:GL2 expression
Leaf size and shape play important roles in overall plant architecture and physiology.Citation55,56,Citation56 Appropriate leaf rolling is of agronomic value as it is associated with erect leaf canopies, higher photosynthetic efficiency, optimum canopy temperature, and protection against UV light.Citation57–59 Leaf rolling is also critical for adaptation to drought as it affects osmotic adjustment and reduces transpirational water loss.Citation60–62 To quantify the effect of EYFP:GL2 expression on the three-dimensional architecture of leaves, we measured the distances between the leaf edges halfway from the leaf blades in curly leaf versus normal/flat plant lines. Measurement of the leaf dimensions revealed significantly reduced leaf blade width in curly leaf lines compared to the normal/flat leaf lines although both displayed similar leaf lengths ()). In accordance, EYFP:GL2 plants exhibited the highest values for the rolling leaf index (LRI) or degree of epinasty, followed by Col wild type, FLAG:GL2 and gl2-5 null mutant plants (). These quantitative data confirm our original observations that the leaf blade edges curl downward to a greater extent in EYFP:GL2 curly leaf lines (). The curly leaf plants also exhibited short stature with somewhat stunted growth () and slightly early flowering () compared to plants with normal leaf morphology. In contrast, there were no significant differences in the lengths of the hypocotyl and root in young seedlings ().
Discussion
In this communication, we quantified the stability of HD-Zip IV transcription factors fused to various tags and reported the phenotypic changes in Arabidopsis lines expressing highly stable EYFP:GL2 fusion proteins. There are several possible reasons behind the altered stability of fluorescently tagged HD-Zip IV proteins. Addition of the EYFP tag could potentially change protein conformation, interfere with binding partners or impact posttranslational modifications (PTMs) such as phosphorylation or ubiquitination, altering the extent of protein activation or degradation. In a related study using mammalian cells, expression of EGFP inhibited ubiquitination by the RING-type E3 ubiquitin ligases TRAF6 and API2, resulting in impaired NF-kB and JNK signaling and enhanced stability of the tumor suppressor p53.Citation63 Intriguingly, it was found that EGFP contains a consensus motif (PNEKRD) implicated in TRAF6 binding.Citation63 In another study, the expression of EGFP impaired actin-myosin interactions and thus contractile properties of cardiac muscle cells by competitive binding to the actin-binding site of myosin.Citation64,Citation65 Proteins also have targeting signals and sites for PTMs such as palmitoylation, myristylation and farnesylationCitation66–68 which are reported to be obscured by fusion to fluorescent tags.Citation68,Citation69 Although the EYFP:GL2 protein is functional in terms of its ability to rescue gl2 null mutants, amino-terminal tagging may nonetheless cause subtle changes in GL2 trafficking or turnover. Fusion to the EYFP tag could additionally disrupt another aspect of GL2 function when it is overexpressed, such as slightly altering its DNA-binding specificity, thus causing misexpression of target genes responsible for leaf curling.
In a previous study, it was reported that ectopic expression of GL2 using the CaMV 35S promoter disrupts the endogenous function of GL2 and fails to rescue the gl2-1 mutant.Citation70 In the same study, additive expression of GL2 under its native promoter in the wild-type genetic background resulted in an increase in trichome initiation along with altered spacing of trichomes.Citation70 However, a curly leaf phenotype was not reported in either scenario that was associated with overexpression of untagged GL2. Therefore, the occurrence of leaf curl in our study seems to be attributed to the EYFP component of the fusion protein. Furthermore, the data presented here show that the more pronounced abaxial leaf curl or epinasty of leaf blade edges in a subset of EYFP:GL2 lines was associated with the enhanced protein level and stability of GL2 (). Not all plants expressing EYFP:GL2 displayed this phenotype () because each transformation event represents a random insertion of the transgene into the genome and positions of the insertion and/or tandem insertions can impact expression levels (). Leaf curling is usually associated with polarity changes in the leaf abaxial–adaxial axis.Citation71,Citation72 A complex regulatory network consisting of various transcription factors including HD-Zip III,Citation73–76 YABBYCitation77–79 and KANADI (KAN),Citation80–84 small non-coding/microRNAsCitation76,Citation85,Citation86 and components of hormonal signaling including the Auxin/Indole-3-Acetic Acid (Aux/IAA) gene family and the redundantly acting AUXIN RESPONSE FACTOR (ARF) genes ETTIN (ETT)/ARF3 ARF4Citation57,Citation87–89 are known to control establishment of leaf polarity. Auxin biosynthesis, polar transport, and signal transduction are important for plant growth and developmentCitation90,Citation91 and defects in these processes can lead to leaf curling and dwarf/semi-dwarf phenotypes.Citation92–94 Since we observed dwarf stature and reduced plant growth in association with leaf curling in plants expressing EYFP:GL2 (), it is possible that GL2 overexpression exerts its effect through auxin signaling or another hormone pathway.
A few HD-Zip IV genes were implicated in leaf curling previously. In one study, knockout of Rice Outermost Cell-Specific 5 (ROC5), an ortholog of GL2, resulted in abaxial leaf rolling because of abnormal bulliform cell formation in the adaxial leaf blade.Citation95 Vice versa, overexpression of ROC5 suppressed the development of bulliform cells giving rise to adaxially rolled leaves in rice.Citation95 However, we observed the opposite effect with GL2 overexpression in Arabidopsis (). A more recent study showed that HD-Zip IV member ROC8 heterodimerizes with ROC5 in transcriptional repression of Abaxially Curled Leaf 1 (ACL1), a positive regulator of bulliform cell development.Citation96 Overexpression of GL2 seems to induce leaf curling by a different mechanism since bulliform cells are specific to monocots. One approach to identify the leaf polarity determinants affected by EYFP:GL2 expression might be transcriptomic analysis of differentially expressed genes in curly versus normal/flat leaves. Similarly, mRNA in situ hybridization could be used to identify tissue-specific or polarized distribution of specific transcripts that regulate leaf polarity.Citation89,Citation97,Citation98 Since changes in epidermal cell shape, size or arrangement may be associated with leaf curling,Citation79 anatomical examination of leaves with curly leaf morphologies will be useful in future studies.
Although the use of fluorescent tags is critical for specific experiments, it is often helpful to use alternative tags of different sizes and/or properties to rule out any tag-specific observations. If feasible, using specific antibodies against the proteins of interest will also aid in resolving tag-related issues, e.g., to determine whether the fusion proteins are overexpressed relative to their endogenous counterparts. The position of the tag may also influence protein activity. While our analysis was restricted to amino-terminal fusions, it would be interesting to see whether attaching EYFP to the carboxyl-terminus or inserting it internally between domains affects GL2 function and/or leaf curling. Nonetheless, chances of affecting protein structural features or disrupting interactions with a ligand or other proteins are high if the site of insertion is not selected properly. In summary, being aware of the limitations of fluorescent tags and available alternatives is important when generating fusion proteins in research studies.
Materials and methods
Plant materials and growth conditions
The wild-type (WT) Arabidopsis thaliana ecotype Columbia (Col), gl2-5 null mutantCitation99 and transgenic plants expressing EYFP or FLAG-tagged GL2 were grown on soil containing Berger BM6, vermiculite and perlite (Hummert International) in a ratio of 4:2:1 at 23°C under continuous light.
Generation of transgenic lines
The proGL2:EYFP:GL2 construct was generated using SR54 binary vector as previously described.Citation45 For FLAG tagging, the proGL2:EYFP:GL2 construct was restriction digested with BamHI and SalI to remove the EYFP tag and the 6xHis 3xFLAG tag from pB7HFC-GFPCitation100 was added to amino-terminus of GL2 using NEBuilder HiFi DNA Assembly (New England Biolabs, E5520S). Construction of HA:GL2, EYFP:PDF2 and HA:PDF2 is described elsewhere.Citation101 The binary vector constructs were used to transform gl2-5 plants by Agrobacterium tumefaciens (GV3101) mediated floral dip.Citation102 Transgenic lines were screened in presence of 20 μg/ml Hygromycin B. For each genotype, 37–42 independent T1 transformants were characterized for rescue of the trichome phenotype, EYFP expression and leaf curling. T2 progeny were analyzed for 3:1 segregation of the transgene. T3 homozygous lines confirmed by PCR genotyping and sequencing were used for phenotypic analysis and further experiments.
In vivo protein stability assay
Seeds from EYFP-, FLAG- and HA-tagged GL2 or PDF2 lines were vapor sterilized, sown on 0.5X MS agar,Citation103 stratified at 4°C for 4–5 days, and germinated in vertical orientation at 23°C under continuous light. After 4 days, samples of ~30 seedlings each were transferred to 24-well plates containing 1 ml 0.5X liquid MS media, followed by the incubation on a shaker at 60 rpm for ~16 h under continuous light at 23°C. Next, cycloheximide (Sigma-Aldrich, C1988) (400 µM in DMSO) or DMSO control was added to the wells. Seedling samples were harvested in screw-cap microcentrifuge tubes at the designated times by immediate freezing in liquid nitrogen followed by storage at −80°C until further use. Proteins were extracted from the seedlings by adding Laemmli sample buffer at 95°C after grinding in liquid nitrogen. For Western blotting, samples were separated on Bio-Rad Mini-PROTEAN TGX gels (10%), transferred to PVDF membranes and incubated with primary antibodies against GFP (1:2000; Roche, 11814460001), FLAG (1:10,000; Sigma, F1804) or HA (1:10,000; Pierce, 26183) followed by goat anti-mouse secondary IgG HRP antibodies (1:3300; GenScript, A00160). Proteins were detected with SuperSignal West Femto Maximum Sensitivity Substrate (ThermoFisher Scientific) using an Azure 300 chemiluminescence imager (Azure Biosystems). The membranes were stained with Bio-Safe Coomassie Blue G-250 (Bio-Rad) for loading controls, and band intensities were quantified with ImageJ software.
Phenotypic assays, leaf biometry, and microscopy
Rosettes and trichomes of ~15-d-old plants and EYFP expression were imaged with a Leica M165 fluorescence stereomicroscope fitted with a GFP2 filter set and a Leica DFC295 digital camera. To measure seedling lengths, sterile seeds were sown on 0.8% agar media, stratified at 4°C for 5 days and the plates were placed vertically at 23°C under continuous light for 4 days. The leaf blade lengths and widths were measured on the 6th leavesCitation104 of 25-d-old plants of each genotype. Digital images of seedlings, whole plants and leaves were captured using a Google Pixel 3XL (12MP f/1.8) camera. To determine the subcellular localization of EYFP-tagged proteins, the primary roots of 4-d-old seedlings stained with propidium iodide (10 μg/ml) were imaged with a Zeiss LSM 880 confocal microscope. For acquiring EYFP signals, a 488 nm Argon laser and a 500–550 nm emission filter was used. For propidium iodide, a 561 nm DPSS laser in combination with a 575–645 nm emission filter was used.
Leaf Rolling Index (LRIs)/ Degree of epinasty was calculated following the protocol described inCitation105 and.Citation57
LRI = (Wm − Wn)/Wm × 100, where Wm is the greatest width of the leaf blade in its expanded state and Wn is the natural distance of the leaf blade margins at the same site.
Interpretation of LRI values:
0 = Flat leaf blade (no curling)
+ve = Epinastic leaf blade (downward curling)
-ve = Hyponastic leaf blade (upcurling)
Author contributions
KS and BS conceived of and designed the experiments. BS performed the experiments and analyzed the data. BS wrote the manuscript and KS revised the manuscript.
Acknowledgments
The authors thank Joel Sanneman (Kansas State University) for help with confocal microscopy and Maria Sorkin (Danforth Plant Science Center) for providing the pB7HFC-GFP construct. This is contribution no. 23-001-J from the Kansas Agricultural Experiment Station.
Disclosure statement
No potential conflict of interest was reported by the authors.
Additional information
Funding
References
- Prasher DC, Eckenrode VK, Ward WW, Prendergast FG, Cormier MJ. Primary structure of the Aequorea victoria green-fluorescent protein. Gene. 1992;111(2):229–10. doi:10.1016/0378-1119(92)90691-H.
- Shimomura O, Johnson FH, Saiga Y. Extraction, purification and properties of aequorin, a bioluminescent protein from the luminous hydromedusan. Aequorea J Cell Comp Physiol. 1962;59(3):223–239. doi:10.1002/jcp.1030590302.
- Blatt MR, Grefen C. Applications of fluorescent marker proteins in plant cell biology. Methods Mol Biol. 2014;1062:487–507.
- Chalfie M, Tu Y, Euskirchen G, Ward WW, Prasher DC. Green fluorescent protein as a marker for gene expression. Science. 1994;263(5148):802–805. doi:10.1126/science.8303295.
- Leffel SM, Mabon SA, Stewart CNsJr. Applications of green fluorescent protein in plants. Biotechniques. 1997;23(5):912–918. doi:10.2144/97235bi01.
- Chudakov DM, Matz MV, Lukyanov S, Lukyanov KA. Fluorescent proteins and their applications in imaging living cells and tissues. Physiol Rev. 2010;90(3):1103–1163. doi:10.1152/physrev.00038.2009.
- Heim R, Prasher DC, Tsien RY. Wavelength mutations and posttranslational autoxidation of green fluorescent protein. Proc Natl Acad Sci U S A. 1994;91(26):12501–12504. doi:10.1073/pnas.91.26.12501.
- Kain SR, AdamsM, Kondepudi A, Yang TT, Ward WW, Kitts P. Green fluorescent protein as a reporter of gene expression and protein localization. Bio Techniques. 1995;19(4):650–655.
- Sheen J, Hwang S, Niwa Y, Kobayashi H, Galbraith DW. Green-fluorescent protein as a new vital marker in plant cells. Plant J. 1995;8(5):777–784. doi:10.1046/j.1365-313X.1995.08050777.x.
- Ormö M, Cubitt AB, Kallio K, Gross LA, Tsien RY, Remington SJ. Crystal structure of the Aequorea victoria green fluorescent protein. Science. 1996;273(5280):1392–1395. doi:10.1126/science.273.5280.1392.
- Yang F, Moss LG, Phillips GNsJr. The molecular structure of green fluorescent protein. Nat Biotechnol. 1996;14(10):1246–1251. doi:10.1038/nbt1096-1246.
- Lisenbee CS, Karnik SK, Trelease RN. Overexpression and mislocalization of a tail-anchored GFP redefines the identity of peroxisomal ER. Traffic. 2003;4(7):491–501. doi:10.1034/j.1600-0854.2003.00107.x.
- Patterson GH, Knobel SM, Sharif WD, Kain SR, Piston DW. Use of the green fluorescent protein and its mutants in quantitative fluorescence microscopy. Biophys J. 1997;73(5):2782–2790. doi:10.1016/S0006-3495(97)78307-3.
- Surribas A, Resina D, Ferrer P, Valero F. Limitations using GFP as a protein expression reporter in Pichia pastoris. Microb Cell Fact. 2006;5(1):56. doi:10.1186/1475-2859-5-S1-P56.
- Costantini LM, Fossati M, Francolini M, Snapp EL. Assessing the tendency of fluorescent proteins to oligomerize under physiologic conditions. Traffic. 2012;13(5):643–649. doi:10.1111/j.1600-0854.2012.01336.x.
- Cranfill PJ, Sell BR, Baird MA, Allen JR, Lavagnino Z, de Gruiter HM, Kremers G-J, Davidson MW, Ustione A, Piston DW, et al. Quantitative assessment of fluorescent proteins. Nat Methods. 2016;13(7):557–562. doi:10.1038/nmeth.3891.
- Botman D, de Groot DH, Schmidt P, Goedhart J, Teusink B. In vivo characterisation of fluorescent proteins in budding yeast. Sci Rep. 2019;9(1):2234. doi:10.1038/s41598-019-38913-z.
- Lenassi Zupan AL, Trobec, S, Gaberc-Porekar, V, Menart, V. High expression of green fluorescent protein in Pichia pastoris leads to formation of fluorescent particles. J Biotechnol. 2004;109(1–2):115–122. doi:10.1016/j.jbiotec.2003.11.013.
- Hanazono Y, Yu J-M, Dunbar CE, Emmons RVB. Green fluorescent protein retroviral vectors: low titer and high recombination frequency suggest a selective disadvantage. Hum Gene Ther. 1997;8(11):1313–1319. doi:10.1089/hum.1997.8.11-1313.
- Liu HS, Jan M-S, Chou C-K, Chen P-H, Ke N-J. Is green fluorescent protein toxic to the living cells? Biochem Biophys Res Commun. 1999;260(3):712–717. doi:10.1006/bbrc.1999.0954.
- Krestel HE, Mihaljevic ALA, Hoffman DA, Schneider A. Neuronal co-expression of EGFP and beta-galactosidase in mice causes neuropathology and premature death. Neurobiol Dis. 2004;17(2):310–318. doi:10.1016/j.nbd.2004.05.012.
- Huang W-Y, Aramburu J, Douglas PS, Izumo S. Transgenic expression of green fluorescence protein can cause dilated cardiomyopathy. Nat Med. 2000;6(5):482–483. doi:10.1038/74914.
- Zhang F, Hackett NR, Lam G, Cheng J, Pergolizzi R, Luo L, Shmelkov SV, Edelberg J, Crystal RG, Rafii S, et al. Green fluorescent protein selectively induces HSP70-mediated up-regulation of COX-2 expression in endothelial cells. Blood. 2003;102(6):2115–2121. doi:10.1182/blood-2003-01-0049.
- Thomas CL, Maule AJ. Limitations on the use of fused green fluorescent protein to investigate structure-function relationships for the cauliflower mosaic virus movement protein. J Gen Virol. 2000;81(Pt 7):1851–1855. doi:10.1099/0022-1317-81-7-1851.
- Luesse DR, DeBlasio SL, Hangarter RP. Plastid movement impaired 2, a new gene involved in normal blue-light-induced chloroplast movements in Arabidopsis. Plant Physiol. 2006;141(4):1328–1337. doi:10.1104/pp.106.080333.
- Ketelaar T, Anthony RG, Hussey PJ. Green fluorescent protein-mTalin causes defects in actin organization and cell expansion in Arabidopsis and inhibits actin depolymerizing factor’s actin depolymerizing activity in vitro. Plant Physiol. 2004;136(4):3990–3998. doi:10.1104/pp.104.050799.
- Javelle M, Klein-Cosson C, Vernoud V, Boltz V, Maher C, Timmermans M, Depège-Fargeix N, Rogowsky PM. Genome-wide characterization of the HD-ZIP IV transcription factor family in maize: preferential expression in the epidermis. Plant Physiol. 2011;157(2):790–803. doi:10.1104/pp.111.182147.
- Nakamura M, Katsumata H, Abe M, Yabe N, Komeda Y, Yamamoto KT, Takahashi T. Characterization of the class IV homeodomain-leucine zipper gene family in Arabidopsis. Plant Physiol. 2006;141(4):1363–1375. doi:10.1104/pp.106.077388.
- Wu R, Li S, He S, Waßmann F, Yu C, Qin G, Schreiber L, Qu L-J, Gu H. CFL1, a WW domain protein, regulates cuticle development by modulating the function of HDG1, a class IV homeodomain transcription factor, in rice and Arabidopsis. Plant Cell. 2011;23(9):3392–3411. doi:10.1105/tpc.111.088625.
- Yu H, Chen X, Hong -Y-Y, Wang Y, Xu P, Ke S-D, Liu H-Y, Zhu J-K, Oliver DJ, Xiang C-B, et al. Activated expression of an Arabidopsis HD-START protein confers drought tolerance with improved root system and reduced stomatal density. Plant Cell. 2008;20(4):1134–1151. doi:10.1105/tpc.108.058263.
- Chew W, Hrmova M, Lopato S. Role of Homeodomain leucine zipper (HD-Zip) IV transcription factors in plant development and plant protection from deleterious environmental factors. Int J Mol Sci. 2013;14(4):8122–8147. doi:10.3390/ijms14048122.
- Rerie WG, Feldmann KA, Marks MD. The GLABRA2 gene encodes a homeo domain protein required for normal trichome development in Arabidopsis. Genes Dev. 1994;8(12):1388–1399. doi:10.1101/gad.8.12.1388.
- Szymanski DB, Jilk RA, Pollock SM, Marks MD. Control of GL2 expression in Arabidopsis leaves and trichomes. Development. 1998;125(7):1161–1171. doi:10.1242/dev.125.7.1161.
- Hung CY, Lin Y, Zhang M, Pollock S, Marks MD, Schiefelbein J. A common position-dependent mechanism controls cell-type patterning and GLABRA2 regulation in the root and hypocotyl epidermis of Arabidopsis. Plant Physiol. 1998;117(1):73–84. doi:10.1104/pp.117.1.73.
- Lin Y, Schiefelbein J. Embryonic control of epidermal cell patterning in the root and hypocotyl of Arabidopsis. Development. 2001;128(19):3697–3705. doi:10.1242/dev.128.19.3697.
- Masucci JD, Rerie WG, Foreman DR, Zhang M, Galway ME, Marks MD, Schiefelbein JW. The homeobox gene GLABRA2 is required for position-dependent cell differentiation in the root epidermis of Arabidopsis thaliana. Development. 1996;122(4):1253–1260. doi:10.1242/dev.122.4.1253.
- Di Cristina M, Sessa G, Dolan L, Linstead P, Baima S, Ruberti I, Morelli G. The Arabidopsis Athb-10 (GLABRA2) is an HD-Zip protein required for regulation of root hair development. Plant J. 1996;10(3):393–402. doi:10.1046/j.1365-313X.1996.10030393.x.
- Western TL, Burn J, Tan WL, Skinner DJ, Martin-McCaffrey L, Moffatt BA, Haughn GW. Isolation and characterization of mutants defective in seed coat mucilage secretory cell development in Arabidopsis. Plant Physiol. 2001;127(3):998–1011. doi:10.1104/pp.010410.
- Western TL, Young DS, Dean GH, Tan WL, Samuels AL, Haughn GW. MUCILAGE-MODIFIED4 encodes a putative pectin biosynthetic enzyme developmentally regulated by APETALA2, TRANSPARENT TESTA GLABRA1, and GLABRA2 in the Arabidopsis seed coat. Plant Physiol. 2004;134(1):296–306. doi:10.1104/pp.103.035519.
- Abe M, Katsumata H, Komeda Y, Takahashi T. Regulation of shoot epidermal cell differentiation by a pair of homeodomain proteins in Arabidopsis. Development. 2003;130(4):635–643. doi:10.1242/dev.00292.
- Ogawa E, Yamada Y, Sezaki N, Kosaka S, Kondo H, Kamata N, Abe M, Komeda Y, Takahashi T. ATML1 and PDF2 play a redundant and essential role in Arabidopsis embryo development. Plant Cell Physiol. 2015;56(6):1183–1192. doi:10.1093/pcp/pcv045.
- Abe M, Takahashi T, Komeda Y. Identification of a cis-regulatory element for L1 layer-specific gene expression, which is targeted by an L1-specific homeodomain protein. Plant J. 2001;26(5):487–494. doi:10.1046/j.1365-313x.2001.01047.x.
- Rombolá-Caldentey B, Rueda-Romero P, Iglesias-Fernández R, Carbonero P, Oñate-Sánchez L. Arabidopsis DELLA and two HD-ZIP transcription factors regulate GA signaling in the epidermis through the L1 box cis -element. Plant Cell. 2014;26(7):2905. doi:10.1105/tpc.114.127647.
- Takada S, Jürgens G. Transcriptional regulation of epidermal cell fate in the Arabidopsis embryo. Development. 2007;134(6):1141–1150. doi:10.1242/dev.02803.
- Schrick K, Bruno M, Khosla A, Cox PN, Marlatt SA, Roque RA, Nguyen HC, He C, Snyder MP, Singh D, et al. Shared functions of plant and mammalian StAR-related lipid transfer (START) domains in modulating transcription factor activity. BMC Biol. 2014;12(1):70. doi:10.1186/s12915-014-0070-8.
- Lin Q, Ohashi Y, Kato M, Tsuge T, Gu H, Qu L-J, Aoyama T. GLABRA2 directly suppresses basic helix-loop-helix transcription factor genes with diverse functions in root hair development. Plant Cell. 2015;27(10):2894. doi:10.1105/tpc.15.00607.
- Nagata K, Ishikawa T, Kawai-Yamada M, Takahashi T, Abe M. Ceramides mediate positional signals in Arabidopsis thaliana protoderm differentiation. Development. 2021;148(2). doi:10.1242/dev.194969.
- Iida H, Yoshida A, Takada S. ATML1 activity is restricted to the outermost cells of the embryo through post-transcriptional repressions. Development. 2019;146(4). doi:10.1242/dev.169300.
- Mukherjee T, Subedi B, Khosla A, Begler EM, Stephens PM, Warner AL, Lerma-Reyes R, Thompson KA, Gunewardena S, Schrick K . START domain mediates Arabidopsis GLABRA2 dimerization and turnover independently of homeodomain DNA binding. Plant Physiol, 2022. doi:10.1093/plphys/kiac383.
- Cubitt AB, Woollenweber LA, Heim R. Methods Cell Biol 58 1999. 19–30 doi:10.1016/s0091-679x(08)61946-9.
- Rizzo MA, Springer GH, Granada B, Piston DW. An improved cyan fluorescent protein variant useful for FRET. Nat Biotechnol. 2004;22(4):445–449. doi:10.1038/nbt945.
- Hopp TP, Prickett KS, Price VL, Libby RT, March CJ, Pat Cerretti D, Urdal DL, Conlon PJ. A short polypeptide marker sequence useful for recombinant protein identification and purification. Bio Technol. 1988;6(10):1204–1210. doi:10.1038/nbt1088-1204.
- Ueda M, Manabe Y, Mukai M. The high performance of 3XFLAG for target purification of a bioactive metabolite: a tag combined with a highly effective linker structure. Bioorg Med Chem Lett. 2011;21(5):1359–1362. doi:10.1016/j.bmcl.2011.01.038.
- Wilson IA, Niman HL, Houghten RA, Cherenson AR, Connolly ML, Lerner RA. The structure of an antigenic determinant in a protein. Cell. 1984;37(3):767–778. doi:10.1016/0092-8674(84)90412-4.
- Govaerts YM, Jacquemoud S, Verstraete MM, Ustin SL. Three-dimensional radiation transfer modeling in a dicotyledon leaf. Appl Opt. 1996;35(33):6585–6598. doi:10.1364/AO.35.006585.
- Moon J, Hake S. How a leaf gets its shape. Curr Opin Plant Biol. 2011;14(1):24–30. doi:10.1016/j.pbi.2010.08.012.
- Fierro AC, Leroux O, De Coninck B, Cammue BPA, Marchal K, Prinsen E, Van Der Straeten D, Vandenbussche F. Ultraviolet-B radiation stimulates downward leaf curling in Arabidopsis thaliana. Plant Physiol Biochem. 2015;93:9–17. doi:10.1016/j.plaphy.2014.12.012.
- Saglam A, Kadioglu A, Demiralay M, Terzi R. Leaf rolling reduces photosynthetic loss in maize under severe drought. Acta Botanica Croatica. 2014;73(2). doi:10.2478/botcro-2014-0012.
- Turner NC, O’Toole JC, Cruz RT, Namuco OS, Ahmad S. Responses of seven diverse rice cultivars to water deficits I. stress development, canopy temperature, leaf rolling and growth. Field Crops Res. 1986;13:257–271. doi:10.1016/0378-4290(86)90027-4.
- Hsiao TC, O’Toole JC, Yambao EB, Turner NC. Influence of osmotic adjustment on leaf rolling and tissue death in rice (Oryza sativa L.). Plant Physiol. 1984;75(2):338–341. doi:10.1104/pp.75.2.338.
- O’Toole JC, Cruz RT. Response of leaf water potential, stomatal resistance, and leaf rolling to water stress. Plant Physiol. 1980;65(3):428–432. doi:10.1104/pp.65.3.428.
- O’Toole JC, Cruz RT, Singh TN. Leaf rolling and transpiration. Plant Sci Lett. 1979;16(1):111–114. doi:10.1016/0304-4211(79)90015-4.
- Baens M, Noels H, Broeckx V, Hagens S, Fevery S, Billiau AD, Vankelecom H, Marynen P. The dark side of EGFP: defective polyubiquitination. PLoS One. 2006;1(1):e54. doi:10.1371/journal.pone.0000054.
- Agbulut O, Coirault C, Niederländer N, Huet A, Vicart P, Hagège A, Puceat M, Menasché P. GFP expression in muscle cells impairs actin-myosin interactions: implications for cell therapy. Nat Methods. 2006;3(5):331. doi:10.1038/nmeth0506-331.
- Agbulut O, Huet A, Niederländer N, Puceat M, Menasché P, Coirault C. Green fluorescent protein impairs actin-myosin interactions by binding to the actin-binding site of myosin. J Biol Chem. 2007;282(14):10465–10471. doi:10.1074/jbc.M610418200.
- Hughes TE, Zhang H, Logothetis DE, Berlot CH. Visualization of a functional Galpha q-green fluorescent protein fusion in living cells. association with the plasma membrane is disrupted by mutational activation and by elimination of palmitoylation sites, but not be activation mediated by receptors or AlF4. J Biol Chem. 2001;276(6):4227–4235. doi:10.1074/jbc.M007608200.
- Martoglio B, Dobberstein B. Signal sequences: more than just greasy peptides. Trends Cell Biol. 1998;8(10):410–415. doi:10.1016/S0962-8924(98)01360-9.
- Tian G-W, Mohanty A, Chary SN, Li S, Paap B, Drakakaki G, Kopec CD, Li J, Ehrhardt D, Jackson D, et al. High-throughput fluorescent tagging of full-length Arabidopsis gene products in planta. Plant Physiol. 2004;135(1):25–38. doi:10.1104/pp.104.040139.
- DeBlasio SL, Sylvester AW, Jackson D. Illuminating plant biology: using fluorescent proteins for high-throughput analysis of protein localization and function in plants. Brief Funct Genomics. 2010;9(2):129–138. doi:10.1093/bfgp/elp060.
- Ohashi Y, Oka A, Ruberti I, Morelli G, Aoyama T. Entopically additive expression of GLABRA2 alters the frequency and spacing of trichome initiation. Plant J. 2002;29(3):359–369. doi:10.1046/j.0960-7412.2001.01214.x.
- McConnell JR, Barton MK. Leaf polarity and meristem formation in Arabidopsis. Development. 1998;125(15):2935–2942. doi:10.1242/dev.125.15.2935.
- Byrne ME. Networks in leaf development. Curr Opin Plant Biol. 2005;8(1):59–66. doi:10.1016/j.pbi.2004.11.009.
- Emery JF, Floyd SK, Alvarez J, Eshed Y, Hawker NP, Izhaki A, Baum SF, Bowman JL. Radial patterning of Arabidopsis shoots by class III HD-ZIP and KANADI genes. Curr Biol. 2003;13(20):1768–1774. doi:10.1016/j.cub.2003.09.035.
- McConnell JR, Emery J, Eshed Y, Bao N, Bowman J, Barton MK. Role of PHABULOSA and PHAVOLUTA in determining radial patterning in shoots. Nature. 2001;411(6838):709–713. doi:10.1038/35079635.
- Prigge MJ, Otsuga D, Alonso JM, Ecker JR, Drews GN, Clark SE. Class III homeodomain-leucine zipper gene family members have overlapping, antagonistic, and distinct roles in Arabidopsis development. Plant Cell. 2005;17(1):61–76. doi:10.1105/tpc.104.026161.
- Merelo P, Ram H, Pia Caggiano M, Ohno C, Ott F, Straub D, Graeff M, Cho SK, Yang SW, Wenkel S, et al. Regulation of MIR165/166 by class II and class III homeodomain leucine zipper proteins establishes leaf polarity. Proc Natl Acad Sci. 2016;113(42):11973–11978. doi:10.1073/pnas.1516110113.
- Siegfried KR, Eshed Y, Baum SF, Otsuga D, Drews GN, Bowman JL. Members of the YABBY gene family specify abaxial cell fate in Arabidopsis. Development. 1999;126(18):4117–4128. doi:10.1242/dev.126.18.4117.
- Bowman JL. The YABBY gene family and abaxial cell fate. Curr Opin Plant Biol. 2000;3(1):17–22. doi:10.1016/S1369-5266(99)00035-7.
- Yang H, Shi G, Li X, Hu D, Cui Y, Hou J, Yu D, Huang F. Overexpression of a soybean YABBY gene, GmFILa, causes leaf curling in Arabidopsis thaliana. BMC Plant Biol. 2019;19(1):234. doi:10.1186/s12870-019-1810-2.
- Zhang G-H, Xu Q, Zhu X-D, Qian Q, Xue H-W. SHALLOT-LIKE1 is a KANADI transcription factor that modulates rice leaf rolling by regulating leaf abaxial cell development. Plant Cell. 2009;21(3):719–735. doi:10.1105/tpc.108.061457.
- Kerstetter RA, Bollman K, Taylor RA, Bomblies K, Poethig RS. KANADI regulates organ polarity in Arabidopsis. Nature. 2001;411(6838):706–709. doi:10.1038/35079629.
- Eshed Y, Baum SF, Perea JV, Bowman JL. Establishment of polarity in lateral organs of plants. Curr Biol. 2001;11(16):1251–1260. doi:10.1016/S0960-9822(01)00392-X.
- Zumajo-Cardona C, Vasco A, Ambrose B. The evolution of the KANADI gene family and leaf development in lycophytes and ferns. Plants. 2019;8(9):313. doi:10.3390/plants8090313.
- Merelo P, Paredes EB, Heisler MG, Wenkel S. The shady side of leaf development: the role of the REVOLUTA/KANADI1 module in leaf patterning and auxin-mediated growth promotion. Curr Opin Plant Biol. 2017;35:111–116. doi:10.1016/j.pbi.2016.11.016.
- Williams L, Grigg SP, Xie M, Christensen S, Fletcher JC. Regulation of Arabidopsis shoot apical meristem and lateral organ formation by microRNA miR166g and its AtHD-ZIP target genes. Development. 2005;132(16):3657–3668. doi:10.1242/dev.01942.
- Song JB, Huang SQ, Dalmay T, Yang ZM. Regulation of leaf morphology by MicroRNA394 and its target LEAF CURLING RESPONSIVENESS. Plant Cell Physiol. 2012;53(7):1283–1294. doi:10.1093/pcp/pcs080.
- Li L-C, Kang D-M, Chen Z-L, Qu L-J. Hormonal regulation of leaf morphogenesis in Arabidopsis. J Integr Plant Biol. 2007;49(1):75–80. doi:10.1111/j.1744-7909.2006.00410.x.
- Pekker I, Alvarez JP, Eshed Y. Auxin response factors mediate Arabidopsis organ asymmetry via modulation of KANADI activity. Plant Cell. 2005;17(11):2899–2910. doi:10.1105/tpc.105.034876.
- Hunter C, Willmann MR, Wu G, Yoshikawa M, de la Luz Gutierrez-Nava M, Poethig SR. Trans-acting siRNA-mediated repression of ETTIN and ARF4 regulates heteroblasty in Arabidopsis. Development. 2006;133(15):2973–2981. doi:10.1242/dev.02491.
- Mockaitis K, Estelle M. Auxin receptors and plant development: a new signaling paradigm. Annu Rev Cell Dev Biol. 2008;24(1):55–80. doi:10.1146/annurev.cellbio.23.090506.123214.
- Teale WD, Paponov IA, Palme K. Auxin in action: signalling, transport and the control of plant growth and development. Nat Rev Mol Cell Biol. 2006;7(11):847–859. doi:10.1038/nrm2020.
- Overvoorde PJ, Okushima Y, Alonso JM, Chan A, Chang C, Ecker JR, Hughes B, Liu A, Onodera C, Quach H, et al. Functional Genomic Analysis of the AUXIN/INDOLE-3-ACETIC ACID gene family members in Arabidopsis thaliana. Plant Cell. 2005;17(12):3282–3300. doi:10.1105/tpc.105.036723.
- Reed JW. Roles and activities of Aux/IAA proteins in Arabidopsis. Trends Plant Sci. 2001;6(9):420–425. doi:10.1016/S1360-1385(01)02042-8.
- Zhao B, Wang B, Li Z, Guo T, Zhao J, Guan Z, Liu K. Identification and characterization of a new dwarf locus DS-4 encoding an Aux/IAA7 protein in Brassica napus. Theor Appl Genet. 2019;132(5):1435–1449. doi:10.1007/s00122-019-03290-8.
- Zou L-P, Sun X-H, Zhang Z-G, Liu P, Wu J-X, Tian C-J, Qiu J-L, Lu T-G. Leaf rolling controlled by the homeodomain leucine zipper class IV gene Roc5 in rice. Plant Physiol. 2011;156(3):1589–1602. doi:10.1104/pp.111.176016.
- Fang J, Guo T, Xie Z, Chun Y, Zhao J, Peng L, Zafar SA, Yuan S, Xiao L, Li X, et al. The URL1–ROC5–TPL2 transcriptional repressor complex represses the ACL1 gene to modulate leaf rolling in rice. Plant Physiol. 2021;185(4):1722–1744. doi:10.1093/plphys/kiaa121.
- Juarez MT, Twigg RW, Timmermans MCP. Specification of adaxial cell fate during maize leaf development. Development. 2004;131(18):4533–4544. doi:10.1242/dev.01328.
- Fahlgren N, Montgomery TA, Howell MD, Allen E, Dvorak SK, Alexander AL, Carrington JC. Regulation of AUXIN RESPONSE FACTOR3 by TAS3 ta-siRNA affects developmental timing and patterning in Arabidopsis. Curr Biol. 2006;16(9):939–944. doi:10.1016/j.cub.2006.03.065.
- Khosla A, Paper JM, Boehler AP, Bradley AM, Neumann TR, Schrick K. HD-Zip proteins GL2 and HDG11 have redundant functions in Arabidopsis trichomes, and GL2 activates a positive feedback loop via MYB23. Plant Cell. 2014;26(5):2184–2200. doi:10.1105/tpc.113.120360.
- Huang H, Alvarez S, Bindbeutel R, Shen Z, Naldrett MJ, Evans BS, Briggs SP, Hicks LM, Kay SA, Nusinow DA, et al. Identification of evening complex associated proteins in Arabidopsis by affinity purification and mass spectrometry. Mol Cell Proteomics. 2016;15(1):201–217. doi:10.1074/mcp.M115.054064.
- Wojciechowska I, Mukherjee T, Knox-Brown P, Hu X, Khosla A, Mathews GL, Thompson KA, Peery ST, Szlachetko J, Thalhammer A, Hincha DK, Skirycz A, Schrick K .Arabidopsis PROTODERMAL FACTOR2 binds lysophosphatidylcholines and transcriptionally regulates phospholipid metabolism. bioRxiv 2021. doi:10.1101/2021.10.20.465175.
- Clough SJ, Bent AF. Floral dip: a simplified method for Agrobacterium-mediated transformation of Arabidopsis thaliana. Plant J. 1998;16(6):735–743. doi:10.1046/j.1365-313x.1998.00343.x.
- Murashige T, Skoog F. A revised medium for rapid growth and bio assays with tobacco tissue cultures. Physiol Plant. 1962;15(3):473–497. doi:10.1111/j.1399-3054.1962.tb08052.x.
- Farmer E, Mousavi S, Lenglet A. 2013 Leaf numbering for experiments on long distance signalling in Arabidopsis. Protocol Exchange. doi:10.1038/protex.2013.071.
- Shi ZY, Wang J, Wan XS, Shen,GZ, Wang XQ Zhang JL. Over-expression of rice OsAGO7 gene induces upward curling of the leaf blade that enhanced erect-leaf habit. Planta. 2006;226:99–108.