ABSTRACT
Phytosulfokines (PSKs) are a class of tyrosine-sulfated pentapeptides. PSK-α, PSK-γ, and PSK-δ are three reported PSK members involved in regulating plant growth, development, and resistance to biotic and abiotic stresses. Here, we reported a novel type of PSK, PSK-ε with the sequence YSO3VYSO3TN, and its precursor proteins (MtPSKε, LjPSKε, and GmPSKε), specifically from legume species. PSK-ε peptide differs from PSK-δ by one amino acid and is close to PSK-δ in the phylogenetic relationship. Expression profile analysis showed that MtPSKε was highly expressed in Medicago truncatula roots, especially in root tips and emerged lateral roots. Application of the synthetic sulfated PSK-ε peptide and overexpression of MtPSKε significantly promoted M. truncatula root elongation and increased lateral root number, probably by inducing cell division and expansion in roots. Furthermore, MtPSKε expression was induced by rhizobia infection and was detected in root nodules including nodule primordia. Both PSK-ε peptide treatment and MtPSKε overexpression significantly increased nodule number in M. truncatula. Taken together, these results demonstrate that PSK-ε, a novel type of phytosulfokine, positively regulates root elongation and formation of lateral root and root nodule in M. truncatula.
1. Introduction
Root system plays an important role in absorption, transport and storage of water and nutrients beyond anchoring and supporting functions. In order to achieve more efficient nutrient acquisition and utilization, root system must flexibly adjust growth and development to adapt to the heterogeneous soil environment.Citation1 Internal developmental factors and external environmental signals coordinately shape root system architecture.Citation2 Legumes can interact with the Rhizobium in the soil to establish a symbiotic relationship, forming a new root-derived organ called root nodule, in which atmospheric dinitrogen is converted to ammonia to support plant growth.Citation3 At the molecular level, coordinating plant root development, including root elongation and lateral root formation, and nodulation process are regulated by a complex signaling network. Further investigation of key factors in this network is of great importance to improve the growth and yield of leguminous crops.
Phytosulfokine (PSK) is a kind of tyrosine-disulfated pentapeptide hormone which is widespread across the plant kingdom.Citation4 The first identified PSK member is PSK-α with the sequence YSO3IYSO3TQ.Citation5 A disulfated tetrapeptide with the sequence Y SO3IYSO3T was also reported and named PSK-β which, however, was found to be a degradation product of PSK-α.Citation6 PSK-α peptides are produced from precursor proteins of about 80–120 amino acids encoded by the PSK gene family.Citation4 These precursor proteins contain an N-terminal signal peptide sequence and the PSK motif near the C-terminus. PSK-α peptides mature through tyrosine sulfation catalyzed by the tyrosylprotein sulfotransferase (TPST) in the Golgi apparatusCitation7 followed by proteolytic cleavage in the apoplast.Citation8,Citation9 Mature PSK-α peptides function through a plasma membrane-localized module comprising the receptor kinase PSKR, putative co-receptor BAK1, CNGC17, and H+-ATPases.Citation10,Citation11 Genetic study and peptide application assay have shown that PSK-α promotes the growth of root, hypocotyl, and leaf by inducing cell expansion or elongation in these organs.Citation12–14 It has also been reported that PSK-α induces cell division both in vivo and in vitro.Citation5,Citation15 Moreover, PSK-α also participates in regulating somatic embryogenesis,Citation16 drought-induced flower drop,Citation17 in vitro regeneration of recalcitrant legume,Citation18 and immune responses.Citation19–21
Our previous work in legumes have identified two analogues of PSK-α peptide, named PSK-γCitation22 and PSK-δ.Citation23 PSK-γ is a disulfated pentapeptide with the sequence YSO3VYSO3TQ, different from PSK-α only at the second amino acid. Precursor genes encoding PSK-γ peptide are primarily expressed in soybean seeds. Overexpression of PSK-γ genes remarkably promoted seed growth by inducing embryo cell expansion.Citation22 PSK-δ is a recently identified disulfated pentapeptide with the sequence YSO3IYSO3TN, distinguishing from PSK-α only at the last amino acid. PSK-δ, as a legume species-specific peptide, accumulates primarily in root nodules. Both application of PSK-δ peptide and genetic manipulation showed that PSK-δ promotes M. truncatula nodulation by enhancing nodule organogenesis.Citation23
In this study, we identified a novel type of PSK, PSK-ε with the sequence YSO3VYSO3TN, specifically from legume species. In M. truncatula, the PSK-ε-encoding precursor gene MtPSKε was primarily expressed in root tips, emerged lateral roots, and root nodules at all developmental stages. Application of the synthetic sulfated PSK-ε peptide and overexpression of MtPSKε in M. truncatula promoted root elongation and increased numbers of lateral roots and root nodules, probably by inducing cell division and expansion. These findings suggest that PSK-ε positively regulates root elongation and formation of lateral roots and root nodules in legumes and can be exploited to improve crop growth and yield.
2. Materials and methods
2.1. Plant materials and growth condition
The Medicago truncatula Jemalong A17 ecotype and Arabidopsis thaliana Columbia-0 ecotype were used for transgenic assays. Seeds of M. truncatula were immersed in H2SO4 for 8 min, rinsed three times with distilled water, surface sterilized with 10% (v/v) NaClO for 3 min and rinsed with distilled water for 6 times. After surface sterilizing, seeds were spread on 1% (w/v) agar medium, and stratified at 4°C for 2 days. Then the seeds were germinated at 28°C in the dark for 12 h. Arabidopsis seeds were surface sterilized for 15 min in 5% (v/v) NaClO solution containing 0.2% (v/v) Tween-20, washed three times with sterilized water, and spread on Murashige and Skoog medium (Duchefa) containing 1% (w/v) sucrose and 0.7% (w/v) agar. Plates were stratified at 4°C for 2 days and then placed in a greenhouse for germination. M. truncatula and Arabidopsis seedlings were transplanted to pots containing a 3:1 ratio of vermiculite: perlite and cultured in a greenhouse with a light/dark cycle of 16 h/8 h at 23°C and 50% relative humidity.
2.2. qRT-PCR gene expression analysis
The plant tissues were homogenated in liquid nitrogen, and the total RNA was isolated using RNAprep pure plant kit (Tiangen, Beijing, China). A NanoDrop spectrophotometer (Thermo Fisher, Waltham, MA, USA) was used to quantify the RNA concentration and the first-strand cDNA was synthesized using the HiScript II Q-RT Supermix kit (Vazyme, Nanjing, China). For gene expression analysis, qRT–PCR experiments were carried out on a CFX96 real-time PCR detection system (Bio–Rad, Hercules, CA, USA) with Hieff qPCR SYBR Green Master Mix (Yeasen, Shanghai, China). PCR conditions and data analysis methods were described in our previous study.Citation24 The housekeeping genes used in qRT–PCR experiments were MtActinB or AtActin2, and the specific primers are listed in Table S1.
2.3. Gene cloning and vector construction
For promoter-GUS assay, the promoter region (approximately 2000 bp upstream of start codon) of MtPSKε was cloned from M. truncatula genomic DNA by PCR and ligated into the binary vector pBI121 to drive the expression of the GUS (β-glucuronidase) gene. For Overexpression constructs, the MtPSKε CDS was PCR amplified from M. truncatula cDNA, and was ligated into the intermediate vector pA7 downstream of the Cauliflower mosaic virus (CaMV) 35S promoter using the restriction sites XhoI and SacI. The resulting 35S:MtPSKε:OCSter cassette was subsequently ligated into a modified binary vector pCambia1300 using the restriction sites HindIII and EcoRI. The modified pCambia1300 vector contains a 35S:GFP module in the T-DNA region, facilitating GFP fluorescence screening of transgenic hairy roots. The primers for the constructs are listed in Table S1.
2.4. Exogenous peptide treatment
PSK-ε (YSO3VYSO3TN) peptide and randomly arranged pentapeptide (TYNYV) were synthesized by Chinese Peptide Company (Hangzhou, China). Each peptide was stored at 1 mM in sterilized double-distilled water as a stock solution. To investigate the effect of PSK-ε on root growth, M. truncatula seedlings were grown on FM plates containing 1 μM corresponding synthetic peptides. Seedlings were photographed and the lateral roots were counted. For the nodulation assay, germinated seeds of M. truncatula were grown on FM plates supplemented with corresponding peptides at a concentration of 1 μM, and S. meliloti 2011 was suspended in FM liquid solution (OD600 = 0.02) and flood-inoculated onto roots. After 20 days of growth on plates in the vertical position, the nodules were photographed and counted.
2.5. Hairy root transformation
For hairy root transformation, the 35S:MtPSKε plasmid were introduced into the A. rhizogenes ARqua1 strain. The recombinant Arqua1 was used to transform M. truncatula roots according to a well established method.Citation25 The positive transgenic roots were selected based on GFP fluorescence examined under a SMZ18 fluorescence stereomicroscope (Nikon, Tokyo, Japan). The transgenic plants were transplanted to pots and inoculated with S. meliloti 2011. After 3 weeks of growth, the nodulation phenotype was analyzed.
3. Results
3.1. Sequence and phylogeny of legume-specific PSK-ε precursor proteins
Through searching genome databases of legume species (phytozome-next.jgi.doe.gov) using reported PSK precursor sequences as queries, we identified a class of legume-specific genes encoding putative PSK pentapeptides. These predicted pentapeptides with the sequence YVYTN differ from PSK-γ (YVYTQ) by the last amino acid (substitution of glutamine by asparagine), and differ from PSK-δ (YIYTN) by the second amino acid (substitution of isoleucine by valine) (), therefore, the new peptide is named PSK-ε in accordance with the naming convention. Similar to PSK-δ, PSK-ε exists only in legume species. In the genomes of M. truncatula (A17), L. japonicus (MG-20) and G. max (Williams 82), one gene was found to encode the PSK-ε precursor protein, named MtPSKε (Medtr8g091700), LjPSKε (Lj4g0007102) and GmPSKε (Glyma.08G140800), respectively (). Like the reported PSK members, all these PSK-ε precursor genes contain two exons (Fig. S1), and the deduced proteins consist of approximately 80 amino acids. Sequence alignment showed that these PSK-ε precursors were highly conserved (78–85% sequence similarity), and were more similar with PSK-δ than with PSK-α and PSK-γ (). As predicted by SignalP-5.0 (www.cbs.dtu.dk/services/SignalP/), all PSK-ε precursors harbor N-terminal signal peptide sequences for targeting the secretory pathway, the PSK-ε pentapeptide motifs and the conserved aspartic acid at the −1 position of pentapeptide were identified at the C-terminus ().
Figure 1. Legume-specific PSKε genes are predicted to encode a novel type of phytosulfokine precursor protein. (a) Sequence alignment of representative PSKα, PSKγ, PSKδ, and predicted PSKε precursor proteins in Medicago truncatula, Lotus japonicus, and Glycine max. Conserved and similar amino acid residues are shaded in black and gray, respectively. The PSK pentapeptide motifs near the C-terminus of PSK precursors are boxed in red. The predicted signal peptide sequences at the N-terminus are underlined. (b) Phylogenetic tree of PSKα, PSKγ, PSKδ, and PSKε members in Arabidopsis and three legume species. Legume-specific PSKε precursor proteins cluster in one phylogenetic clade, as indicated by a red dashed-line box. The tree was constructed using MEGA6 software with the neighbor-joining method, and bootstrap values from 1000 replications are included.
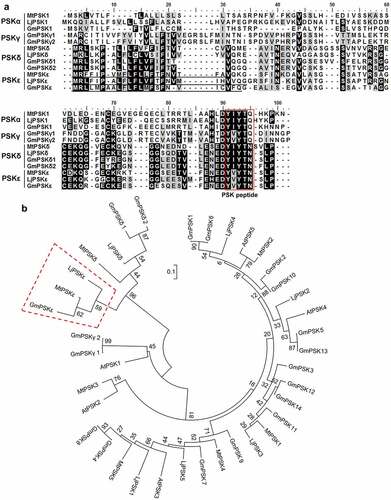
Phylogenetic analysis revealed that the legume-specific PSK-ε precursor proteins MtPSKε, LjPSKε, and GmPSKε have a closer relationship with PSK-δ proteins, which are also legume-specific. PSK-ε and PSK-δ members are clustered into one clade (), forming a PSK sub-family. These results indicate that, like PSK-δ, PSK-ε has a specific function in legume growth and development.
3.2. Expression pattern of PSK-ε precursor genes in M. truncatula
To investigate the expression pattern of the PSK-ε precursor gene MtPSKε, cDNA from various organs of M. truncatula were prepared for quantitative RT-PCR assay. The results showed that MtPSKε was highly expressed in roots and weakly in nodules, while in other organs (leaf, stem, flower, and pod), was expressed at extremely low levels (). To investigate whether MtPSKε is regulated by rhizobia infection, the transcription level of MtPSKε was examined in M. truncatula roots at 1, 3, 5, and 7 d post-inoculation (dpi) with S. meliloti 2011. As shown in , MtPSKε expression was upregulated upon rhizobia inoculation at an early stage (1 dpi) and each stage followed, indicating that MtPSKε expression in root is induced by rhizobia. Additionally, published data of nodule laser capture micro-dissection coupled to RNA-seq showed that MtPSKε is predominantly expressed in the apical meristem and partial infection zone of developing M. truncatula nodules ().
Figure 2. Expression pattren of MtPSKε in M. truncatula. (a) Expression pattern of MtPSKε determined by qRT–PCR. cDNA was prepared from wild-type M. truncatula (A17 ecotype) organs, including roots, root nodules (14 d post-inoculation, 14 dpi), leaves, stems, flowers, and pods. qRT–PCR was employed to detect MtPSKε transcript levels in the various tissues. Expression of MtActinB was used as an internal control. Statistically significant differences indicated by different letters were determined with one-way ANOVA (P < .05) (b) Expression of MtPSKε in M. truncatula roots inoculated with S. meliloti 2011 at different times (1–7 dpi) with expression in uninoculated roots as controls. Statistical significance was evaluated by Student’ s t test; * P < .05, ** P < .01. (c) Expression level of MtPSKε in different regions of developing nodules at 15 dpi. FI, meristematic zone; FIId, distal infection zone; FIIp, proximal infection zone; IZ, interzone; ZIII, nitrogen-fixation zone. The data was collected from published RNA-Seq data (https://iant.toulouse.inra.fr/symbimics/). Values represent the means ± SE of three biological replicates. (d-i) Histochemical staining of pMtPSKε:GUS transgenic hairy roots of M. truncatula (A17). GUS activity detected in transgenic primary roots (d) and emerged lateral roots (e). GUS staining of root nodule primordia at 4 dpi (f), young nodules (7 dpi, G), elongating nodules (12 dpi, H), and mature nodules (20 dpi, I). Scale bars = 500 μm.
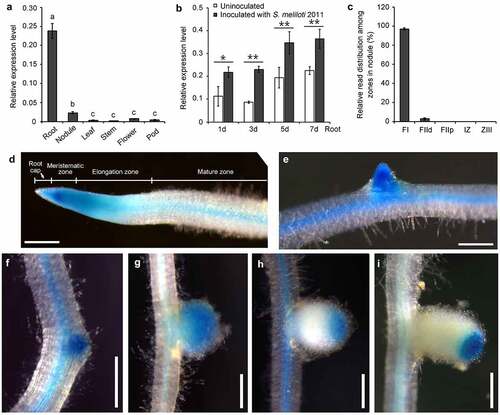
To further determine the spatiotemporal expression of MtPSKɛ, we cloned the promoter of MtPSKɛ and fused it with the GUS coding gene. The resulting pMtPSKɛ:GUS construct was transformed into M. truncatula hairy roots mediated by Agrobacterium rhizogenes. Histochemical staining showed that pMtPSKɛ:GUS was expressed in the tip of primary roots (), emerged lateral roots (), and root vascular tissues (). In root tip, the strongest GUS activity was detected in the meristematic zone and lower activity in elongation zone and root cap (). Moreover, pMtPSKɛ:GUS was expressed throughout the root nodule developmental process. Strong GUS signal was detected as early as in the nodule primordia (), and was persistently detected in the whole young nodules (), where active cell division and expansion occurred. In elongating () and mature () nodules, GUS signal was restricted to the apical regions, corresponding mainly to meristematic and partial infection zones, consistent with the findings in . These results demonstrate that MtPSKɛ gene is mainly expressed in root and nodule regions where cell division and expansion are active, suggesting a key role of PSK-ɛ peptide in regulating root and nodule growth and development.
3.3. PSK-ε peptide promotes root system development
To investigate the biological function of PSK-ε peptide in plant growth and development, we supplied 1 μM chemically synthesized PSK-ε pentapeptide exogenously to wild-type M. truncatula seedling grown on vertical plates. Seedlings treated with randomly arranged pentapeptide at the same concentration were used as a control. After 7 days of growth, we found that the length of primary root treated with PSK-ε is 23% longer than that of the random peptide treatment (). After 20 days of growth, the PSKε-treated M. truncatula plants generated more lateral roots compared to the random peptide control ().
Figure 3. PSK-ε peptide promotes root development. (a-c) Exogenous application of synthetic PSK-ε peptide in M. truncatula. (a) Seven-day-old M. truncatula (A17) seedlings grown on vertical FM plates containing 1 μM PSK-ε peptide or 1 μM randomly arranged pentapeptide. Scale bars = 2 cm. (b) Primary root length of M. truncatula seedlings treated with PSK-ε or random peptide for 7 days (n = 25). (c) Lateral root number of PSK-ε and random peptide-treated M. truncatula plants at 20 days of age (n = 20). (d-h) Overexpression of MtPSKε in M. truncatula (A17) transgenic hairy roots. (d) Comparison of MtPSKε overexpressing (MtPSKεOX) transgenic roots and the empty vector (EV) control roots at 20 d post-inoculation (dpi). Scale bar = 2 cm. (e) Semi-quantitative RT–PCR analysis of MtPSKε transcript abundance in underground tissues (including roots and nodules) of the control and MtPSKεOX plants at 20 dpi. Expression of MtActinB was used as an internal control. (f) Root length of the MtPSKεOX and control plants at 20 dpi. (g) Number of lateral roots formed on 20 dpi MtPSKεOX and control transgenic roots. (h) Lateral root number per unit length (cm) of the transgenic roots. In (f-h), n = 24 for MtPSKεOX, n = 25 for empty vector control. (i-m) Heterologous overexpression of MtPSKε or GmPSKε promotes root growth in Arabidopsis. (i) Eight-day-old wild-type and transgenic Arabidopsis seedlings grown on vertical MS-agar plates. Col-0 seeds from two stocks were used as wild-type controls. Scale bars = 2 cm. (j) qRT-PCR analyses of MtPSKε and GmPSKε transcript levels in MtPSKε and GmPSKε-overexpressing Arabidopsis lines. Values are the means ± SE of three independent biological replicates normalized against the reference gene AtActin2. (k) Primary root length of the wild-type and transgenic Arabidopsis lines showed in (I). (l) Number of lateral roots formed on the wild-type and transgenic Arabidopsis lines showed in (I). (m) Lateral root number per unit length (cm) of the wild-type and transgenic Arabidopsis roots. Values of root length and lateral root number are means ± SE. n > 25. In (B, C, F, G, H), statistical significance was evaluated by Student’ s t test; * P < .05, ** P < .01. In (k-m), statistically significant differences indicated by different letters were determined with one-way ANOVA, P < .05.
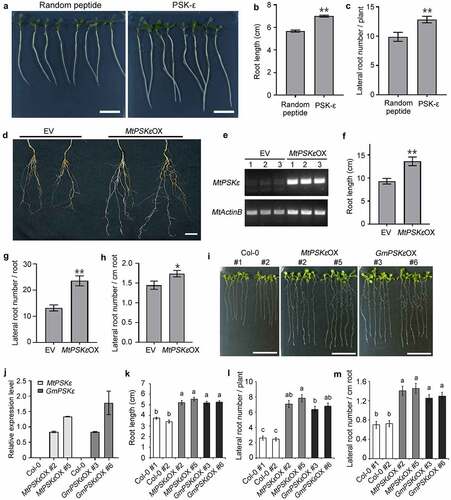
To further determine the effect of PSK-ε on growth and development of the root system, we constructed MtPSKε overexpression vector driven by the enhanced cauliflower mosaic virus (CaMV) 35S promoter and transformed into M. truncatula hairy roots mediated by Agrobacterium rhizogenes. Semi-quantitative RT-PCR showed that MtPSKε was successfully overexpressed in transgenic hairy roots compared with the empty vector transformation roots (). Phenotypic observation revealed that the MtPSKε-overexpressing transgenic plants developed better root system than did the empty vector control (). Specifically, the length of primary roots () and the number of lateral roots () increased by 46% and 78%, respectively; and the lateral root density was also increased (). These results coincide with the findings of exogenous peptide treatments.
Additionally, we constructed constitutive expression vectors of MtPSKε and GmPSKε under the control of 35S promoter, and transformed into Arabidopsis mediated by Agrobacterium tumefaciens. For each gene, more than 16 homozygous transgenic lines (T3 generation) were obtained. Among these lines, MtPSKε-overexpressing lines 2 and 5, and GmPSKε-overexpressing lines 3 and 6 were highly expressed (). For phenotypic observation, overexpression lines and wild-type Arabidopsis were grown on vertical plates for 8 days. The results showed that both MtPSKε and GmPSKε overexpression significantly increased root length (), number of lateral roots per plant (), and density of lateral roots (). Microexamination revealed that expression of MtPSKε or GmPSKε in Arabidopsis increased root meristem size and cell length, leading to longer roots (Fig. S2). Therefore, PSK-ε positively regulates the root development by promoting root growth and lateral root formation in plants.
3.4. PSK-ε promotes nodulation in M. truncatula
Based on the findings that MtPSKε expression is induced by rhizobia and is throughout the root nodule developmental process, it is reasonable to presume that PSK-ε is involved in the regulation of nodule formation in legumes. To test this assumption, we supplied 1 μM synthesized PSK-ε peptide or randomly arranged pentapeptide exogenously to M. truncatula seedling grown on vertical nitrogen-free plates, and then inoculated them with S. meliloti. At 20 dpi, the plants treated with PSK-ε peptide formed approximately one-fold more nodules than did the control plants (). To validate the function of PSK-ε in nodulation, 35S:MtPSKε construct was transformed into M. truncatula hairy roots. At 20 dpi of S. meliloti, phenotypical observation revealed that although the shape and size of nodules were not obviously altered, the number of nodules on MtPSKε-overexpressing transgenic roots was increased by 39% relative to control roots (). These results demonstrate that PSK-ε peptide positively regulates root nodule formation.
Figure 4. PSK-ε peptide promotes root nodule formation in M. truncatula. (a) M. truncatula (A17) seedlings inoculated with S. meliloti 2011 for 20 days were grown on FM agar plates supplemented with 1 μM synthetic peptides (PSK-ε or random peptide). (b) Statistic analysis of the nodule number on PSK-ε and random peptide-treated M. truncatula seedlings (n = 20). (c, d) Nodulation phenotype of empty vector (c) and MtPSKε-overexpressing (d) transgenic M. truncatula roots at 20 dpi of S. meliloti 2011. Left panels, bright-filed images; right panels, fluorescence images. (e) Numbers of nodules formed on 21 dpi transgenic M. truncatula roots (n = 27). Scale bars = 1 cm in (A), (C), and (D). In (B) and (E), values are means ± SE, statistical significance was evaluated by Student’ s t test; * P < .05, ** P < .01. Experiments were repeated three times with similar results.
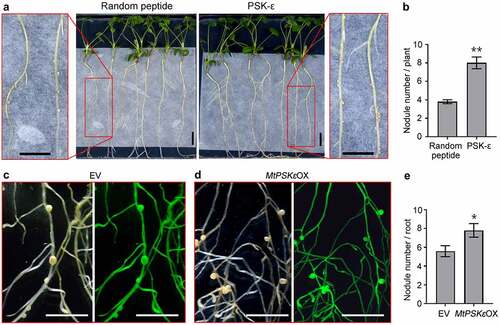
4. Discussion
PSK peptides are widespread across the plant kingdom. The first member PSK-α and its precursor genes have been extensively identified and studied in multiple plant species, revealing their important roles in plant growth, development, and innate immunity.Citation4 Recently, we have identified two new PSK members, PSK-γ and PSK-δ, specifically in legume species. Both PSK-γ and PSK-δ pentapeptides have one amino acid different from the PSK-α peptide and were viewed as analogues of the latter.Citation22,Citation23 PSK-γ was primarily expressed in soybean seeds, and overexpression of its precursor gene remarkably increased seed size through inducing cell expansion;Citation22 while PSK-δ peptide, accumulated mainly in legume nodules, promotes symbiotic nodulation by enhancing nodule organogenesis.Citation23 In this study, we identified a novel type of PSK, PSK-ε, specifically in legume species. Similar to the other PSK members, PSK-ε precursors have a typical protein length and contain N-terminal signal peptide and PSK-ε pentapeptide motifs near the C-terminus. Additionally, PSK-ε differs from PSK-δ peptide only at the second amino acid (the isoleucine in PSK-δ substituted by a valine in PSK-ε). Given that isoleucine and valine are similar in chemical characteristics, it is reasonable to presume that the two types of PSKs share similar biochemical activities. On the other hand, PSK-ε has a closer phylogenetic relationship with PSK-δ than with the other two PSK members. These findings strongly indicate that PSK-ε is a functional analogue of PSK-δ.
Promoter:GUS assay showed that MtPSKε gene was primarily expressed in root tips, emerged lateral roots, and root nodules at all developmental stages, which is similar with the expression profile of MtPSKδ revealed by GUS staining.Citation23 Although the two PSK members exhibit similar expression positions in under-ground tissues, the expression abundance, however, is different. As revealed by qRT-PCR experiments, MtPSKε is highly transcribed in root system and much lower in nodules. Conversely, MtPSKδ is primarily expressed in nodules and only a weak transcript level is detected in roots.Citation23 This differential expression pattern indicates that PSK-ε primarily regulates root growth and development while PSK-δ is mainly involved in nodule formation. Actually, both application of PSK-ε peptide and overexpression of MtPSKε significantly increased root elongation and lateral root number in M. truncatula, and even heterologous expression of MtPSKε or GmPSKε in Arabidopsis remarkably promoted root growth and lateral root formation. In contrast, PSK-δ exhibited only a weak promotion effect on root growth and development.Citation23 Furthermore, GUS staining demonstrated that, in M. truncatula root system, MtPSKε was heavily expressed in meristematic and elongation zones of root tip and in emerged lateral roots where rapid cell division and expansion occurred, indicating that PSK-ε peptide induces cell division and expansion to promote root elongation and lateral root formation. Actually, heterologous expression of MtPSKε or GmPSKε in Arabidopsis induced both cell division and elongation to promote root growth. This mechanism is reminiscent of the previous findings that the other three PSK members function through inducing cell proliferation or cell growth.Citation12–15,Citation22,Citation23 These results demonstrate that as a new PSK member, PSK-ε has similar biochemical activity with the reported PSKs.
In this study, we also found that MtPSKε expression was induced by rhizobia infection and was detected throughout the whole root nodule developmental process. Accordingly, both Application of PSK-ε peptide and overexpression of MtPSKε significantly increased nodule number in M. truncatula. Given that MtPSKε was expressed as early as in the nodule primordia, it is conceivable that PSK-ε peptide might positively regulate primordium initiation to enhance nodule formation. Our previous work reported that PSK-δ promotes M. truncatula nodulation by inducing nodule organogenesis.Citation23 These results suggest that PSK-ε and PSK-δ peptides regulate legume nodulation in a similar mechanism.
Supplemental Material
Download Zip (2 MB)Disclosure statement
No potential conflict of interest was reported by the author(s).
Supplementary material
Supplemental data for this article can be accessed online at https://doi.org/10.1080/15592324.2022.2134672
Additional information
Funding
References
- Lopez-Bucio J, Cruz-Ramirez A, Herrera-Estrella L. The role of nutrient availability in regulating root architecture. Curr Opin Plant Biol. 2003;6(3):280–8. doi:10.1016/S1369-5266(03)00035-9.
- Hodge A, Berta G, Doussan C, Merchan F, Crespi M. Plant root growth, architecture and function. Plant Soil. 2009;321:153–187. doi:10.1007/s11104-009-9929-9.
- Oldroyd GE, Murray JD, Poole PS, Downie JA. The rules of engagement in the legume-rhizobial symbiosis. Annu Rev Genet. 2011;45(1):119–144. doi:10.1146/annurev-genet-110410-132549.
- Sauter M. Phytosulfokine peptide signalling. J Exp Bot. 2015;66(17):5161–5169. doi:10.1093/jxb/erv071.
- Matsubayashi Y, Sakagami Y. Phytosulfokine, sulfated peptides that induce the proliferation of single mesophyll cells of Asparagus officinalis L. Proc Nati Acad Sci USA. 1996;93(15):7623–7627. doi:10.1073/pnas.93.15.7623.
- Yang H, Matsubayashi Y, Nakamura K, Sakagami Y. Oryza sativa PSK gene encodes a precursor of phytosulfokine-α, a sulfated peptide growth factor found in plants. Proc Nati Acad Sci USA. 1999;96(23):13560–13565. doi:10.1073/pnas.96.23.13560.
- Komori R, Amano Y, Ogawa-Ohnishi M, Matsubayashi Y. Identification of tyrosylprotein sulfotransferase in Arabidopsis. Proc Nati Acad Sci USA. 2009;106(35):15067–15072. doi:10.1073/pnas.0902801106.
- Stuhrwohldt N, Buhler E, Sauter M, Schaller A, Bozhkov P. Phytosulfokine (PSK) Precursor processing by SBT3.8 and phytosulfokine signaling contribute to drought stress tolerance in Arabidopsis. J Exp Bot. 2021;72(9):3427–3440. doi:10.1093/jxb/erab017.
- Srivastava R, Liu JX, Howell SH. Proteolytic processing of a precursor protein for a growth-promoting peptide by a subtilisin serine protease in Arabidopsis. Plant J. 2008;56(2):219–227. doi:10.1111/j.1365-313X.2008.03598.x.
- Matsubayashi Y, Ogawa M, Morita A, Sakagami Y. An LRR receptor kinase involved in perception of a peptide plant hormone, phytosulfokine. Science. 2002;296(5572):1470–1472. doi:10.1126/science.1069607.
- Ladwig F, Dahlke RI, Stuhrwohldt N, Hartmann J, Harter K, Sauter M. Phytosulfokine regulates growth in arabidopsis through a response module at the plasma membrane that includes CYCLIC NUCLEOTIDE-GATED CHANNEL17, H+-ATPase, and BAK1. Plant Cell. 2015;27(6):1718–1729. doi:10.1105/tpc.15.00306.
- Kutschmar A, Rzewuski G, Stuhrwohldt N, Beemster GT, Inze D, Sauter M. PSK-alpha promotes root growth in Arabidopsis. New Phytol. 2009;181(4):820–831. doi:10.1111/j.1469-8137.2008.02710.x.
- Stuhrwohldt N, Dahlke RI, Steffens B, Johnson A, Sauter M, Bendahmane M. Phytosulfokine-alpha controls hypocotyl length and cell expansion in Arabidopsis thaliana through phytosulfokine receptor 1. PLoS One. 2011;6(6):e21054. doi:10.1371/journal.pone.0021054.
- Yu L, Liu Y, Luo L . Overexpression of phytosulfokine-alpha induces male sterility and cell growth by regulating cell wall development in Arabidopsis. Plant Cell Rep. 2016;35(12):2503–2512. doi:10.1007/s00299-016-2050-7.
- Heyman J, Cools T, Vandenbussche F, Heyndrickx KS, Van Leene J, Vercauteren I, Vanderauwera S, Vandepoele K, De Jaeger G, Van Der Straeten D, et al. ERF115 controls root quiescent center cell division and stem cell replenishment. Science. 2013;342(6160):860–863. doi:10.1126/science.1240667.
- Hanai H, Matsuno T, Yamamoto M, Matsubayashi Y, Kobayashi T, Kamada H, Sakagami Y. A secreted peptide growth factor, phytosulfokine, acting as a stimulatory factor of carrot somatic embryo formation. Plant Cell Physiol. 2000;41(1):27–32. doi:10.1093/pcp/41.1.27.
- Reichardt S, Piepho HP, Stintzi A, Schaller A. Peptide signaling for drought-induced tomato flower drop. Science. 2020;367(6485):1482–1485. doi:10.1126/science.aaz5641.
- Ochatt S, Conreux C, Mcolo RM, Despierre G, Magnin-Robert J, Raffiot B. Phytosulfokine-alpha, an enhancer of in vitro regeneration competence in recalcitrant legumes. Plant Cell Tiss Org. 2018;135(2):189–201. doi:10.1007/s11240-018-1455-0.
- Igarashi D, Tsuda K, Katagiri F. The peptide growth factor, phytosulfokine, attenuates pattern-triggered immunity. Plant J. 2012;71(2):194–204. doi:10.1111/j.1365-313X.2012.04950.x.
- Mosher S, Seybold H, Rodriguez P, Stahl M, Davies KA, Dayaratne S, Morillo SA, Wierzba M, Favery B, Keller H, et al. The tyrosine-sulfated peptide receptors PSKR1 and PSY1R modify the immunity of Arabidopsis to biotrophic and necrotrophic pathogens in an antagonistic manner. Plant J. 2013;73(3):469–482. doi:10.1111/tpj.12050.
- Shen Y, Diener AC, McDowell JM. Arabidopsis thaliana resistance to fusarium oxysporum 2 implicates tyrosine-sulfated peptide signaling in susceptibility and resistance to root infection. PLoS Genet. 2013;9(5):e1003525. doi:10.1371/journal.pgen.1003525.
- Yu L, Liu Y, Zeng S, Yan J, Wang E, Luo L. Expression of a novel PSK-encoding gene from soybean improves seed growth and yield in transgenic plants. Planta. 2019;249(4):1239–1250. doi:10.1007/s00425-019-03101-w.
- Yu L, Di Q, Zhang D, Liu Y, Li X, Mysore KS, Wen J, Yan J, Luo L. A legume-specific novel type of phytosulfokine, PSK-delta, promotes nodulation by enhancing nodule organogenesis. J Exp Bot. 2022;73(8):2698–2713. doi:10.1093/jxb/erac051.
- Yu L, Chen H, Sun J, Li L. PtrKOR1 is required for secondary cell wall cellulose biosynthesis in Populus. Tree Physiol. 2014;34(11):1289–1300. doi:10.1093/treephys/tpu020.
- Boisson-Dernier A, Chabaud M, Garcia F, Becard G, Rosenberg C, Barker DG. Agrobacterium rhizogenes-Transformed roots of medicago truncatula for the study of nitrogen-fixing and endomycorrhizal symbiotic associations. Mol Plant Microbe Interact. 2001;14(6):695–700. doi:10.1094/MPMI.2001.14.6.695.