Abstract
In this study, the poly(3-hydroxybutyrate)/multi-walled carbon nanotubes (PHB/MWNTs) nanocomposite was prepared by means of a melt blending method. To improve the compatibility/dispersability of MWNTs within the PHB matrix, the acrylic acid grafted poly(3-hydroxybutyrate) (PHB-g-AA) and the multihydroxyl functionalized MWNTs (MWNTs-OH) were used as alternatives. Based on the results of TEM micrographs, FTIR spectra, 13C solid-state NMR spectra, XRD patterns, and thermal and mechanical characterizations, the PHB-g-AA/MWNTs-OH blend demonstrated dramatic enhancement in thermal and mechanical properties of PHB due to the formation of ester carbonyl groups through the reaction between carboxylic acid groups of PHB-g-AA and hydroxyl groups of MWNTs-OH. For example, with an addition of 1 wt.% MWNTs-OH, the increases in the initial decomposition temperature and the tensile strength at break were 75 °C and 15.1 MPa, respectively. Finally, the optimal amount of MWNTs-OH was 1 wt.% because excess MWNTs-OH caused separation of the organic and inorganic phases and reduced their compatibility.
1. Introduction
To address the increasing public awareness over the environmental hazards caused by the disposal of petroleum-based plastics, development and utilization of biodegradable polymeric materials [e.g. polylactic acid, poly(ϵ-caprolactone) (PCL), poly(3-hydroxybutyrate) (PHB), and thermoplastic proteins] are the important issues. The PHB, whose mechanical properties are similar to those of isotactic polypropylene, has attracted an industrial attention as environmentally degradable plastics for a wide range of agricultural, marine, and medical applications because it is 100% biodegradable and it can be produced from renewable resource Citation[1,2]. Besides the higher cost as compared to petroleum-based plastics, the main drawbacks of PHB are its brittleness and thermal instability. To overcome these problems, some attempts have been extensively made to modify the physical properties of PHB, to improve the processability and to lower the cost by blending with other polymers such as poly(ethylene oxide), poly(vinyl alcohol), PCL, cellulose esters, and starch Citation[3–7].
In the past decades, the inorganic minerals, such as montmorillonite and hectrite, had been widely used as reinforcement materials for polymers due to their nanoscale size and intercalation/exfoliation properties Citation[8–10]. At this scale, the inorganic fillers can improve dramatically the physical and mechanical macroscopic properties of polymer even though their amount was small. Since the discovery of carbon nanotubes (CNTs) by Iijima in 1991 Citation[11], extensive researches had been devoted to use carbon nanotubes as inorganic fillers to improve the performance of polymers or to achieve new desired properties Citation[12–16]. However, to obtain uniform dispersion of carbon nanotubes in the polymer matrix is very difficult due to the insolubility of carbon nanotubes and the inherently poor compatibility between the two phases, and the resulting inhomogeneous nanocomposites often have unsatisfying properties. Consequently, some methods must be proposed to functionalize carbon nanotubes (i.e. anchoring the matrix polymer on the carbon nanotubes) before using them as reinforcements for the polymer matrix. Among those methods, oxidatively generating carboxylic acid groups on the surface of the carbon nanotubes and then covalently linking oligomers or polymers with these carboxylic acid groups is an effective skill to improve the compatibility of hybrids Citation[17–24]. Generally, the linkage of small or large molecules to the CNTs by covalent methods is more stable and effective than the noncovalent ones. So, the covalent methods, by which carboxylic acid groups formed at the ends and the defect sites of CNTs, were commonly used for the precursor functionalization in the formation of ester, and organometallic structures Citation[25,26].
Though the addition of multi-walled carbon nanotubes (MWNTs) as reinforced fillers to PHB has been studied Citation[27], the acrylic acid grafted poly(3-hydroxybutyrate) (PHB-g-AA) was used as an alternative to PHB to enhance the compatibility/dispersability of MWNTs within the PHB matrix in this research. The resulting composites (poly(3-hydroxybutyrate)/multi-walled carbon nanotubes [PHB/MWNTs] and PHB-g-AA/MWNTs-OH) were then thoroughly characterized with a Fourier transform infrared (FTIR) spectroscopy, a 13C solid-state NMR spectroscopy, an X-ray diffractometer (XRD), a differential scanning calorimetry (DSC), a thermogravimetry analyzer (TGA), a transmission electron microscopy (TEM), and a tensile testing machine.
2 Experimental
2.1 Materials
The MWNTs (purity > 95%, diameter = 40–60 nm), produced via chemical vapor deposition, were purchased from Seasunnano Pro. Co. (China). Sulfuric acid (96%), nitric acid (61%), thionyl chloride, 1,3-propanediol, poly(3-hydroxybutyrate), acrylic acid (AA), and benzoyl peroxide (BPO) were obtained from Aldrich Chemical Corporation. (Milwaukee, WI, USA). AA was purified before use by recrystallization from chloroform and BPO was purified by dissolving in chloroform and reprecipitating with methanol. According to the procedures described in our previous work Citation[28], the PHB-g-AA copolymer was prepared in our laboratory and its grafting percentage was determined as being about 5.60 wt.% when BPO loading and AA loading were kept at 0.3 and 10 wt.%, respectively.
2.2 Preparation of MWNTs-OH and hybrids
The synthesis route of MWNTs-OH, PHB-g-AA/MWNTs-OH, and PHB/MWNTs is outlined in Scheme . About 500 mg crude MWNTs were stirred in 30 ml of a H2SO4/HNO3 (1:3) mixture to generate carboxyls onto them, and then the attached carboxyls were converted to carbonyl chloride groups via the reaction with thionyl chloride. After the reaction was completed, the unreacted SOCl2 was evaporated with a rotary evaporator, and the collected acyl chloride functionalized MWNTs (MWNTs-COCl) were kept away from air and dried in a vacuum oven overnight. Finally, MWNTs-COCl reacted with 1,3-propanediol to produce the multihydroxyl functionalized MWNTs (MWNTs-OH). The resulting solid (MWNTs-OH) was separated by vacuum filtration using the 0.22 m Millipore polycarbonate membrane filter and subsequently washed with anhydrous xylene. After repeated washing and filtration, the MWNTs-OH was dried overnight in a vacuum oven.
All the hybrids were prepared using a Brabender ‘Plastograph’ 200 Nm W50EHT mixer with a blade type rotor. Prior to blending, MWNTs and MWNTs-OH were dried in a vacuum oven at 60 °C for 2 days. The contents of MWNTs or MWNTs-OH in hybrids (PHB/MWNTs or PHB-g-AA/MWNTs-OH) were set at 0, 0.5, 1, 2, and 3 wt.%. About 20 g of mixture (polymer and filler) was put into the mixer for 15 min to obtain complete mixing under conditions that the rotor speed and the blending temperature were set at 50 rpm and 190 °C, respectively. For the preparation of PHB-g-AA/MWNTs-OH hybrids, the blended mixture was further put into a vacuum oven at 100 °C for 8 h to proceed the esterification reaction between PHB-g-AA and MWNTs-OH. Finally, the hybrids were pressed into 1 mm-thick plate using a hydraulic press at 190 °C and 100 atm, and then put into a dryer for cooling. The cooled plates were made into standard specimens for further characterizations. Before characterization of samples, the specimens were conditioned at a relative humidity of 50 ± 5% for 24 h in the desiccator.
2.3 Characterizations of hybrids
FTIR measurements were performed on a BIO-RAD FTS-7PC FT-IR spectrometry ranging from 500 to 4000 cm−1. For the FTIR tests, the sample was ground into fine powders by the milling machine and then it was coated in a KBr plate. The FTIR spectra thus obtained were used to investigate the grafting reaction of acrylic acid onto PHB and to verify the incorporation of a MWNTs-OH phase to the extent that ester carbonyl bonds were formed in hybrids. To further confirm the result obtained from FTIR analysis, the solid-state 13C NMR analysis was performed using a Bruker AMX 400 13C NMR spectrometer at the condition of 50 MHz. The NMR spectra were observed under cross-polarization; magic antic angle sample spinning and power decoupling conditions with 90° pulse, and 4 s cycle time. The XRD intensity curves were recorded on the Rigaku D/max 3 V X-ray diffractometer (Tokyo, Japan), using a Cu target and Kα radiation (λ = 0.1541 nm) with a scanning speed of 1 deg min−1 at 30 kV and 15 mA, to study changes in crystalline structure. The glass transition temperature (T g), the melting temperature (T m), and the melting enthalpy (ΔH m) of samples were determined from a TA Instrument 2010 DSC system (New Castle, DE, USA). For DSC tests, sample sizes ranged from 4 to 6 mg and the melting curves were obtained at a temperature range of −30 to 250 °C, scanned at a heating rate of 10 °C/min. The thermogravimetry analyzer (TA Instrument 2010 TGA, New Castle, DE, USA) was used to assess whether organic–inorganic phase interactions influenced thermal degradation of hybrids. Samples were placed in alumina crucibles and tested with a thermal ramp over the temperature range of 30–500 °C at a heating rate of 20 °C/min, and then the initial decomposition temperatures (IDT) of hybrids were obtained from the shoulder of the mass loss (TG%) curve. A micrograph of a microtome section of the hybrids of 60–100 nm thickness, mounted in epoxy resin, was conducted with a TEM (JEM-100CX Ⅱ, Jeol Company, Japan) at an acceleration voltage of 100 kV. According to the ASTM D638 method, the tensile testing machine (Model Lloyd, LR5 K type, Segensworth, Fareham, UK) was used to measure the tensile stress and strain using a 20 mm/min crosshead speed. Five measurements of mechanical properties were performed for each sample and the results were averaged to obtain a mean value.
3 Results and discussion
3.1 FTIR analysis
Figure illustrates the FTIR spectra of MWNTs, MWNTs-COOH, and MWNTs-OH in the range of 1000–4000 cm −1. Figure shows that the characteristic peaks of MWNTs appear at 1350 cm−1 (C−O), 1450–1600 cm−1 (aromatic ring), 1641 cm−1 (C=C), and 3390 cm−1 (−OH). As compared with MWNTs, the FTIR spectrum of MWNTs-COOH (Figure ) gives two extra peaks at around 1180 cm−1 and 1721 cm−1, coming from the stretching vibration of C=O and C−O groups in the carboxyl group (−COOH), respectively. This result is in agreement with the findings of Huang et al. Citation[29] and indicates that long ropes of MWNTs are cleaved into short open-ended pipes and that their tube ends and sidewalls are also covered with the oxygen-containing functional groups, such as carboxyls (−COOH), carbonyls (−C=O), and hydroxyls (−OH) after treatment of chemical oxidation Citation[24]. For the MWNTs-OH, Figure shows that the peaks arise from the stretching vibration of C=O and –C−O bonds of ester groups are shifted from 1721 cm−1 and 1180 cm−1 to 1736 cm−1 and 1200 cm−1, respectively, as compared with the MWNTs-COOH. This observation confirms that −COCl group of MWNTs-COCl can react with 1,3-propanediol to form MWNTs-OH Citation[30,31]. It is also found that the hydroxyl-stretching band appears as a strong broad band at 3438 cm−1 for the MWNTs-COOH but it is observed at 3419 cm−1 for the MWNTs-OH. This region at 3200–3500 cm−1 is therefore identified as being representative of nonhydrogen bonded hydroxyl groups (labeled as isolated or free hydroxyl groups).
It can be seen from Figure that characteristic peaks of PHB at 3300–3700, 1700–1760, and 500–1500 cm−1 all appear in neat PHB and PHB-g-AA Citation[32,33], but an extra peak at 1710 cm−1 assigned to −C=O as well as a broad O−H stretching absorbance centered at 3265 cm−1 are observed for the modified PHB. Similar results have been reported elsewhere Citation[34,35]. This result demonstrates that acrylic acid had been grafted onto PHB because the discernible shoulder near 1710 cm−1 is a product of free acid from the modified polymer. For the PHB-g-AA/MWNTs hybrid (Figure ), the characteristic peaks of PHB-g-AA and MWNTs are nearly unchanged, revealing that MWNTs can only be dispersed physically in the polymer matrix. As compared with Figure , due to the formation of ester carbonyl groups through the reaction between PHB-g-AA and MWNTs-OH, Figure shows that the peak at 1710 cm−1 and the hydroxyl-stretching band at 3265 cm−1 for PHB-g-AA are shifted to 1739 and 3216 cm−1, respectively, when it is blended with MWNTs-OH. As compared with PHB-g-AA, the FTIR spectrum of the MWNT–CO-O-(CH2)3-O-OC-PHB hybrid (Figure ) shows that the peak at 1710 cm−1 in Figure was shifted to 1739 cm−1, which can be assigned to the ester carbonyl stretching vibration in the copolymer Citation[36]. The shift of absorption peak from 1710 to 1739 cm−1 is due to the formation of ester carbonyl functional groups through the reaction between carboxylic acid groups of PHB-g-AA and hydroxyl groups of MWNTs-OH. In addition, the hydroxyl-stretching band appears as a strong broad band at 3265 cm−1 for the PHB-g-AA copolymer (Figure ), but this absorbance is shifted to 3216 cm−1 for PHB-g-AA/MWNTs-OH hybrid (Figure ). The reason for this shift in wave number is the presence of H2O formed from esterification of PHB-g-AA and MWNTs-OH.
3.2 NMR analysis
To further confirm the grafting of AA onto PHB and to investigate compatibility between PHB-g-AA and MWNTs-OH, the 13C NMR spectra (Figure ) were used to examine the structure of neat PHB, PHB-g-AA, and PHB-g-AA/MWNTs-OH. Figure shows that carbon peaks appear in four places for the neat PHB (4: δ = 19.96 ppm; 3: δ = 67.46 ppm; 2: δ = 40.96 ppm; and 1: δ = 170.06 ppm). This result was similar to that reported by Doi et al. Citation[37] in which the 3-hydroxybutyrate and 4-hydroxybutyrate co-polyester was studied. A comparison of signals of PHB and PHB-g-AA, Figure and 3(B), reveals that three extra peaks (Cβ: δ = 35.63 ppm; Cα: δ = 42.19 ppm; and a: δ = 175.05 ppm) could be observed in the latter. The extra peaks, Cα, Cβ, and ‘a,’ do indeed confirm that AA has been grafted onto PHB. Additionally, as compared with the PHB-g-AA copolymer, it can be seen from Figure that extra peaks at δ = 120–125 ppm (aromatic carbon of MWNTs) and δ = 177.35 ppm appear in the spectrum of the PHB-g-AA/MWNTs-OH hybrid. The new peak ‘b’ at δ = 177.35 ppm is due to the reaction between –COOH groups of PHB-g-AA and –OH groups of MWNTs-OH. This provides the further evidence of formation of ester carbonyl groups via the condensation reaction between PHB-g-AA and MWNTs-OH. Formation of ester carbonyl functional groups has a profound effect on thermal and mechanical properties, something that will be discussed in the following sections.
3.3 XRD analysis
The crystallization properties of neat PHB, PHB/MWNTs and PHB-g-AA/MWNTs-OH composites, and the MWNTs-OH were also examined by XRD technique, and the resulting XRD diagrams are presented in Figure . Similar to the result proposed by Galego et al. Citation[2], neat PHB (Figure ) exhibits seven peaks at about 13.1°, 16.9°, 19.6°, 21.3°, 22.5°, 25.6°, and 27.1°. As the comparison between the XRD patterns of neat PHB and PHB/MWNTs composite (Figure ), it is found that there is only an extra peak at about 23.8° in the spectrum of the latter, coming from the structure of MWNTs Citation[38]. This result reveals that MWNTs is dispersed physically in the PHB matrix. As compared with Figure , a new peak appears at 18.1° in the XRD pattern of the PHB-g-AA/MWNTs-OH composite (Figure ). This new peak, as identified by Shogren et al. Citation[39], was caused by the formation of an ester carbonyl group, and provided the evidence that the crystalline structure of PHB/MWNTs was different from that of PHB-g-AA/MWNTs-OH.
3.4 Thermal properties of blends
The thermal properties (T m, T g, ΔH m) of hybrids with various MWNTs or MWNTs-OH contents were obtained from the temperature and the area of melting peaks of DSC heating thermograms (not shown here), and the results are given Table . The glass transition temperature (T g) of the hybrids was associated with a cooperative motion of long-chain segments, which may be hindered by the inorganic filler (MWNTs or MWNTs-OH). Therefore, as expected, both the PHB/MWNTs and the PHB-g-AA/MWNTs-OH gave the higher glass transition temperature than the neat PHB and the PHB-g-AA copolymer. With the same filler content, Table also shows that the PHB-g-AA/MWNTs-OH hybrid has higher T g value than the PHB/MWNTs one. The larger enhancement in T g for PHB-g-AA/MWNTs-OH hybrids may be due to the formation of chemical bonds via the condensation reaction between PHB-g-AA and MWNTs-OH since these strong bonds are able to hinder the motion of the polymer chains. For the PHB/MWNTs hybrids, the improvement in T g was slight since MWNTs was dispersed physically in the PHB matrix. It was also found that the T g value of PHB-g-AA/MWNTs-OH hybrids increased with increasing MWNTs-OH content to a maximum value at 1 wt.%, and then decreased. This result may be due to the low grafting percentage (about 5.60 wt.%) of the PHB-g-AA copolymer since the increment of T g depended on the number of functional groups in the copolymer matrix to react with the hydroxyl groups in the MWNTs-OH Citation[40]. With MWNTs-OH content above 1 wt.%, the excess fillers were dispersed physically in the polymer matrix. Such excess MWNTs-OH brought separation of the organic and inorganic phases, and reduced their compatibility, causing the enhancement on the T g value became slightly.
Table 1. Thermal properties and tensile strength at break of PHB/MWNTs and PHB-g-AA/MWNTs-OH hybrids.
Table also shows that the addition of inorganic filler (MWNTs or MWNTs-OH) reduces the melting temperature (T m) for both hybrids, and that the lowest T m occurs at 1 wt.%. Notably, with the same filler content, all the PHB-g-AA/MWNTs-OH hybrids gave lower value of T m than their PHB/MWNTs equivalents. This lower melting temperature of PHB-g-AA/MWNTs-OH made it a more easily processed blend. The value of melting enthalpy indicated the crystallinity of a polymer. For both hybrids (PHB/MWNTs and PHB-g-AA/MWNTs-OH), their melting enthalpy decreases with an increase of MWNTs or MWNTs-OH content. The decrease in crystallization was probably caused by increased difficulty in arranging the polymer chain because of MWNTs or MWNTs-OH prohibiting movement of the polymer segments. Additionally, the melting enthalpy of PHB-g-AA was lower than the neat PHB but the ΔH m value of PHB-g-AA/MWNTs-OH blends was about 2–7 J/g higher than that of PHB/MWNTs ones, caused by the formation of the ester carbonyl functional group from the reaction between the −OH group of MWNTs-OH and the −COOH group of PHB-g-AA.
The IDT values of hybrids were obtained from the shoulder of the mass loss curves of PHB/MWNTs (not shown here) and PHB-g-AA/MWNTs-OH (Figure ), and the result is summarized in Table . Figure illustrates that there is no weight loss up to 220 °C; above this temperature only the single-step degradation occurs for all polymers. For the plain PHB, mostly statistical intramolecular decomposition with formation of crotonate end groups takes place during thermal but PHB thermal decomposition could be easily decreased by carboxylate end groups due to E1cB mechanism. So, PHB-g-AA had a lower IDT value than PHB Citation[41,42]. As compared with the neat PHB or the PHB-g-AA copolymer, the PHB/MWNTs or PHB-g-AA/MWNTs-OH hybrids had a positive effect on the value of IDT and gave the higher amount of residual ash (Figure ). With same filler content, it can be seen from Table that the PHB-g-AA/MWNTs-OH hybrid exhibits the higher IDT value than the PHB/MWNTs one. This outcome was a result of the difference in interfacial forces in the two hybrids: the weaker hydrogen bonds of PHB/MWNTs compared with the stronger coordination sites associated with the carboxylic acid groups of PHB-g-AA and the –OH group of MWNTs-OH. A comparison between PHB-g-AA and PHB-g-AA/MWNTs-OH showed that the increment of IDT was about 80 °C with 1 wt.% MWNTs-OH but the increment is only about 20 °C as the MWNTs-OH content was increased from 1 wt.% to 2 wt.%. This result further confirmed that the optimal loading of MWNTs-OH was 1 wt.% because excess MWNTs-OH caused separation of the organic and inorganic phases and impaired their compatibility and dispersability (Figure ).
Figure 6 TEM micrographs for PHB-g-AA/MWNTs-OH composites with different MWNTs-OH contents: (A) 1.0 wt.% and (B) 3.0 wt.%.
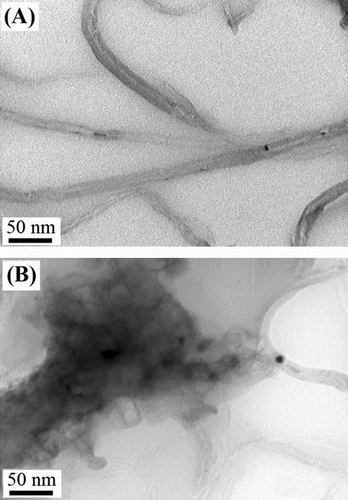
In addition, the residual yields of the PHB-g-AA/MWNTs-OH nanocomposites increased with increasing MWNTs-OH content, indicating that thermal decomposition of the polymer matrix was retarded in the PHB-g-AA/MWNTs-OH nanocomposites. This result may be attributed to a physical barrier effect, resulting from the fact that MWNTs-OH would prevent the transport of decomposition products in the polymer nanocomposites. Similar observations had been reported that the thermal stability of polypropylene/MWNTs nanocomposites was improved by a physical barrier effect, enhanced by ablative reassembling of the MWNTs layer Citation[43]. Therefore, the TGA results demonstrated that the incorporation of a small quantity of MWNTs-OH could significantly improve the thermal stability of the PHB-g-AA/MWNTs-OH nanocomposites.
3.5 Hybrid morphology
It is necessary to study the morphology of the MWNTs-OH and polymer blends since the mechanical properties depend on it. In this study, the TEM was used to examine the tensile fractured surfaces of PHB-g-AA/MWNTs-OH blends and their micrographs are shown in Figure . It is clear that the individually embedded MWNTs-OH in the polymer matrix is really difficult to find if insufficient dispersion and poor interfacial adhesion between filler and matrix occur. So, the lack of wettability may lead to some agglomerated MWNTs-OH clusters. As shown in Figure , the MWNTs-OH can be dispersed well in the polymer matrix for 1 wt.% of MWNTs-OH but the formation of agglomerates can be observed for 3 wt.% MWNTs-OH. The reason for this result was that the properties of MWNTs-OH surfaces and PHB-g-AA matrix became more similar because the PHB-g-AA/MWNTs-OH blend could produce branched ester carbonyl bonds from the condensation reaction between them.
3.6 Mechanical properties of hybrids
The effect of MWNTs-OH content on the stress–strain curves of PHB-g-AA/MWNTs-OH blends is presented in Figure . It can be seen that all the stress–strain curves were nearly linear up to the breaking strain for the PHB-g-AA copolymer and PHB-g-AA/MWNTs-OH blends. For the PHB-g-AA copolymer, its film yielded up to a breaking strain of 7.9% with a stress of 16.3 MPa. For the PHB-g-AA/MWNTs-OH nanocomposites, this breaking strain tended to decrease with increasing MWNTs-OH content and occurred at 3.9% with a stress of 25.6 MPa for the 3 wt.% composite followed by a stiffing of the material Citation[44]. The tensile strength (TS) at break of PHB/MWNTs and PHB-g-AA/MWNTs-OH was obtained from their stress–strain curves, and the result was listed in Table . For the PHB/MWNTs blends, the TS value decreased with increasing MWNTs content because the interfacial force between PHB and MWNTs was only one of relatively weak hydrogen bonds. The PHB-g-AA/MWNTs-OH hybrid exhibited much better tensile strength than the equivalent PHB/MWNTs, even though PHB-g-AA had a lower tensile strength than pure PHB. However, the TS value of PHB-g-AA/MWNTs-OH increased with increasing MWNTs-OH content up to 1 wt.% and then decreased. The positive effect on tensile strength was attributed to the presence of immobilized or partially immobilized polymer phases Citation[45], high aspect ratio and surface area of the carbon nanotubes, the nanoscale dispersion of MWNTs-OH layers in the polymer matrix, and the formation of chemical bonds through the condensation reaction between PHB-g-AA and MWNTs-OH. For MWNTs-OH content above 1 wt.%, the decrease in tensile strength could be attributed to the inevitable aggregation of the MWNTs-OH in higher MWNTs-OH content (see Table ).
Figure 7 Representative stress–strain curves for PHB-g-AA-based composites with different MWNTs-OH contents: (A) 0 wt.%, (B) 0.5 wt.%, (C) 1.0 wt.%, and (D) 3.0 wt.%.
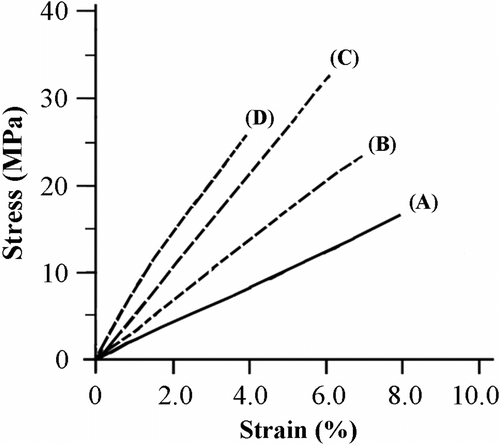
Table 2. The decomposition temperature at 5 wt.%, maximum decomposition rate, 95 wt.% for neat PHB and blends.
4 Conclusions
We report a simple method for the functionalization of multi-walled carbon nanotubes and the preparation of high performance poly(3-hydroxybutyrate)/MWNTs nanocomposites. For the modification of MWNTs, the process involves the chemical oxidation to cleave MWNTs and to open the tube ends, the creation of acyl chloride functionalities on the MWNTs, and the conversion of acyl chlorides into hydroxyls with excess 1,3-propanediol. Hydroxyl groups’ functionalization makes the carbon nanotubes very effective side chain. We have demonstrated that the MWNTs-OH can be incorporated into the PHB-g-AA copolymer through the formation of strong covalent bonds producing from the reaction between carboxylic acid groups of PHB-g-AA and hydroxyl groups of MWNTs-OH. Thus, MWNTs-OH can play a reinforcement role in the PHB-g-AA polymer matrix. FTIR and 13C solid-state NMR spectra showed that the acrylic acid had been grafted onto the PHB copolymer and that ester carbonyl bonds had formed in the PHB-g-AA/MWNTs-OH hybrid. The newly formed ester carbonyl bonds may be produced through dehydration of carboxylic acid groups in the PHB-g-AA matrix with grafted hydroxyl groups in the MWNTs-OH. The TEM examination also proves that the formation of agglomerates of carbon nanotubes can be observed for hybrids with higher MWNTs-OH content. As the result of DSC analysis, it can be found that the gap between T g and T m of the PHB-g-AA/MWNTs-OH hybrid is smaller than that of the PHB/MWNTs hybrid. This implies that the compatibility between PHB and MWNTs has been enhanced. TGA tests show that the PHB-g-AA/MWNTs-OH hybrid gives much better thermal and mechanical properties than the neat PHB. Maximum values of tensile strength of the PHB-g-AA/MWNTs-OH hybrid occurred at about 1 wt.% of MWNTs-OH and excess MWNTs-OH reduced the compatibility of hybrid due to the inevitable aggregation of carbon nanotubes. Finally, though the carbon nanotubes were not involved in biodegradation, the preparation of PHB-g-AA/MWNTs-OH nanocomposites was worthy to study since the optimal amount of MWNTs-OH was 1 wt.% only.
References
- Savenkova , L , Gercberga , LZ , Nikolaeva , V , Dzene , A , Bibers , I and Kalnin , B . 2000 . Mechanical properties and biodegradation characteristics of PHB-based films . Process Biochemistry , 35 : 573 – 9 .
- Galego , N , Rozsa , C , Sanchez , R , Fung , J , Vazquez , A and Tomas , JS . 2000 . Characterization and application of poly(β-hydroxyalkanoates) family as composite biomaterials . Polymer Testing Journal , 19 : 485 – 95 .
- Zhang , L , Deng , X , Zhao , S and Huang , Z . 1997 . Biodegradable polymer blends of poly(3-hydroxybutyrate) and hydroxyethyl cellulose acetate . Polymer , 38 : 6001 – 7 .
- Yoon , JS , Lee , WS , Jin , HJ , Chin , IJ , Kim , MN and Go , JH . 1999 . Toughening of poly(3-hydroxybutyrate) with poly(cis-1,4-isoprene) . European Polymer Journal , 35 : 781 – 8 .
- Wu , CS . 2005 . Improving polylactide/starch biocomposite by grafting polylactide with acrylic acid—characterization and biodegradability assessment . Macromolecular Bioscience , 5 : 352 – 61 .
- Ikejima , T and Inoue , Y . 2000 . Crystallization behavior and environmental biodegradability of the blend films of poly(3-hydroxybutyric acid) with chitin and chitosan . Carbohydrate Polymers , 41 : 351 – 7 .
- Godbole , S , Gote , S , Latkar , M and Chakrabarti , T . 2003 . Preparation and characterization of biodegradable poly-3-hydroxybutyrate–starch blend films . Bioresource Technology , 86 : 33 – 7 .
- Tseng , CR , Wu , JY , Lee , YH and Chang , FC . 2001 . Preparation and crystallization behavior of syndiotactic polystyrene–clay nanocomposites . Polymer , 42 : 10063 – 70 .
- Vaia , RA , Ishii , H and Giannelis , EP . 1993 . Synthesis and properties of two-dimensional nanostructures by direct intercalation of polymer melts in layered silicates . Chemistry of Materials , 5 : 1694 – 6 .
- Okamoto , M , Morita , S and Kotaka , T . 2001 . Dispersed structure and ionic conductivity of smectic clay/polymer nanocomposites . Polymer , 42 : 2685 – 8 .
- Iijima , S . 1991 . Helical microtubules of graphitic carbon . Nature , 354 : 56 – 58 .
- Yao , Z , Braidy , N , Botton , GA and Adronov , A . 2003 . Polymerization from the surface of single-walled carbon nanotubes − preparation and characterization of nanocomposites . Journal of the American Chemical Society , 125 : 16015 – 24 .
- Qin , S , Qin , D , Ford , WT , Resasco , DE and Herrera , JE . 2004 . Functionalization of single-walled carbon nanotubes with polystyrene via grafting to and grafting from methods . Macromolecules , 37 : 752 – 7 .
- Lepoitevin , B , Pantoustier , N , Alexandre , M , Calberg , C , Jerome , R and Dubois , P . 2002 . Polyester layered silicate nanohybrids by controlled grafting polymerization . Journal of Materials Chemistry , 12 : 3528 – 32 .
- Fisher , FT , Bradshaw , RD and Brinson , LC . 2002 . Effects of nanotube waviness on the modulus of nanotube-reinforced polymers . Applied Physics Letters , 80 : 4647 – 9 .
- Chen , J , Ramasubramaniam , R , Xue , C and Liu , H . 2006 . A versatile, molecular engineering approach to simultaneously enhanced, multifunctional carbon-nanotube–polymer composites . Advanced Functional Materials , 16 : 114 – 9 .
- Liu , IC , Huang , HM , Chang , CY , Tsai , HC , Hsu , CH and Tsiang , RC . 2004 . Preparing a styrenic polymer composite containing well-dispersed carbon nanotubes: anionic polymerization of a nanotube-bound p-methylstyrene . Macromolecules , 37 : 283 – 7 .
- Hu , H , Ni , Y , Montana , V , Haddon , RC and Parpura , V . 2004 . Chemically functionalized carbon nanotubes as substrates for neuronal growth . Nano Letters , 4 : 507 – 11 .
- Lin , J , Rinzler , AG , Dai , H , Hafner , JH , Bradley , RK , Boul , PJ , Lu , A , Iverson , T , Shelimov , K , Huffman , CB , Rodriguez-Macias , F , Shon , YS , Lee , TR , Colbert , DT and Smalley , RE . 1998 . Fullerene pipes . Science , 280 : 1253 – 6 .
- Zhang , Y , Li , J , Shen , Y and Wang , M . 2004 . Poly-l-lysine functionalization of single-walled carbon nanotubes . Journal of Physical Chemistry B , 108 : 15343 – 6 .
- Chen , J , Hamon , MA , Hu , H , Chen , Y , Rao , AM , Eklund , PC and Haddon , RC . 1998 . Applications of functionalized single-walled carbon nanotubes . Science , 282 : 95 – 8 .
- Zeng , HL , Gao , C and Yan , DY . 2006 . Poly(caprolactone)-functionalized carbon nanotubes and their biodegradation properties . Advanced Functional Materials , 16 : 812 – 8 .
- Zhang , J , Zou , H , Qing , Q , Yang , Y , Li , Q , Liu , Z , Guo , X and Du , Z . 2003 . Effect of chemical oxidation on the structure of single-walled carbon nanotubes . Journal of Physical Chemistry B , 107 : 3712 – 8 .
- Jung , YC , Sahoo , NG and Cho , JW . 2006 . Polymeric nanocomposites of polyurethane block copolymers and functionalized multi-walled carbon nanotubes as crosslinkers . Macromolecular Rapid Communications , 27 : 126 – 31 .
- Zhu , J , Peng , H , Fernando , RM , Margrave , JL , Khabashesku , VN , Imam , AM , Lozano , K and Barrer , EV . 2004 . Reinforcing epoxy polymer composites through covalent integration of functionalized nanotubes . Advanced Functional Materials , 14 : 643 – 8 .
- Sano , M , Kamino , A , Okamura , J and Shinkai , S . 2001 . Self-organization of PEO-graft-single-walled carbon nanotubes in solutions and Langmuir−Blodgett films . Langmuir , 17 : 5125 – 8 .
- Xu , C and Qiu , Z . 2009 . Synthesis and eutectic behavior of liquid crystalline copolyesters . Journal of Polymer Science Part B: Polymer Physics , 47 : 2171 – 7 .
- Liao , HT and Wu , CS . 2007 . Performance of an acrylic-acid-grafted poly(3-hydroxybutyric acid)/starch bio-blend: characterization and physical properties . Designed Monomers and Polymers , 10 : 1 – 18 .
- Huang , HM , Lin , IC , Chang , CY , Tsai , HC , Hsu , CH and Tsiang , RC . 2004 . Preparing a polystyrene-functionalized multiple-walled carbon nanotubes via covalently linking acyl chloride functionalities with living polystyryllithium . Journal of Polymer Science Part A: Polymer Chemistry , 42 : 5802 – 10 .
- Besteman , K , Lee , JO , Wiertz , FGM , Heering , HA and Dekker , C . 2003 . Enzyme-coated carbon nanotubes as single-molecule biosensors . Nano Letters , 3 : 727 – 30 .
- Chen , S , Wu , G and Liu , Y . 2006 . Preparation of poly(acrylic acid) grafted multiwalled carbon nanotubes by a two-step irradiation technique . Macromolecules , 39 : 330 – 4 .
- Jedlinski , Z , Kowalczuk , M , Adamus , G , Sikorska , W and Rydz , J . 1999 . Novel synthesis of functionalized poly(3-hydroxybutanoic acid) and its copolymers . International Journal of Biological Macromolecules , 25 : 247 – 53 .
- Xu , J , Guo , BH , Yang , R , Wu , Q , Chen , GQ and Zhang , ZM . 2002 . In situ FTIR study on melting and crystallization of polyhydroxyalkanoates . Polymer , 43 : 6893 – 9 .
- Wu , CS and Laio , HT . 2002 . Influence of a compatibilizer on the properties of polyethylene–octene elastomer/starch blends . Journal of Applied Polymer Science , 86 : 1792 – 8 .
- Kim , J and Tirrell , DA . 1999 . Synthesis of well-defined poly(2-ethylacrylic acid) . Macromolecules , 32 : 945 – 8 .
- Bikiaris , D and Panayiotou , C . 1998 . LDPE/starch blends compatibilized with PE-g-MA copolymers . Journal of Applied Polymer Science , 70 : 1503 – 21 .
- Doi , Y , Kunioka , M , Nakamura , Y and Soga , K . 1988 . Nuclear magnetic resonance studies on unusual bacterial copolyesters of 3-hydroxybutyrate and 4-hydroxybutyrate . Macromolecules , 21 : 2722 – 7 .
- Bellayer , S , Gilman , JW , Eidelman , N , Bourbigot , S , Flambard , X , Fox , DM , Long , HCD and Yrulove , PC . 2005 . Preparation of homogeneously dispersed multiwalled carbon nanotube/polystyrene nanocomposites via melt extrusion using trialkyl imidazolium compatibilize . Advanced Functional Materials , 15 : 910 – 6 .
- Shogren , RL , Thompson , AR , Felker , FC , Harry-Okuru , RE , Gordon , SH , Greeen , RV and Gould , JM . 1992 . Polymer compatibility and biodegradation of starch–poly(ethylene-co-acrylic acid)–polyethylene blends . Journal of Applied Polymer Science , 44 : 1971 – 8 .
- Xu , Y , Gao , C , Kong , H , Yan , D , Jin , YZ and Watts , PCP . 2004 . Growing multihydroxyl hyperbranched polymers on the surfaces of carbon nanotubes by in situ ring-opening polymerization . Macromolecules , 37 : 8846 – 53 .
- Ariffin , H , Nishida , H , Shirai , Y and Hassan , MA . 2008 . Determination of multiple thermal degradation mechanisms of poly(3-hydroxybutyrate) . Polymer Degradation and Stability , 93 : 1433 – 9 .
- Ariffin , H , Nishida , H , Shirai , Y and Hassan , MA . 2010 . Highly selective transformation of poly[(R)-3-hydroxybutyric acid] into trans-crotonic acid by catalytic thermal degradation . Polymer Degradation and Stability , 95 : 1375 – 81 .
- Bom , D , Andrews , R , Sreekumar , TV , Kumar , S , Moore , VC , Hauge , RH and Smalley , RE . 2002 . Thermogravimetric analysis of the oxidation of multiwalled carbon nanotubes: evidence for the role of defect sites in carbon nanotube chemistry . Nano Letters , 2 : 615 – 9 .
- Coleman , JN , Cadek , M , Blake , R , Nicolosi , V , Ryan , KP , Belton , C , Fonseca , A , Nagy , JB , Cun-ko , YK and Blau , WJ . 2004 . High performance nanotube-reinforced plastics: understanding the mechanism of strength increase . Advanced Functional Materials , 14 : 791 – 8 .
- Zhang , X , Liu , T , Sreekumar , TV , Kumar , S , Moore , VC , Hauge , RH and Smalley , RE . 2003 . Poly(vinyl alcohol)/SWNT composite film . Nano Letters , 3 : 1285 – 8 .