Abstract
Two new block copolymers containing photocrosslinkable moieties, poly(2-cinnamoyloxyethyl methacrylate)-block-poly(N-methacryloyloxyethyl N′-adamantyl urea) (PCEMA-b-PMAdU) and poly(2-cinnamoyloxyethyl methacrylate)-block-poly(methyl methacrylate)-block-poly(N-methacryloyloxyethyl N′-adamantyl urea) (PCEMA-b-PMMA-b-PMAdU) were synthesized by atom transfer radical polymerization. The structure, thermal properties, and morphology of the synthesized copolymers were investigated by 1H NMR and Fourier transform infrared/UV/vis spectroscopy, thermogravimetric analysis, differential scanning calorimetry, gel permeation chromatography, atomic force microscopy(AFM), and scanning electron microscopy/environmental scanning electron microscopy. 1H NMR analysis of the copolymer structure confirmed a molar fraction of adamantane units of 0.39 and of 0.66 for diblock and triblock copolymer ,respectively. Special attention was given to the effect of UV/laser irradiation and operating conditions on the block copolymers properties, which could be exploited in the development of micro(nano)structures. The degree of phototransformation of cinnamate groups was between 57 (thin film) and 84% (solution). Modifications of the polymer morphology and surface roughness were induced after laser irradiation (308 and 248 nm) with different fluences (83–203 mJ cm−2). Also, copolymers solutions of different concentrations (c: 25% for PCEMA-b-PMAdU and c: 10, 15 and 20% for PCEMA-b-PMMA-b-PMAdU) were used in electrospinning/electrospraying processes for the preparing of micro/nano particles (0.5–2 μm) and micro/nanofibers (0.6–2.6 μm), the Gaussian distribution curves confirming that the resulting products are dimensionally homogeneous.
1. Introduction
Structurally, adamantane (tricyclo[3.3.1.13,7]-decane) is a highly symmetrical tricyclic hydrocarbon that has fused chair-form cyclohexane rings Citation[1], which exhibits unusual physical and chemical properties as high thermal and oxidation stability, low surface energy, and high hydrophobicity. When the adamantane has been inserted into the macromolecular backbones, for example, in polynaphthol Citation[2], polysiloxane Citation[3], polyfluorene Citation[4], polyimides Citation[5,6], and polymethacrylates Citation[7,8], the oxidative and thermal stability of these polymers was improved. For some of them, the impact of the adamantane on transparency to ultraviolet light, low dielectric constant, hydrophobicity, low surface energy and high density was also demonstrated. Although these features are attractive for a broad range of applications including biomedical ones, relatively less attention has been paid to the study of acrylic polymers using adamantane containing monomers in different polymerization techniques, as conventional radical polymerization Citation[9], living anionic polymerization Citation[10], and controlled radical polymerization, to name a few Citation[11–13]. Therefore, the use of polymer synthesis in a controlled manner (e.g. atom transfer radical polymerization (ATRP), reversible addition-fragmentation chain transfer polymerization) for producing complex macromolecular architectures with well-defined molecular weights, low polydispersities and specialized functionalities could be worthwhile.
On the other hand, given the widespread applications of photosensitive polymers in the photoresists technology (the manufacture of integrated circuits, printing materials, coatings, paints, compact discs, cathode tubes, etc.) Citation[14,15], the study of novel materials has become an especially appealingtarget. Particularly, the polymers with cinnamate groups continue to be an area of great interest, because their photochemistry based mainly on photoisomerization and photodimerization reactions Citation[16,17] which were employed in the development of negative-type photoresists Citation[18]. In connection, some photopolymers as poly(vinylalcohol-co-vinylcinnamate) and polystyrene-b-poly(2-cinnamoylethyl methacrylate) have been prepared and evaluated in terms of photoreactivity and nanostructuring including self-assembly of the former into micellar micro/nanoparticles Citation[19]. In the case of photoreactive (co)polymers with poly(2-cinnamoylethyl methacrylate) units, the formation of nanospheres with a narrow size distribution Citation[20], cross-linked polymeric monolayers Citation[21], thin films with tunable nanochannels Citation[22], superparamagnetic nanotubes Citation[23,24], or nanofibers Citation[25,26] has also been studied.
Another approach of the cinnamates, first reported by Gupta et al. Citation[27], was directed towards the obtaining of submicron fibers with an average diameter in the range of 100 nm–5 μm Citation[28–31] via a simple electrospinning process, which is able to create materials with applications in the area of optical and chemosensor materials, nanocomposites, nanofibers with specific surface chemistry for tissue scaffolds and drug delivery systems Citation[32–34]. The advantages of this powerful technique to produce polymer nanofibers have provided impetus for novel developments in the above mentioned field. Recently, we have reported the synthesis of poly(2-cinnamoyloxyethyl methacrylate) (PCEMA) by microwave-mediated polymerization and ATRP, together with the corresponding block copolymers with methyl methacrylate, accompanied of a study concerning the formation of electrospun micro/nanofibers Citation[35]. Having this in mind, the present work is focused on a combination of the above presented polymers into an attempt to create several polymeric materials. The aim of this paper is to synthesize di- and triblock copolymers with cinnamate and adamantane building blocks to be investigated in the electrospinning and UV/laser irradiation process, together with a discussion of the observed differences in photobehavior and polymer properties, useful in further construction of functional nanocomposite fibers from a mixture of photopolymers and inorganic nanoparticles.
2 Experimental
2.1 Monomer synthesis
Preparation of N-methacryloyloxyethyl N′-adamantyl urea (MAdU) was performed as follows: 2-isocyanatoethyl methacrylate (4.8 mL, 33 mmol) was added drop wise to a 20 mL THF solution of 1-adamantylamine (5 g, 33 mmol) in presence of dibutyltin dilaurate used as the catalyst. The mixture was stirred at 40 °C for 48 h and then the resulting product (MAdU) was concentrated under reduced pressure, washed with diethyl ether, and dried under reduced pressure.
FTIR (KBr): 3351 (NH), 1724 (C=O), 1623 (C=C), 1165 cm−1 (C–O).
2.2 Preparation of poly(2-cinnamoyloxyethyl methacrylate)-Br macroinitiator (PCEMA-Br)
Poly(2-cinnamoyloxyethyl methacrylate)-Br (PCEMA-Br) prepared as previously reported Citation[35] was used as macroinitiator, the molar ratio of the components 2-cinnamoyloxyethyl methacrylate (CEMA)/(N,N,N′,N′,N″-pentamethyldiethylentriamine) (PMDETA)/ethyl 2-bromoisobutyrate (EBiB) /CuBr being 25:2:1:1.).
1H NMR for PCEMA-Br (CDCl3): δ = 7.69 (C6H5–CH=), 6.5(=CHCOO), 7.5 and 7.35 (Ar–H), 4.4–4.0 (COO–CH 2–CH 2–OCO), and 2.1–0.9 (–CH 2 and –CH 3 of PCEMA).
FTIR, PCEMA-Br (KBr): 2900–2994 (C–H), 1716 (CO), and 1165 cm−1 (C–O). M w = 5800 g/mol (PI = 1.13). Conversion: 86.23%.
2.3 Preparation of diblock copolymer (PCEMA-block-PMAdU)
Into a polymerization ampoule maintained under Ar atmosphere 0.0255 g CuBr (0.173 mmol), 0.073 mL PMDETA (0.346 mmol), and 0.794 g MAdU (2.595 mmol) dissolved in 10 ml dioxane were introduced, this mixture being stirred until it becomes homogeneously green. Subsequently, 1 g PCEMA-Br (0.173 mmol; M w = 5800 g/mol) used as ATRP macroinitiator was dissolved in 10 ml dioxane and poured in the above mixture. After the addition of macroinitiator, the system becomes deep green indicating the start of polymerization. The ampoule was heated at 80 °C for 72 h, and then, the reaction mixture was cooled to room temperature and precipitated into diethyl ether. The diblock copolymer, poly(2-cinnamoyloxyethyl methacrylate)-b-poly(N-methacryloyloxyethyl N′-adamantyl urea) (PCEMA-b-PMAdU) was collected after filtration under vacuum and dried. The molar ratio in the reaction mixture was MAdU/PMDETA/PCEMA-Br/CuBr = 15:2:1:1. M w = 8900 g/mol (PI = 1.11). Conversion: 67.53%
FT-IR (KBr): 2849–2906 cm−1 (C–H), 1727 cm−1 (CO), 1639 cm−1 (C=C), 1165 cm−1 (C–O–C), and 864 cm−1(C=C).
2.4 Preparation of PCEMA-block-PMMA-Br macroinitiator
The second macroinitiator, poly(2-cinnamoyloxyethyl methacrylate-block-poly(methyl methacrylate)-Br was obtained by a method described earlier Citation[35], with the mention that the molar ratio of components used in synthesis was MMA/PMDETA/PCEMA-Br/CuBr = 18:2:1:1.
1H NMR for PCEMA-b-PMMA-Br (CDCl3): δ = 7.7 (C6 H5–CH=); 6.5 (=CHCOO) 7.5 and 7.35 (Ar–H); 4.4–4.0 (–COO–CH 2–CH 2–O–CO–); 3.69 (–OCOCH 3 of MMA); and 2.1–0.9 (–CH 2 and –CH 3 of PCEMA and PMMA). M w = 7400 g/mol (PI = 1.15). Conversion: 88.9%
2.5 Preparation of triblock copolymer (PCEMA-block-PMMA-block-PMAdU)
Poly(2-cinnamoyloxyethyl methacrylate)-block-poly(methyl methacrylate)-block-poly(N-mehtacryloyloxyethyl N′-adamantyl urea) (PCEMA-b-PMMA-b-PMAdU) was prepared by ATRP in dioxane using PCEMA-b-PMMA-Br as macroinitiator, PMDETA as ligand, and Cu(I)Br as the catalyst. The reaction mixture, MAdU/PMDETA/PCEMA-b-PMMA-Br/CuBr = 70:2:1:1 (mol) consisting of 2.88 g (9.43 mmol) MAdU, 0.0564 mL (0.268 mmol) PMDETA, 0.0195 g (0.134 mmol) CuBr, and 1 g (0.134 mmol) PCEMA-b-PMMA-Br was introduced into a polymerization ampoule under argon atmosphere at 80 °C for 72 h. The resulting triblock copolymer was purified by precipitation in a large excess of diethyl ether and dried for 48 h at 60 °C under reduced pressure.
FT-IR (KBr): 2849–2906 cm−1 (C–H), 1722 cm−1 (CO), 1639 cm−1 (C=C), 1165 cm−1 (C–O–C), and 864 cm−1(C=C). M w = 25,100 (PI = 1.15). Conversion: 82.6%.
2.6 Electrospinning process
Electrospinning was carried out according to our previous report Citation[35], starting from solutions of block copolymers (PCEMA-b-PMAdU and PCEMA-b-PMMA-b-PMAdU) in a solvent mixture (DMF/chloroform: 3:1 v/v). The electrospinning apparatus was composed of several components: a high-voltage supplier (Gamma High Voltage Research, E3 30P-5 W), a capillary tube with a stainless steel needle, a syringe pump (Harvard Apparatus), and a stainless steel collecting screen. A high electric field from the high voltage supplier is generated between the needle and the collection plate. As it reaches a certain voltage difference, the electrical charge passing through the polymer solution overcomes surface tension of the droplet formed at the tip of the needle. Electrospinning was performed using a syringe with a 1.2 mm diameter spinneret at an applied voltage difference of 15–20 kV over the distance of 15 cm and syringe pump was set to deliver the solution at a flow rate of 1.5 mL h−1. In our experiments, the spinning solutions were kept at room temperature (22 °C), and the syringe/needle were set horizontal baseline to maintain a constant presence of droplet at the tip of the nozzle.
2.7 Measurements
The structures of the monomer, macroinitiators, and polymers were verified by 1H NMR and FT-IR (Fourier transform infrared) spectroscopy using a Bruker 400 MHz spectrometer and Bruker Vertex 70 equipments, respectively. The UV absorption spectra on thin films were measured with a Specord M42 spectrophotometer. The polymer could be cast into a thin film from a N,N-dimethylformamide (DMF) solution (1%). For UV spectral investigation, the films were exposed to UV irradiation with a high-pressure mercury lamp. The spectral changes are reflected in the photoisomerization rate values, for which the rate constant k was determined according to equation:
Gel permeation chromatography (GPC) measurements were made with a PL MD-950 instrument (Polymer Laboratories) equipped with an evaporative mass detector and two 5 m PL gel columns. For measurements, a solution of polymer (1.0 g dL−1) in DMF was used, as the flow rate of the carrier solvent being 1 mL min−1. The average molecular weight was calculated on the basis of the molecular weight vs. retention volume curve of low polydispersity polystyrene standards. The thermal stability of the polymers was analyzed through thermogravimetry using a MOM Q-1500 D (Paulik-Paulik-Erdey) derivatograph (made in Budapest, Hungary) at a heating rate of 10 °C min−1, in air atmosphere. The thermal transitions of thin polymer films were measured on a Perkin–Elmer differential scanning calorimeter (DSC) on the temperature range 20–160 °C with a heating rate of 10 °C min−1, under nitrogen atmosphere. Sample of 10–11 mg was used and the inception of endotherms was taken as a transition temperature.
For laser irradiation of the triblock copolymer, a COMPexPRO 205F (Coherent) excimer laser operating at 248 nm (KrF) and LPXPro 220 excimer laser operating at 308 nm (XeCl), pulse length (τ) 30 and 25 ns, respectively, was used. The surface morphology was examined with a SOLVER PRO-M atomic force microscope (AFM) by tapping mode. For the obtaining of electrospun fibers an electrospinner equipped with a KD Scientific syringe pump and a high voltage source was used in our experiments. The morphology and diameter of the electrospun fibers, were observed using optical microscopy (OM) (LEICA DM 2500 M) and environmental scanning electron microscopy (ESEM) type Quanta 200 (FEI), operating in high vacuum mode at 30 kV with secondary electrons. ESEM studies were performed on a sample fixed by means of colloidal silver or copper supports, the sample being covered with a thin layer of gold, by sputtering (Emitech K550X). The particles and fibers distribution was obtained by determining the particle size with Leica Application. Water contact angle measurements were made on homogeneous surfaces using goniometer KSV Cam 200. Ion-exchanged water droplets of 1 μL in volume were dropped onto the composite films, and the average contact angle was calculated starting from at least 10 separate measurements.
3 Results and discussion
3.1 Synthesis and characterization of block copolymers
Block copolymers bearing cinnamate and adamantane functionalities introduced by means of N-methacryloyloxyethyl N′-adamantyl urea monomer were synthesized according to Scheme . The new monomer (MAdU) was prepared from 2-isocyanatoethyl methacrylate and 1-adamantylamine through a classical addition reaction. The 1H NMR signals (Figure ) of the unsaturated protons (6.07 and 5.63 ppm) from N-methacryloyloxyethyl N′-adamantyl urea, urea protons (5.79 and 5.69 ppm), methylene protons from ester (4.03) together with those adjacent to the urea (3.24 ppm), adamantyl protons (1.9, 1.85 and 1.6 ppm), and methyl protons from methacrylate (1.98 ppm) confirmed the expected structure for MAdU. For obtaining block copolymers, it was necessary to prepare bromo-end capped poly(2-cinnamoylethyl methacrylate) and poly(2-cinnamoylethyl methacrylate)-block-poly(methyl methacrylate (PCEMA-Br; PCEMA-b-PMMA-Br) to be further used as macroinitiator under given ATRP conditions. The choice of the second monomer for synthesis of the latter macroinitiator by similar sequential polymerization of MMA with the PCEMA-Br is motivated of its filmogene qualities desired in the resultant triblock copolymer. The diblock (PCEMA-b-PMAdU) and triblock (PCEMA-b-PMMA-b-PMAdU) copolymers were prepared using N,N,N′,N′,N″-pentamethyldiethylenetriamine (PMDETA) as ligand, CuBr as catalyst, MAdU as comonomer, and one of the synthesized ATRP catalytic macroinitiators. By adjusting the molar ratio between the components taken in synthesis, copolymers with building blocks carrying adamantane and photoreactive cinnamate structures were thus obtained.
Scheme 1 Synthesis of N-methacryloyloxyethyl N′-adamantyl urea (MAdU) and block copolymers with adamantane and cinnamate blocks (PCEMA-b-PMAdU; PCEMA-b-PMMA-b-PMAdU).
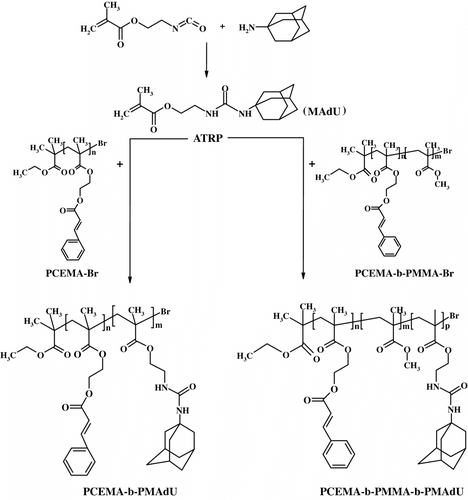
Figure 1 The 1H NMR spectra of N-methacryloyloxyethyl N′-adamantyl urea (a), diblock copolymer PCEMA-b-PMAdU (b), and triblock copolymer PCEMA-b-PMMA-b-PMAdU (c) in DMSO-d6.
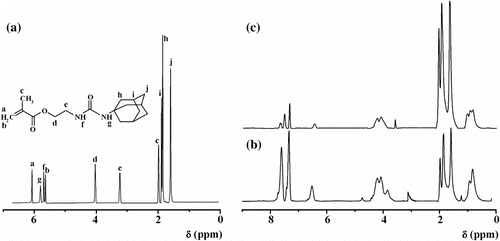
The structures and purity of the block copolymers were confirmed by 1H NMR, FT-IR, and UV spectroscopy. Figure shows the 1H NMR spectrum of PCEMA-b-PMAdU, where can be observed the resonance peaks associated with unsaturated cinnamoyl proton in the neighborhood of aromatic ring (7.6 ppm, Ar–HC=), aromatic protons in ortho (7.43 ppm) and para position (7.33 ppm) to the cinnamoyl double bond, unsaturated cinnamoyl proton linked to the ester sequence (6.6 ppm, =CH–COO–), and with the methylene protons near the ester units (4.5–4 ppm). Other resonance signals belong to the methyne/methylene protons from adamantane moieties and the polymer backbone (1.99, 1.87, and 1.60 ppm), whereas the additional peak at 0.60–0.95 ppm is ascribed to the methyl protons from the methacrylate monomer unit. 1H NMR spectrum of the PCEMA-b-PMMA-b-PMAdU (Figure ) illustrates the presence of the same peaks excepting those due the methyl protons connected to the ester function from PMMA block (3.58 ppm). The number average molecular weight, M n, was calculated from the NMR spectrum of the PCEMA-b-PMAdU diblock copolymer by a comparison of the ratio of the methyne protons from cinnamate (6.43 ppm) with that of the protons from backbone and adamantane unit in the 0.84–1.99 ppm range. The 1H NMR analysis of the diblock copolymer showed that this contains a long PCEMA block (molar fraction is of 0.61) and a shorter adamantane block (molar fraction is of 0.39). The composition of the triblock copolymer was evaluated from its 1H NMR spectrum by the integration ratio of the methyne protons from cinnamate (6.5 ppm) to the methyl protons linked to the ester group from PMMA (3.6 ppm) and the aliphatic protons from the backbone and adamantane (0.85–2.05 ppm). The molar fraction of cinnamate, methyl methacrylate, and adamantane units present in the synthesized triblock copolymer was of 0.21, 0.13, and 0.66, respectively. Therefore, M n, NMR was determined to be 8800 for PCEMA-b-PMAdU and 25,000 for PCEMA-b-PMMA-b-PMAdU. It was observed that the M n values obtained by NMR fit well with those determined from GPC analysis of block copolymers in DMF solution. Figure presents the typical GPC curves of the synthesized polymers. In the chain extension with MAdU, the molecular weight of the PCEMA-b-PMAdU sample increased to 8900 with M w/M n = 1.11, while for PCEMA-b-PMMA-b-PMAdU this increased to 25,100 with M w/M n = 1.15. Therefore, in both cases, complete consumption of the initial macroinitiators was confirmed through a clear shift to a higher molecular weight as observed from the GPC traces. The resulting copolymers exhibit relatively narrow M w/M n values and a unimodal distribution, indicating that the ATRP was an efficient way for the block copolymerization of MAdU using the PCEMA-Br or PCEMA-b-PMMA-Br macroinitiators.
3.2 Thermal analysis
The thermogravimetric analysis (Table ) indicated that the synthesized block copolymers undergo a two-step decomposition process, for which the initial and final decomposition temperatures were determined (Figure ). The thermal decomposition temperature was defined as the temperature of 10% weight loss and it had a value of 250 °C for the diblock copolymer and of 280 °C for the triblock copolymer. The first stage of the thermal decomposition process for both copolymers starts around 215 °C and reaches 400 °C, when more than 50% weight loss was recorded. This result seems to support the view that our photopolymers have a good thermal stability because in the absence of adamantane, the first stage of the thermal decomposition, for instance, in the case of PCEMA and PCEMA-b-PMMA copolymer begins at ∼150 °C, and the temperature of 50% weight loss was about 340 °C Citation[35].
Table 1. The TGA analysis data of the block copolymers.
Figure 3 The TGA curves and differential scanning calorimetric thermograms (a) of PCEMA-b-PMAdU (b) (1), PCEMA-b-PMMA-b-PMAdU (2).
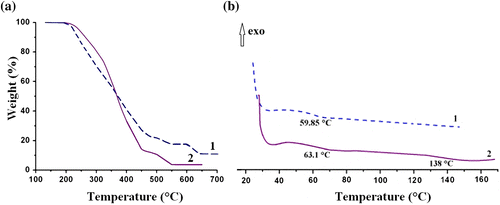
Additionally, phase transition temperatures were determined by DSC measurements. Figure presents the second DSC heating curves for both block copolymers in film state. It appears that the PCEMA-b-PMAdU shows a single endothermic transition corresponding to the glass transition temperature of PCEMA block at 59.85 °C, while for the triblock copolymer two endotherms at 63.1 and 138 °C were recorded in the DSC traces. Such observation may be explained by the compatibility of the blocks in PCEMA-b-PMAdU, and the fact that the DSC is not sensitive enough to distinguish the PMAdU glass transition temperature, most probably due to its relatively short length (molar fraction: 0.39 adamantane units). At this point, we should note that the transition temperature is affected of the higher molecular weight of PCEMA-b-PMMA-b-PMAdU and the longer PMAdU block from copolymer (0.66 adamantane monomer units), and this result is in agreement with the data reported for the other poly(methyl acrylate)s having adamantane in structure Citation[7]. The fact that the T g of the PMMA segments (molar fraction: 0.13) was not identified indicates that the phase separation is not completed in the triblock copolymer obtained with the above composition.
3.3 Photoirradiation experiments
Since it is well documented that the cinnamoyl group undergoes [2 + 2] photocycloaddition with formation of cyclobutane ring Citation[22], we decided to evaluate the photoreactivity of our block copolymers in solid state and in solution. The photocrosslinking reaction of PCEMA-b-PMAdU and PCEMA-b-PMMA-b-PMAdU in DMF or in thin films exposed to UV irradiation was performed at room temperature in the absence of any kind of photosensitizer. Figure displays the changes in the UV absorption band for PCEMA-b-PMAdU solution with increasing irradiation time, when a gradual decrease of the absorption due to the cinnamate groups (278 nm) is observed. Under these conditions, the transformation degree of the photoreactive groups is around 84% after 1530 s of irradiation, and this finding is related to the photoinduced interchain C=C cinnamate dimerization reaction and formation of cyclobutane rings. A similar behavior for PCEMA-b-PMMA-b-PMAdU in solution was evidenced (not shown), with the mention that the double bonds phototransformation degree (PD) was approximately 82% (after 1530 s). In case of the triblock copolymer in thin film (Figure ), the absorption maximum intensity at 276 nm decreases with increasing irradiation time, so that after 1400 s of irradiation, the PD of the cinnamoyl groups was around 78%. In the literature, similar results were reported by Egerton et al. for the earliest synthetic poly(vinyl cinnamate) Citation[36].
Figure 4 The changes in UV spectra of the PCEMA-b-PMAdU copolymer in DMF solution (a) PCEMA-b-PMMA-b-PMAdU copolymer in thin film (b) upon UV irradiation, and their kinetic evaluation (c) including PCEMA-b-PMAdU film.
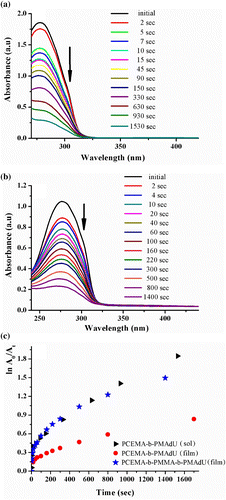
Later on, a plot of the dependence of relative absorbance vs. irradiation time (Figure ) is the proof that the both reactions coexist in copolymer solutions as well as in the thin films. It can be observed that the photocrosslinking reaction in polymer solutions has a rapid evolution and is characterized by similar values of rate constant as follows: k sol = 1.8 × 10−2 s−1 for both block copolymers (PD: ∼84%). In thin films obviously the photocrosslinking reaction occurs much more slowly in PCEMA-b-PMAdU (k film = 0.32 × 10−2 s−1; PD: 57% after 1700 s) than that of PCEMA-b-PMMA-b-PMAdU film (k film = 1.7 × 10−2 s−1; PD: 78%). Such difference can be attributed to the chain mobility induced by means of PMMA in the latter.
Complementary, the photocrosslinking reaction in the triblock copolymer has been monitored by FT-IR spectroscopy. As example, the FTIR spectra within the 1800–1600 cm−1 spectral region for the PCEMA-b-PMMA-b-PMAdU film exposed to different irradiation time are given in Figure . As can be seen, the intensity of the absorption band associated with the carbonyl groups (1721 cm−1) increases in a small extent but this band shifts to 1731 cm−1, while the intensities of the absorption bands due to the C=C groups (
C=C at 1637 cm−1 and
CH at 981 cm−1) continuously decrease with irradiation time. These two effects may be ascribed to the cycloaddition reaction Citation[17,36] which caused a decrease of the amount of C=C units from triblock copolymer and, implicitly, of the number of conjugated carbonyl moieties.
In tandem, the photochemical effects generated by the exposure of PCEMA-b-PMMA-b-PMAdU surface at 308 nm (XeCl) and 248 nm (KrF) were also investigated by AFM. Analysis of surface modification of the polymers is rather a complicated process because this depends on working parameters (wavelength, spot size of the laser beam, energy, and fluence of the light source), as well as on the nature of polymeric material including its morphology, stacking of photodissociated moieties on the surface, formation of pillars, self assembly, surface ablation, etc. Citation[37]. In our experiment, we operated at two wavelengths namely, 308 nm with fluences of 200 and 126 mJ cm−2 and at 248 nm with a fluence of 203 mJ cm−2, 177mJ cm−2, and 83 mJ cm−2, the polymer films being exposed to 5 and 25 pulses. At the first glance, it seems that the surface morphology of the irradiated PCEMA-b-PMMA-b-PMAdU film is modified as visualized by tapping-mode AFM. The surface morphology of the nonexposed areas of polymeric film is displayed in Figure , where a microphase separation with granular structures of block copolymer and a uniform distribution of grains on the surface can be observed. In this case, the value of surface roughness is about 12 nm (10 × 10 μm2, AFM scanned area). Following then, its exposure at 308 nm with a fluence of 200 or 126 mJ cm−2, the morphology and surface roughness of PCEMA-b-PMMA-b-PMAdU film were not severely affected (Figure (b–e)). Similarly, the polymer surface was irradiated at 248 nm with different fluence (203, 177, and 83 mJ cm−2), when the molecules undergo a self-assembling process in few high regular structures (110–200 nm) of conic shape. Furthermore, a decrease of laser fluence at 83 mJ cm−2 increases the height of the structures appeared on the copolymer surface. The surface modification is evidenced from the presented 3D images (Figure (f–i)), where the granular structure disappeared, and other distinct structures relatively uniformly distributed on the surface can be visualized. However, for all energy values, the roughness increased up to three times compared to the nonirradiated sample, and the morphology changed especially by using laser light of 248 nm due to the photocrosslinking, which may force such system to self-organize in nanostructures Citation[38]. Given the investigated block structure, it is reasonable to suppose that the ablated moieties (such as photocleavage of the cyclobutane rings) cannot be neglected. The processing of the AFM images and the calculation of surface texture parameters by the NOVA 9.09 Software (Table ) gave the next value for the root mean square (Sq, defined as the root mean square value of the surface departures within the sample area), on the 10 × 10 μm2 scanned area. The histogram comes to confirm that the increase of the roughness after irradiation (from 11 to 35 nm) is reflected by an increase in the value of the height areas (50–200 nm) on the copolymer surface. According to the contact angle measurements thus obtained, the surfaces showed a modest decrease of hydrophobicity comparatively with nonirradiated ones.
Figure 6 3D AFM images of PCEMA-b-PMMA-b-PMAdU thin film before (a) and after laser irradiation at 308 (b–e) and 248 nm and (f–i) using different fluences and number of pulses.
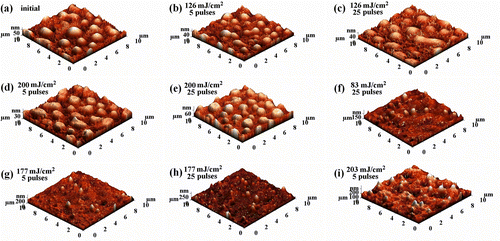
Table 2. Surface parameters determined by the processing of the AFM images collected on the PCEMA-b-PMMA-b-PMAdU film.
3.4 Electrospun/electrospray products
As mentioned, the fiber size and morphology are dependent on the electrospinning conditions as electrospinning voltage (kV), the molecular weight, and the concentration of polymer solution as well as the feeding rate of the polymer solution (mL/h) through the syringe. Based on this consideration, uniform micro/nanofibers mats have been prepared from block copolymer solutions of different concentrations (25 wt.% for PCEMA-b-PMAdU and 20 wt.% for PCEMA-b-PMMA-b-PMAdU), using a solvent mixture DMF/CHCl3 (3:1). The OM images corresponding to the fibrous mats obtained for both block copolymers are shown in Figure , demonstrating the existence of a distinct morphology in the formed electrospun fibers. Therefore, the uniform fibers of copolymer containing a higher amount of adamantane groups (PCEMA-b-PMAdU) have a relatively large diameter (1–8 μm) in comparison with the fibers generated of the triblock copolymer (PCEMA-b-PMMA-b-PMAdU), whose average diameter was in the range of 0.5–4.0 μm. This result could be explained through the effects of molecular weight of the polymer in solution.
Depending on the concentration of polymer solution used in this process, the electrospinning of PCEMA-b-PMMA-b-PMAdU solutions allowed the obtaining of polymeric micro/nanoparticles and micro/nanofibers. Thus, in the next step, uniform micro/nanofiber mats have been prepared at 10, 15 and 20 wt.% concentrations of PCEMA-b-PMMA-b-PMAdU. Figure displays OM images of the resulting products from the electrospinning process of the triblock copolymer solutions namely, nano/microspherical particles (a), beaded nano/microfibers (b), and nano/microfibers (c). It is believed that at a lower polymer concentration (10 wt.%), the liquid jet of the polymer solution breaks up into droplets and subsequently, solvent evaporation led to the formation of polymer particles of microspherical shape. In fact, this result may be explained through the manifestation of electrospraying process Citation[39], which is considered responsible for producing microspherical particles (Figure ). Furthermore, as the concentration of polymer increased, the liquid jet emitted from cone can form beaded-nano/microfibers (Figure ) or nano/microfibers (Figure ). In good agreement with these findings, the ESEM images provided the second evidence for the formation of micro/nano spherical particles (Figure ) and micro/nanofibers (Figure ) based on PCEMA-b-PMMA-b-PMAdU. In this case, spherical particles with diameter ranging from 0.5 to 2 μm and micro/nanofibers with dimensions between 0.6 and 2.6 μm were obtained at higher concentrations of triblock copolymer. Again, the important role of the polymer solution concentration in the formation of such micro/nanostructures is confirmed Citation[40,41], without to neglect the contribution of molecular weight of the block copolymer.
Figure 8 OM images of nano/microspherical particles (a), beaded nano/microfibers (b), and nano/microfibers (c) obtained by electrospinning process of the PCEMA-b-PMMA-b-PMAdU solutions.
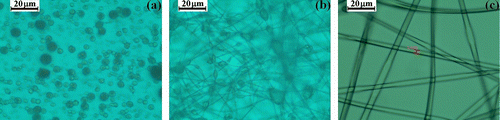
Figure 9 ESEM images of electrospun/electrospray products obtained from (a) 10% solution of triblock copolymer and (b) 20% solution of PCEMA-b-PMMA-b-PMAdU.
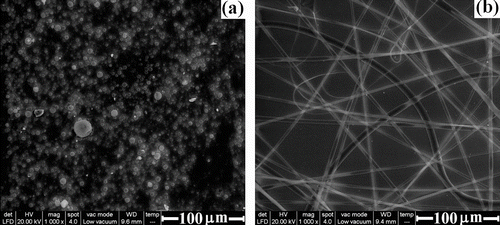
Finally, using the soft Leica Application program, the size distribution of particles and fibers was estimated for about 200 species presented in Figure , which maps the morphology of PCEMA-b-PMMA-b-PMAdU. The bars on the Figure represent ranges of values, whereas the line is an approximation of the Gaussian distribution function, the mean fibers diameter being calculated on the base of this approximation. As shown in Figure ), the copolymer particles prepared in the above conditions have a mean droplet diameter around 1.4 μm. With increasing concentration of triblock copolymer, the morphology undergoes important changes going from particles of spherical shape towards a beaded fiber structure. Hence, beads and fibers with diameter around 1.6 and 0.5 μm as well as uniform nano/microfibers with an average diameter of 2.5 μm were produced in our study, as shown in Figure (b–d). An extension to other macromolecular architectures based on new monomer systems and different nanoparticles is currently under investigation.
4 Conclusions
In this article, the synthesis of a new monomer with adamantane units (MAdU) to be used as comonomer in the ATRP polymerization, initiated by suitable macroinitiators, for preparing di- and triblock copolymers with cinnamate and adamantane building blocks, PCEMA-b-PMAdU and PCEMA-b-PMMA-b-PMAdU is described. The formation of block copolymers was confirmed by 1H NMR spectrometry, FT-IR and UV spectroscopy, thermal analysis, and GPC. The photophysical properties investigation revealed that the synthesized photopolymers can be micro(nano) structured upon UV and laser irradiation, as well as through the electrospinning process.
Acknowledgment
This work was supported by CNCSIS – UEFISCDI, project number PN-II-ID-PCE-2011-3-0164 (Nr. 40/5.10.2011).
References
- Fort , RC Jr and Schleyer , R . 1964 . Adamantane: consequences of the diamondoid structure . Chemical Reviews , 64 : 277 – 300 .
- Matsumoto , K , Shibasaki , Y , Ando , S and Ueda , M . 2006 . Synthesis of novel poly[(1,3-adamantyl)bis(2-naphthol)] with low dielectric constant . Polymer , 47 : 3043 – 8 .
- Hattori , Y , Miyajima , T , Sakai , M , Nagase , Y and Nemoto , N . 2008 . Synthesis and thermal characterization of novel adamantane-based polysiloxane . Polymer , 49 : 2825 – 31 .
- Li , J , Hirayama , Y , Sano , T , Tomita , T , Fujii , H and Wakisaka , K . 2006 . Reduction of chain interactions in a class of blue fluorene copolymers with adamantane units . Thin Solid Films , 515 : 2686 – 91 .
- Kung , YC , Liou , GS and Hsiao , SH . 2009 . Synthesis and characterization of novel electroactive polyamides and polyimides with bulky 4-(1-adamantoxy)triphenylamine moieties . Journal of Polymer Science Part A: Polymer Chemistry , 47 : 1740 – 55 .
- Hsiao , SH , Liou , GS , Kung , YC , Pan , HY and Kuo , CH . 2009 . Electroactive aromatic polyamides and polyimides with adamantylphenoxy-substituted triphenylamine units . European Polymer Journal , 45 : 2234 – 48 .
- Kavitha , AA and Singha , NK . 2009 . Tailor-made poly(methyl acrylate) bearing amantadine functionality (amino adamantyl) via atom transfer radical polymerization (ATRP). A precursor of a supramolecular cross-linked polymer . Macromolecules , 42 : 5499 – 508 .
- Fuchise , K , Sone , M , Miura , Y , Sakai , R , Narumi , A , Sato , SI , Satoh , T and Kakuchi , T . 2010 . Precise synthesis of poly(1-adamantyl methacrylate) by atom transfer radical polymerization . Polymer Journal , 42 : 626 – 31 .
- Matsumoto , A , Tanaka , S and Otsu , T . 1991 . Synthesis and characterization of poly(1-adamantyl methacrylate): effects of the adamantyl group on radical polymerization kinetics and thermal properties of the polymer . Macromolecules , 24 : 4017 – 24 .
- Ishizone , T , Tajima , H , Torimae , H and Nakahama , S . 2002 . Anionic polymerizations of 1-adamantyl methacrylate and 3-methacryloyloxy-1,1′-biadamantane . Macromolecular Chemistry and Physics , 203 : 2375 – 84 .
- Hawker , CJ , Bosman , AW and Harth , E . 2001 . New polymer synthesis by nitroxide mediated living radical polymerizations . Chemical Reviews , 101 : 3661 – 88 .
- Matyjaszewski , K and Xia , JH . 2001 . Atom transfer radical polymerization . Chemical Reviews , 101 : 2921 – 90 .
- Moad , G , Rizzardo , E and Thang , SH . 2009 . Polymerization by the RAFT process – a second update . Australian Journal of Chemistry , 62 : 1402 – 72 .
- Sato , H and Nishio , S . 2001 . Polymer laser photochemistry, ablation, reconstruction, and polymerization . Journal of Photochemistry and Photobiol C Photochemistry Reviews , 2 : 139 – 52 .
- Lippert , T . 2004 . Laser application of polymers . Advances in Polymer Science , 168 : 147 – 215 .
- Naozumi , T and Mitsuhiro , S . 2007 . Synthesis and photocuring of cinnamoyl trehalose esters . Polymers for Advanced Technologies , 18 : 971 – 7 .
- Mahy , R , Bouammali , B , Oulmidi , A , Challioui , A , Derouet , D and Brosse , JC . 2006 . Photosensitive polymers with cinnamate units in the side position of chains: synthesis, monomer reactivity ratios and photoreactivity . European Polymer Journal , 42 : 2389 – 97 .
- Nakayama , Y and Matsuda , T . 1993 . Surface fixation of poly(ethylene glycol) via photodimerization of cinnamate group . Journal of Polymer Science Part A: Polymer Chemistry , 31 : 3299 – 305 .
- Luadthong , C , Tachaprutinun , A and Wanichwecharungruang , SP . 2008 . Synthesis and characterization of micro/nanoparticles of poly(vinylalcohol-co-vinylcinnamate) derivatives . European Polymer Journal , 44 : 1285 – 95 .
- Liu , G , Yan , X , Li , Z , Zhou , J and Duncan , S . 2003 . End coupling of block copolymer nanotubes to nanospheres . Journal of the American Chemical Society , 125 : 14039 – 45 .
- Ding , J , Birss , VI and Liu , G . 1997 . Formation and properties of polystyrene-block-poly(2-cinnamoylethyl methacrylate) brushes studied by surface-enhanced Raman scattering and transmission electron microscopy . Macromolecules , 30 : 1442 – 8 .
- Liu , GJ , Ding , JF , Hashimoto , T , Kimishima , K , Winnik , FM and Nigam , S . 1999 . Thin films with densely, regularly packed nanochannels: preparation, characterization, and applications . Chemistry of Materials , 11 : 2233 – 40 .
- Yan , XH , Liu , GJ , Liu , FT , Tang , BZ , Peng , H , Pakhomov , AB and Wong , CY . 2001 . Superparamagnetic triblock copolymer/Fe2O3 hybrid nanofibers . Angewandte Chemie International Edition , 40 : 3593 – 6 .
- Yan , X , Liu , G and Li , Z . 2004 . Preparation and phase segregation of block copolymer nanotube multiblocks . Journal of the American Chemical Society , 126 : 10059 – 66 .
- Liu , G . 1997 . Nanofibers . Advanced Materials , 9 : 437 – 9 .
- Liu , G , Yan , X , Qiu , X and Li , Z . 2002 . Fractionation and solution properties of PS-b-PCEMA-b-PtBA nanofibers . Macromolecules , 35 : 7742 – 7 .
- Gupta , P , Trenor , SR , Long , TE and Wilkes , GL . 2004 . In stu photo-cross-linking of cinnamate functionalized poly(methyl methacrylate-co-2-hydroxyethyl acrylate) fibers during electrospinning . Macromolecules , 37 : 9211 – 8 .
- Doshi , J and Reneker , DH . 1995 . Electrospinning process and applications of electrospun fibers . Journal of Electrostatics , 35 : 151 – 60 .
- Fong , H , Chun , I and Reneker , DH . 1999 . Beaded nanofibers formed during electrospinning . Polymer , 40 : 4585 – 92 .
- Kim , JS and Reneker , DH . 1999 . Polybenzimidazole nanofiber produced by electrospinning . Polymer Engineering & Science , 39 : 849 – 54 .
- Deitzel , JM , Kleinmeyer , JD , Hirvonen , JK and Beck , TNC . 2001 . Controlled deposition of electrospun poly(ethylene oxide) fibers . Polymer , 42 : 8163 – 70 .
- Wang , X , Lee , SH , Drew , C , Senecal , KJ , Kumar , J and Samuelson , LA . 2001 . Electrospun nanofibrous membranes for optical sensing . Polymeric Materials Science and Engineering , 85 : 617 – 8 .
- Fong , H , Liu , W , Wang , CS and Vaia , RA . 2002 . Generation of electrospun fibers of nylon 6 and nylon 6-montmorillonite nanocomposite . Polymer , 43 : 775 – 80 .
- Deitzel , JM , Kosik , W , McKnight , SH , Tan , NCB , DeSimone , JM and Crette , S . 2002 . Electrospinning of polymer nanofibers with specific surface chemistry . Polymer , 43 : 1025 – 9 .
- Buruiana , EC , Jitaru , F , Buruiana , T and Olaru , N . 2010 . Polycinnamates and block co-polymers prepared by atom transfer radical polymerization and microwave irradiation . Designed Monomers and Polymers , 13 : 167 – 80 .
- Egerton , PL , Pitts , R and Reiser , A . 1981 . Photocycloaddition in solid poly(vinyl cinnamate). The photoreactive polymer matrix as an ensemble of chromophore sites . Macromolecules , 14 : 95 – 100 .
- Sarantopoulou , E , Kollia , Z , Cefalas , AC , Douvas , AM , Chatzichristidi , M , Argitis , P and Kobe , S . 2007 . Nano-scale spatial control over surface morphology of biocompatible fluoropolymers at 157 nm . Materials Science and Engineering C , 27 : 1191 – 6 .
- Sarantopoulou , E , Kollia , Z , Cefalas , AC , Douvas , AM , Chatzichristidi , M , Argitis , P and Kobe , S . 2007 . Polymer self-assembled nano-structures and surface relief gratings induced with laser at 157 nm . Applied Surface Science , 253 : 7884 – 9 .
- Wang , C , Chien , H-S , Yan , K-W , Hung , C-L , Hung , K-L , Tsai , S-J and Jhang , H-J . 2009 . Correlation between processing parameters and microstructure of electrospun poly(D,l-lactic acid) nanofibers . Polymer , 50 : 6100 – 10 .
- Sutasinpromprae , J , Jitjaicham , S , Nithitanakul , M , Meechaisue , C and Supaphol , P . 2006 . Preparation and characterization of ultrafine electrospun polyacrylonitrile fibers and their subsequent pyrolysis to carbon fibers . Polymer International , 55 : 825 – 33 .
- Mit-uppatham , C , Nithitanakul , M and Supaphol , P . 2004 . Ultrafine electrospun polyamide-6 fibers: effect of solution conditions on morphology and average fiber diameter . Macromolecular Chemistry and Physics , 205 : 2327 – 38 .