Abstract
Allyloxy polyethoxy ether (APEG) and succinic anhydride were used to prepare allyloxy polyethoxy carboxylate (APEL). The 8-hydroxy-1,3,6-pyrene trisulfonic acid trisodium salt (PY) was reacted with allyl chloride to produce fluorescent monomer 8-allyloxy-1,3,6-pyrene trisulfonic acid trisodium salt (PA). APEL and PA were copolymerized with acrylic acid (AA) to synthesize PA-tagged no phosphate and nitrogen free calcium phosphate inhibitor AA–APEL–PA. Structures of PA, APEG, APEL, and AA–APEL–PA were carried out by FT-IR and 1H NMR. Different AA:APEL mole ratios were employed for the manufacture of AA–APEL–PA to study the effect of mole ratio on performance of AA–APEL–PA. Relationship between AA–APEL–PA’s fluorescent intensity and its dosage was studied. The results indicate that capability of AA–APEL–PA is heavily depended on the mole ratio of AA:APEL. Correlation coefficient r of AA–APEL–PA’s fluorescent intensity and its dosage is 0.9995, and detection limit of AA–APEL–PA is 0.98 mg/L. AA–APEL–PA can be used to accurately measure polymer consumption on line besides providing excellent calcium phosphate inhibition.
1. Introduction
In industrial recycling water systems, the precipitation of corrosion products is a troublesome issue Citation[1–3]. Phosphonates, such as polyamino polyether methylenephosphonate (PAPEMP), hexa methylene diamine tetra methyl-phosphonic acid (HMDTMP), thiomorpholin-4-ylmethyl-phosphonic acid (TMPA), 1-hydroxy ethylidine-1,1-diphosphonic acid (HEDP), and 2-phosphonobutane-1,2,4-tricarboxylic acid (PBTC) are used to prevent mineral formation and/or metallic corrosion by creating protective films on metal surfaces of industrial equipment Citation[4–10]. However, phosphonates can create serious problems such as insolubility of phosphonate complexes, and they are also susceptible to breakdown to form orthophosphates under the influence of hydrolysis and/or chlorination thereby increasing the potential of formation of calcium phosphate deposits in the presence of excessive amounts of calcium Citation[11–13].
Recently, there is a new project which identified phosphate deposit control agent. It is maleic anhydride (MA)–ammonium allylpolyethoxy sulfate (APES) copolymer. It offers twice as effective relative to all available phosphate inhibitor and it is no phosphate Citation[14].
Unfortunately, it is quite difficult to test for MA–APES by traditional way because there is no phosphate active component in it. Improper feed rate of treating agent leads to serious problems. Another shortcoming of MA–APES is that it still contains nitrogen nutrition. Researches show that N is the key limiting element for the occurrence of algal blooms which leads to great economic loss. In despite of low P level in lake water, N becomes the limited factor of alga blooms when the ratio of N–P in lake water is lower than in the alga Citation[15].
Fluorescence methods provide direct measurement and control of a wide array of treatment actives Citation[16–18]. The concentration of a fluorescent tracer is directly determined from a calibration curve of tracer concentration versus emission. Fluorescent tracer permits the determination of the concentration of scale inhibitor range from parts per million (ppm) to parts per billion (ppb), and its compounds are environmentally acceptable, and are available at low cost.
Presently, fluorophore in fluorescent monomer usually contains benzene ring or heterocyclic, and their structure is rigid planar, so the fluorescence chromophore are hydrophobic. It makes the process of preparation fluorescent-tagged water-soluble copolymer agent more complex. McCormick prepared copolymers of acrylamide (AM) and N-alkylacrylamides by micellar copolymerization in aqueous solution utilizing sodium dodecyl sulfate as a surfactant to solubilize hydrophobic monomer and decrease the adverse effects of solvents Citation[19]. Lei et al. described a fluorescent-tagged water-soluble copolymer [4-methoxy-N-(2-N0,N0-dimethyl aminoethyl) naphthalimide allyl chloride quaternary ammonium salt] (FM)-acrylamide (AM) Citation[20]. They prepared the copolymer by reverse microemulsion because FM is a hydrophobic monomer. Either micellar copolymerization or reverse microemulsion copolymerization requires to at least the procedure of demulsification, lavation, and drying to get the final product and the cost of production is comparatively high. In addition, emulsifier and other impurities in the product are not easily to be removed clearly thus decrease scale inhibition property of copolymer. It is obviously that obtain water-soluble fluorescent monomer is important and aqueous solution polymerization is a better way to prepare fluorescent-tagged copolymer than micellar copolymerization or reverse microemulsion. Moriarty performed 8-allyloxy-1,3,6-pyrene trisulfonic acid trisodium salt (PA) fluorescent monomers. Hydrophilic of PA is strong because of sulfoacid hydrophilic groups Citation[21,22].
On the basis of all these information, the study offers a new calcium phosphate inhibitor. Synthesized allyloxy polyethoxy carboxylate (APEL) by carboxylic acid functionalization of the surface hydroxyl groups and prepared fluorescent-tagged no phosphate and nitrogen free inhibitor acrylic acid (AA)–APEL–PA by free copolymerization. AA–APEL–PA compensates the weaknesses of AA–APES which contain nitrogen and cannot be monitor.
2 Experimental section
2.1 Materials and characterization
The 8-hydroxy-1,3,6-pyrenetrisulfonic acid trisodium salt (pyranine) was purchased from Xuhua Chemical (Shanghai, China). Allyloxy polyethoxy ether (APEG) was purchased from Zhongshan Chemical (Nanjing, Jiangsu, China). Other reagents such as acrylic acid, succinic anhydride, potassium peroxydisulfate, allyl chloride, and ammonium persulfate, of AR grade were obtained from Zhongdong Chemical Reagent (Nanjing, Jiangsu, China). Distilled water was used for all the studies.
Fourier-transform infrared (FT-IR) spectra were taken on a Bruker FT-IR analyzer (VECTOR-22, Bruker Co., Germany) by using the KBr-pellet method (compressed powder). Structures of PA, APEG, APEL, and AA–APEL–PA were also explored by a Bruker NMR analyzer (AVANCE AV-500, Bruker, Switzerland) operating at 500 MHz. Fluorescence measurements were carried out on a luminescence spectrometry (LS-55, Perkin-Elmer, UK) with a xenon lamp as a light.
2.2 Synthesis of APEL and PA
The carboxylic acid functionalization of the surface hydroxyl groups was realized by reaction with succinic anhydride (SA) in acetone Citation[23]. The synthesis procedure of APEL is shown in Scheme .
PA was synthesized according to Moriarty Citation[21]. The product was light yellow power. Synthesis procedure of PA from pyranine and allyl chloride is shown in Scheme .
2.3 Synthesis of AA–APEL–PA
Ninety gram (5 mol) distilled water, 7.2 g (0.1 mol) AA, and 16 g (0.03 mol) APEL (the mole ratio of AA and APEL was 3:1) were mixed together in a 250 mL five-neck round-bottom flask fitted with a thermometer and a magnetic stirrer. The mixture was heated to 70 °C with stirring under nitrogen atmosphere. Around 0.66 g, 1 mmol of AP (the amount of AP in the tagged copolymers is 2.5 wt%) in 20 g distilled water, 1.0 g ammonium persulfate in 20 g distilled water and 1.0 g sodium metabisulfite in 20 g distilled water were added dropwise in the 250 mL round-bottom flask over a period of 1.0 h at 70 °C. And then, the reactant was heated with stirring at 80 °C for 1.5 h under nitrogen atmosphere. The mixture was subsequently cooled and the polymer was then isolated by successive precipitations in a large volume of acetone. The insoluble product was filtered, collected, and extracted in a soxhlet extractor for 16.0 h to remove the unused AA and APEL. The crude product was dried in vacuum oven until constant weight, and re-crystallized from water–acetone mixture (3:7 V/V) to remove the residual PA and gain AA–APEL–PA as a white solid. The synthesis procedure of AA–APEL–PA from AA, APEL, and PA is shown in Scheme .
2.4 Synthesis of AA–APEL–PA at different AA:APEL mole ratio
AA–APEL–PA was radical polymerized at feed AA:APEL mole ratios of 3:2, 1:1, 2:3, and 1:3 to study the effect of different mole ratio of AA:APEL on AA–APEL–PA’s phosphoric inhibition. The process was the same as initial feed mole ratio of AA: APEL was 3:1 in synthesis of AA–APEL–PA while kept the usage of AP at 2.5 wt% in AA–APEL–AP.
2.5 Precipitation Conditions
All precipitation experiments were carried out in flask tests and all inhibitors dosages given below are on a dry-inhibitor basis. Analytical reagents and distilled water were used throughout. The precipitation experiments were done under the conditions of 250 mg/L Ca2+, 5 mg/L PO4 3−, pH = 9.0, T = 80 °C, t = 10 h according to the national standard of P.R. China concerning the code for the design of industrial recirculating cooling-water treatment (GB 50,050–95). Inhibitor efficiency as a calcium phosphate inhibitor was calculated by using the following equation:
where [phosphate]final = concentration of phosphate in the filtrate in the presence of inhibitors at 10 h, [phosphate]blank = concentration of phosphate in the filtrate in the absence of inhibitors at 10 h, and [phosphate]initial = concentration of phosphate at the beginning of the experiment.
2.6 Excitation and emission wavelength measurement of AP and AA–APEL–PA
Excitation and emission wavelengths of PA and AA–APEL–PA were all measured at β ex = 402 nm (10 nm slid width) and β em = 430 nm (5 nm slid width), respectively. The excitation and emission wavelengths were chose the same as the excitation and emission wavelength of pyranine. The 5 × 10−8 mol/L PA distilled water solution was prepared and AA–APEL–PA was dissolve in quantum sufficient distilled water and concentration of PA in AA–APEL–PA solution was also 5 × 10−8 mol/L.
2.7 Detection of AA–APEL–PA fluorescent intensity with different concentration
Use of inert fluorescent tracers and online fluorometer provides accurate control of treatment dosage and immediate response to changes in treatment dosage. Fluorescent light is emitted that ought to directly proportional to the dosage of treatment in the water, which translates into reliable control of treatment dosage. A serial concentration of AAAPEL–AP sample should reflect a corresponding serial of the fluorescence intensity. Prepared for 2, 4, 6, 8, 10, 12, 14, 16, 18, and 20 mg/L AA–APEL–PA aqueous solution samples which has the best mole ratio of AA:APEL in AA–APEL–PA. Estimated AAAPEL–AP fluorescent intensity respond to their concentration.
3 Results and discussion
3.1 FT-IR measurements
The FT-IR spectra of PA are exhibited in Figure . PA (FT-IR, cm−1): 660–880 (C–H plane deforAAtion vibration of aroAAtic compound), 1008 (C–H plane deformation vibration of C = H2), 1047 (alkyl oxide characteristic absorption of PA), 1276 (fragrant ether characteristic absorption), 1450–1630 (aromatic compound absorption band), 1657 (C=C stretching vibration), and 3449 (O–H stretching vibration).
The characteristic vibration bands of 1276 cm−1 are the fragrant ether characteristic absorption, the alkyl oxide characteristic absorption of PA shows an intense peak at 1047 cm−1. Presently, two bands both exist prove the monomer structure includes alkyl aryl ether Citation[24]. The C=C stretching shows a sharp intense peak at 1657 cm−1. The FT-IR proves that the synthesized monomer has the anticipated structure.
The FT-IR spectra of APEG, APEL, and AA–APEL are exhibited in Figure . The 1736 cm−1 strong intensity absorption peak (–C = O) in curve b reveals clearly that APEL has been synthesized successfully. The fact that the (–C = C–) stretching vibration at 1646 cm−1 appears in curve b but disappears completely in curve c reveals that free radical polymerization between AA, APEL, and PA has happened.
3.2 1H NMR studies
Figure is 1H NMR of PY (a) and PA (b), PY ((CD3)2SO, δ ppm): 2.40–2.60 (solvent residual peak of (CD3)2SO), 8.10–9.15 (six protons of benzene ring in PY), 10.63 (–OH, active hydrogen in PY) (Figure ). PA ((CD3)2SO, δ ppm): 2.40–2.60 (solvent residual peak of (CD3)2SO), 4.97–6.33 (CH2=CH–CH2–, propenyl protons), 8.10–9.15 (six protons of benzene ring in PA). (Figure ). PA has two different types of protons, the propenyl and benzene ring protons corresponding position in two absorption peaks, and the figure shows the integral structure consistent with the area. It certifies that the product has expected structure.
APEG ((CD3)2SO, δ ppm): 2.50 (solvent residual peak of (CD3)2SO), 3.00–3.80 (–OCH2CH2–, ether groups), 3.80–6.00 (CH2=CH–CH2–, propenyl protons), 4.40–4.60 (–OH, active hydrogen in APEG) (Figure ).
APEL ((CD3)2SO, δ ppm): 2.25–2.55 (–CH2CH2–, protons in –COCH2CH2COOH), 2.50 (solvent residual peak of (CD3)2SO), 3.00–3.80 (–OCH2CH2–, ether groups), 3.80–4.10 and 5.00–6.00 (CH2 = CH–CH2–, propenyl protons) (Figure ).
The δ 4.40–4.60 ppm (–OH) active hydrogen in (a) disappeared completely and (–CH2CH2–) protons in –COCH2CH2COOH appeared obviously in δ 2.25–2.55 ppm in (b). It proves that –OH in APEG has been entirely replaced by –COCH2CH2COOH.
AA–APEL–PA ((CD3)2SO, δ ppm): 2.50 (solvent residual peak of (CD3)2SO), 3.00–3.80 (–OCH2CH2–, ether groups), and 8.10–9.15 (six protons of benzene ring in PA). (Figure ).
The δ 3.80–6.00 ppm in (b) double bond absorption peaks completely disappeared in (c). This reveals that free radical polymerization among APEL, AA, and PA has happened. From FT-IR and 1H NMR analysis, it can conclude that synthesized AA–APEL–PA has anticipated structure.
3.3 Calcium phosphate inhibition of AA–APEL–PA at different mole ratio of AA:APEL
The properties of AA–APEL–PA (6 mg/L) prepared at different AA:APEL mole ratio is summarized in Figure. . The results demonstrated that mole ratio has large impact on the properties of polymers. Conversion rate of the reaction will be different when monomer ratio of AA:APEL is different, thus affects chain sequence structure and molecular structure of the macromolecular polymer. Copolymers can have very different properties with the same composition but different sequence structure. At the same time, the proportions of functional groups in the long-chain polymer macromolecules are also different. Polymers have good scale performance only when the proportions of functional groups in long-chain macromolecules are appropriate. On the other hand, there is a best molecular weight for calcium phosphate scale inhibition. Monomer ratio changes of AA:APEL in AA–APEL–PA have relative impact on their molecular weight. The calcium phosphate inhibition testing results manifest that AA–APEL–PA which mole ratio of AA:APEL is 1:1 show superior efficacy in calcium phosphate inhibition than other mole ratio.
3.4 Influence of AA–APEL–PA dosage on Ca3(PO4)2 inhibition
The dosage of AA–APEL–PA copolymer has a strong effect on the formation of calcium phosphate precipitation, which was investigated and shown in Figure. . When the copolymer concentration changes, the inhibition effect also changes correspondingly, scale inhibition effect increases with increasing the copolymer concentration. There is a sudden increase of scale inhibition as the concentration increases from 2 to 6 mg/L, which reaches 15.7% using 2 mg/L, 98.3% for 6 mg/L, and moderately higher by further increasing the concentrations from 6 to 20 mg/L.
3.5 Influence of solution property on calcium phosphate inhibition
Solution properties have a great influence on the precipitation of calcium phosphate. In order to optimize parameters of the recycling water process on an industrial scale, we investigated the effect of the solution parameters on the calcium phosphate inhibition of AA–APEL–PA copolymer. The results are shown in Figure as follows:
Figure 7 Inhibition at a level of 6 mg/L AA–APEL–PA as a function of solution Ca2+ concentration (a), temperature (b), pH (c), and Fe2+ concentration (d).
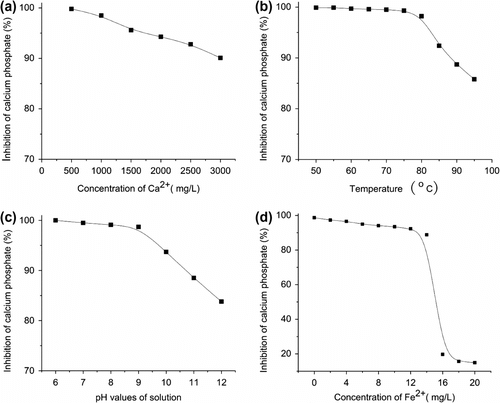
Figure indicates AA–APEL–PA which provides unexceptionable calcium phosphate inhibition under conditions of water with a much higher hardness. Figure clearly demonstrates that the AA–APEL–PA copolymers have good thermal stability. When increasing the solution temperature from 50 to 95 °C, AA–APEL–PA retains most of its activity, only 12% loses in calcium phosphate inhibition. As illustrated in Figure , calcium phosphate inhibitory power drops 16% with increasing the solution pH from 6 to 12. The reason is probably that the solubility of calcium phosphate decreases when increasing the pH. At a pH of 8.8–9.2, the usual pH values of the industry recycling water, AA–APEL–PA still shows superior calcium phosphate inhibition. Thus, the incorporation of the high performance scale inhibitor AA–APEL–PA into recycling water ensures a better overall system performance.
In consideration of the favorable reaction with iron ions, some scale inhibitors, such as homopolymer of maleic acid, would lose most of their effectiveness against calcium phosphate scale in the presence of trace amounts of iron in solutions. The results in Figure show AA–APEL–PA still has excellent calcium phosphate inhibition at levels of 0–14 mg/L iron ions in supersaturated solutions of calcium phosphate. However, the calcium phosphate inhibition of AA–APEL–PA decreases dramatically at levels of 14–16 mg/L iron ions, and AA–APEL–PA is totally ineffective against the calcium phosphate scale when the concentrations of iron ions in solutions are above 16 mg/L. The trace amounts of iron are usually on the order of 1–5 mg/L in industrial recycling water systems, hence, the copolymer of AA–APEL–PA is still an excellent scale inhibitor for calcium phosphate inhibition, even in the presence of trace amounts iron ions in aqueous solutions.
3.6 The mechanism of calcium phosphate inhibition
AA–APEL–PA have high molecular weight, and the (–COOH) anionic active groups are at the lateral and exposed. They have large extension in water because of electrostatic repulsion and chelate calcium ion. Next to chelate calcium ion, they adsorb in calcium phosphate micro-crystal particles surface and then increase negative charge density of the particles. It makes the electrostatic repulsion force increasing between particles thus prevents grow up of calcium phosphate micro-crystal particles. Furthermore, copolymer particles can be embedded in the lattice of calcium phosphate micro-crystal particles. Particles of AA–APEL–PA and calcium phosphate micro-crystal particles have different sizes. This enables AA–APEL–PA particles set in calcium phosphate crystal and prevent calcium phosphate micro-crystal growth in the lattice. In addition, ether structure and (–COOH) functional groups of AA–APEL–PA are similar to some anionic surfactants. Only one hydrophilic group needed to perform hydrophilicity. (–COOH) are water soluble functional groups, the oxygen atoms in ether bond formed weak hydrogen bonds with hydrogen atoms in water. Strong hydrophilic makes AA–APEL–PA attach to one end or adsorbed on all calcium phosphate micro-crystal particles surface so both proved to be quite robust and superior efficiency in calcium phosphate inhibition.
3.7 Excitation and emission properties of PA and AA–APEL–PA
Excitation and emission wavelength of PA (a) and AA–APEL–PA (b) are represented in Figure . It can be achieved by the figure that excitation and emission wavelengths of PA and AA–APEL–PA are also 402 and 430 nm. Chromophore of PA and AA–APEL–PA are all pyranine so AA–APEL–PA and PA have the same excitation and emission wavelengths as pyranine. Excitation spectra and emission spectra of AA–APEL–PA show good mirror-image relationship as PA. It demonstrates that molecule structure of fluorescent configuration changed a little from monomer to copolymer.
The fluorescence intensity of AA–APEL–PA increased compared with AP after copolymerization because of the form of hydrogen bond. The lowest singlet excited states of aroAAtic carbonyl compounds such as AP is (n, p∗). The excited state possess (n, p∗) character in nonpolar and weakly hydrogen bonding solvents but they exhibits enhanced (p∗, p∗) character in very polar hydrogen bonding solvents. (p∗, p∗) states are, or become, the energetically lowest states after copolymerization. The yields of fluorescence grow in quantity in (p∗,p∗) states than in (n, p∗) states Citation[25,26].
3.8 Response of fluorescent intensity over a range of AA–APEL–PA
Result of linearity testing between AA–APEL–PA fluorescence intensity and their concentration is shown in Figure . The relationship between AA–APEL–PA concentration and fluorescence intensity provided exceptionally linear response (correlation coefficient r = 0.9995, where perfect line = 1.0000). This positive linear relationship can be used to quantitatively measure the concentration of AA–APEL–PA. The dosage change of AA–APEL–PA is pointed out by the fluorescence spectra of AA–APEL–PA. This optical technique is able to characterize early corrosion and to quantify corrosion defects. The detection limit of AA–APEL–PA is 0.98 mg/L according to the detection limit formula: Dr = 3σ/k, where σ is 11 times determination of blank solution’s standard deviation and k is slope of calibration curve.
4 Conclusions
Water-soluble monomer APEL was produced from APEG and succinic anhydride by carboxylic acid functionalization method. Fluorescent monomer PA was prepared from pyranine and allyl chloride. Structures of APEG, APEL, and PA were identified by FT-IR and 1H-NMR. Fluorescent-tagged no phosphate and nitrogen free calcium phosphate inhibitor AA–APEL–PA was successfully synthesized by free polymerization of AA, APEL, and PA. FT-IR and 1H NMR identified that AA–APEL–PA has the expected structures. The most suitable of AA:APEL in AA–APEL–PA for its calcium phosphate inhibition is 1:1 by investigation of the effects of AA:APEL mole ratio on AA–APEL–PA performance. The dosage of AA–APEL–PA copolymer has a strong effect on the formation of calcium phosphate precipitation. AA–APEL–PA maintains most of its calcium phosphate inhibition under the conditions of solution pH 6–12, temperature 50–95 °C, calcium hardness 500–3000 mg/L, and at levels of 0–14 mg/L iron ions in aqueous solutions.
Excitation and emission spectra of PA and AA–APEL–PA have been detected readily with a fluorospectrophotometer and they show good mirror symmetry relations. AA–APEL–PA detection limit is 0.98 mg/L according to the detection limit formula. Good relationship between AA–APEL–PA fluorescent intensity and its dosage (the correlation coefficient r = 0.9995) ensures that AA–APEL–PA is a valuable indicator for cooling-water system performance. The new active polymer test method provides an easy, quick, and accurate method to determine polymer consumption.
Acknowledgments
This work was supported by the Prospective Joint Research Project of Jiangsu Province (BY2012196); the National Natural Science Foundation of China (51077013); Special funds for Jiangsu Province Scientific and Technological Achievements Projects of China (BA2011086); Program for Training of 333 High-Level Talent, Jiangsu Province of China (BRA2010033); Scientific Innovation Research Foundation of College Graduate in Jiangsu Province (CXLX-0134); and The Scientific Research Foundation of Graduate of Southeast University (YBJJ1110).
References
- Belton , D and Stupp , SI . 1983 . Adsorption of ionizable polymers on ionic surfaces: poly(acrylic acid) . Macromolecules , 16 : 1143 – 50 .
- Nesrin , OZ and Ahmet , AK . 2000 . Low molecular weight polyacrylic acid with pendant aminomethylene phosphonic acid groups . Journal of Applied Polymer Science , 78 : 870 – 4 .
- Doherty , WOS , Fellows , CM , Gorjian , S , Senogles , E and Cheung , WH . 2004 . Inhibition of calcium oxalate monohydrate by poly(acrylic acid)s with different end groups . Journal of Applied Polymer Science , 91 : 2035 – 41 .
- Zang , DM , Cao , DK and Zheng , L . 2011 . Strontium and barium 4-carboxylphenylphosphonates: hybrid anti-corrosion coatings for magnesium alloy . Inorganic Chemistry Communications , 14 : 1920 – 3 .
- Khodyrev , YP , Batyeva , ES , Badeeva , EK , Platova , EV , Tiwari , L and Sinyashin , OG . 2011 . The inhibition action of ammonium salts of O,O′-dialkyldithiophosphoric acid on carbon dioxide corrosion of mild steel . Corrosion Science , 53 : 976 – 83 .
- Amara , H , Braisaz , T , Villemin , D and Moreau , B . 2008 . Thiomorpholin-4-ylmethyl-phosphonic acid and morpholin-4-methyl-phosphonic acid as corrosion inhibitors for carbon steel in natural seawater . Materials Chemistry and Physics , 110 : 1 – 6 .
- Laamari , R , Benzakour , J , Berrekhis , F , Abouelfida , A , Derja , A and Villemin , D . 2011 . Corrosion inhibition of carbon steel in hydrochloric acid 0.5 M by hexa methylene diamine tetramethyl-phosphonic acid . Arabian Journal of Chemistry , 4 : 271 – 7 .
- Demadis , KD , Katarachia , SD and Koutmos , M . 2005 . Crystal growth and characterization of zinc-(amino-tris-(methylenephosphonate)) organic-inorganic hybrid networks and their inhibiting effect on metallic corrosion . Inorganic Chemistry , 8 : 254 – 8 .
- Sathiyanarayanan , S , Syed Azim , S and Venkatachari , G . 2008 . Performance studies of phosphate-doped polyaniline containing paint coating for corrosion protection of aluminium alloy . Journal of Applied Polymer Science , 107 : 2224 – 30 .
- Tomson , MB and Nancollas , GH . 1978 . Mineralization kinetics: a constant composition approach . Science , 200 : 1059 – 60 .
- Tsortos , A and Nancollas , GH . 2002 . The role of polycarboxylic acids in calcium phosphate mineralization . Journal of Colloid and Interface Science , 250 : 159 – 67 .
- Amjad , Z . 1989 . Constant-composition study of dicalcium phosphate dihydrate crystal-growth in the presence of poly(acrylic acids) . Langmuir , 5 : 1222 – 5 .
- Zhang , BR , Zhang , L , Li , FT , Hu , W and Hannam , PM . 2010 . Testing the formation of Ca-phosphonate precipitates and evaluating the anionic polymers as Ca-phosphonate precipitates and CaCO3 scale inhibitor in simulated cooling water . Corrosion Science , 52 : 3883 – 90 .
- Kessler , SM . 2003 . Analyses of the performance of a next generation phosphate inhibitor for industrial water application . Hydrocarbon Engineering , 8 : 66 – 72 .
- White , E and Payneg , WNZ . 1978 . Chlorophyll production, in response to nutrient additions, by the algae in Lake Rotorua water . Journal of Marine and Freshwater Research , 12 : 131 – 8 .
- Gatti , R and Lotti , C . 2011 . Development and validation of a pre-column reversed phase liquid chromatographic method with fluorescence detection for the determination of primary phenethylamines in dietary supplements and phytoextracts . Journal of Chromatography A , 1218 : 4468 – 73 .
- Li , C and Song , G . 2009 . Photocatalytic degradation of organic pollutants and detection of chemical oxygen demand by fluorescence methods . Sensors and Actuators, B: Chemical Sensors and Materials , 137 : 432 – 6 .
- Crespo-Sempere , A , López-Pérez , M , Martínez-Culebras , PV and González-Can delas , L . 2011 . Development of a green fluorescent tagged strain of aspergillus carbonarius to monitor fungal colonization in grapes . International Journal of Food Microbiology , 148 : 135 – 40 .
- McCormick , CL , Nonaka , T and Johnson , CB . 1988 . Water-soluble copolymers. 27. Synthesis and aqueous-solution behavior of associative acrylamide N-alkylacrylamide copolymers . Polymer , 29 : 731 – 9 .
- Lei , W , Wang , FH , Xia , MZ , Lu , LD and Wang , FY . 2006 . Properties of fluorescent polymer F-PAM prepared by reverse microemulsion polymerization . Journal of Chemical Industry and Engineering (China) , 57 : 2464 – 8 .
- Moriarty BE, Hoots JE, Workman DP and Rasimas JP. Fluorescent monomers and polymers containing same for use in industrial water systems. Patent US 6,312,644. 2001.
- Dai , Y , Jian , XG , Liu , XM and Guiver , MD . 2001 . Synthesis and characterization of sulfonated poly(phthalazinone ether sulfone ketone) for ultrafiltration and nanofiltration membranes . Journal of Applied Polymer Science , 79 : 1685 – 92 .
- Meng , QW , Chen , DZ , Yue , LW , Fang , JL , Zhao , H and Wang , LL . 2007 . Hyperbranched polyesters with carboxylic or sulfonic acid functional groups for crystallization modification of calcium carbonate . Macromolecular Chemistry and Physics , 208 : 474 – 84 .
- Xia , D.W. and Zhang , Z.X. 1990 . Analysis of Polymer , Beijing : Chemical Industry Press . p. 52–3
- Hiroaki , B , Goodman , L and Valenti , PC . 1966 . Solvent effects on the fluorescence spectra of diazines. Dipole moments in the (n,π∗) excited states . Journal of the American Chemical Society , 88 : 5410 – 5 .
- Rusakowicz , R , Byers , GW and Leermakers , PA . 1971 . Electronically excited aromatic carbonyl compounds in hydrogen bonding and acidic media . Journal of the American Chemical Society , 93 : 3263 – 6 .