Abstract
Nanoporous silica aerogel particles were synthesized via sol–gel process and modified with a hydrophobic surfactant. Batch polymerizations of styrene in presence of silica aerogel particles were studied via free radical and reversible addition–fragmentation chain transfer (RAFT) polymerizations. Monomer conversion, molecular weight, and polydispersity index of each system were monitored during polymerization to investigate the reaction kinetics. According to results, in both systems, the presence of silica aerogel particles has a sensible influence on polymerization kinetic and adding aerogels results in decreased polymerization rate, conversion, and molecular weight. Moreover, the prepared samples were characterized by Fourier transform infrared spectroscopy. Also, thermal gravimetric analysis (TGA) and differential scanning calorimetric techniques was used to observe the effect of aerogel particles on thermal properties of synthesized nanocomposites. According to TGA depiction, in free radical samples, one-stage degradation, related to random chain scission, is observed while degradation of RAFT-prepared nanocomposites occurred in two steps due to the decomposition of RAFT moieties and random chain scission.
1. Introduction
Nanoporous materials have gained much attention due to their potential applications in industrial processes. Porous solids with the nanometer pore sizes are high performance materials, because the proportion of surface area is great and the surface atoms exhibit different properties compared to the atoms in bulk.[1–2]Citation1Citation2 Out of all known solid porous materials, aerogels are particularly known for their small pore size, large specific surface area, and best optical transmission. These materials are consisting of more than 90% air and less than 10% solid materials in the form of high cross-linked network structures.Citation[3] Among all aerogels, silica aerogels have received significant attention in many fields, because they possess a wide variety of exceptional properties such as high porosity, low density, high specific surface area, and low thermal conductivity.[4–6]Citation4Citation5Citation6
Until now, different polymerization techniques have been adapted for the preparation of polymer nanocomposites. Among them, controlled living radical polymerization systems are used for preparation of nanocomposites with narrow molecular weight distribution and well-defined topology.Citation[7] The most prominent of these techniques are the nitroxide-mediated polymerization,Citation[8] the atom transfer radical polymerization,Citation[9] and the reversible addition fragmentation chain transfer (RAFT) polymerization.Citation[10] Some advantages of RAFT polymerization include the applicability toward a wide range of monomers, no need a specific catalyst, initiator, or difficult reaction condition; also, it is convenient to the mild condition and conventional free radical initiators.Citation[11]
Type and amount of nanoparticles affect the kinetics of polymerization and change the physical properties of nanocomposites.[12–13]Citation12Citation13 Many kinds of silica nanoparticles have been used to synthesize polymer nanocomposites via different polymerization systems.Citation[14] Although some research works have been done on the properties of aerogel/polymer nanocomposites,[15–17]Citation15Citation16Citation17 there is no study on the effect of aerogel particles on the kinetics of polymerization and physical properties of nanocomposites. In this work, free radical and RAFT polymerizations of styrene, including different amounts of nanoporous particles (silica aerogel), were investigated. The changes in conversion, molecular weight, and polydispersity index (PDI) values were evaluated during polymerization in both free radical and RAFT systems. Also, thermal gravimetric analysis (TGA) and differential scanning calorimetry (DSC) techniques was used to observe the effect of aerogel nanoparticles on thermal properties of synthesized nanocomposites.
2 Experimental part
2.1 Materials
Styrene (Merck, 99%) was passed through a basic alumina-filled column and dried over calcium hydride. Azobisisobutyronitrile (AIBN, Acros) was recrystallized from methanol. Toluene (Merck, 99%), tetrahydrofuran (THF, Merck, 99%), methanol (Merck, 99%), ethanol (Merck, 99%), cetyltrimethylammonium bromide (CTAB, Merck), acetone (Merck, EMPLURA®), chloroform (Merck, EMPLURA®), hydrochloric acid (Merck, 37%), 1-dodecanetiol (Riedel-de Haen, 99%), carbon disulfide (Fluka, >99%), magnesium sulfate (Daejung), n-hexane (Sigma–Aldrich, >95%), basic aluminum oxide (Fluka), tetraethoxysilane (TEOS, Merck), oxalic acid (Aldrich, >99%), ammonium hydroxide (Tabriz Petrochemical Company), and hexamethyldisilazane (Aldrich, 99%) were used as received.
2.2 Synthesis of silica aerogel particles
Silica aerogel particles were prepared by a two-step, acid–base, catalyzed sol–gel polymerization followed by the ambient pressure drying as reported previously.Citation[18] TEOS was used as a precursor for preparation of silica sol. Oxalic acid and ammonium hydroxide (1 M) were used as acidic and basic catalyst, respectively, with the molar ratio of 1:16:3.5 for [TEOS]:[EtOH]:[oxalic acid]. This mixture was stirred for 30 min and kept at room temperature for 24 h. Adding basic catalyst, the mixture kept for gelation at room temperature. After the formation of the gel, more Ethanol was added to prevent shrinkage and cracks formation and the gel was kept for 4 h at 50 °C. The obtained hydrogel was washed by n-hexane and immersed in n-hexane for 24 h at 50 °C for solvent exchanging. Then the wet gel immersed in solution of n-hexane/hexamethyldisilazane (mol ratio of [TEOS]:[HDMS] 1:0.68) for surface modification at 50 °C for 24 h. The surface-modified wet gel was washed repeatedly in n-hexane to remove the unreacted surface modifier agents and dried at 50 °C for 1 h, at 150 °C for 2 h and at 200 °C for 1 h.
2.3 Preparation of 2-(dodecylthiocarbonothioylthio)-2-methylpropionic acid (DDMAT)
DDMAT was synthesized according to the previously reported procedure.Citation[19] 1-Dodecanethiol (40.400 g, 0.20 mol), acetone (0.12 g, 1.655 mol), and CTAB (3.245 g, 0.0089 mol) were added into a 250 mL round-bottom flask equipped with mechanical stirrer and stirred for 5 min at 12.5 °C under ambient atmosphere. Sodium hydroxide solution (50%) (16.800 g, 0.21 mol) was added dropwise over 10 min and then the mixture was stirred for additional 20 min. Afterward, the solution of carbon disulfide (12.1 mL, 0.20 mol) in acetone (0.02 mL, 0.345 mol) was added for over 30 min. Ten minutes later, chloroform (24.2 mL, 0.3 mol) was added in one portion, followed by dropwise addition of 50% sodium hydroxide solution (40 g) over 40 min. The mixture was stirred overnight and filtered and washed thoroughly with acetone. Acetone was removed under reduced pressure and the obtained yellow powder was dissolved in deionized water (200 mL) and then precipitated by adding 30 mL of HCl (37%). DDMAT was collected by filtration and dried under reduced pressure. Then, DDMAT was dissolved in 400 mL of n-hexane and the solution was dried over anhydrous MgSO4, and filtered to remove byproducts. DDMAT was recrystallized from the solution, and filtered and dried under vacuum (12 g, yield: 32.9%).
1HNMR (in CDCl3): 0.89 ppm (t, 3 H), 1.30–1.5 ppm (m, 18H), 1.6–1.7 ppm (m, 2H), 1.7–1.8 ppm (s, 6H), 3.2 ppm (t, 2H), 11.0 ppm (s, 1H).
Fourier transform infrared spectroscopy (FT IR): 2849 cm−1 (CH), 1716 cm−1 (C=O), 1069 cm−1 (C=S), 814 cm−1 (C–S).
2.4 Polymerization of styrene via free radical and RAFT polymerization
Batch polymerizations were performed in the glass vials which were placed in an oil bath thermostated at 70 °C. Polymerization reactions with different content of nanoparticles were run for 12 h for free radical systems and 16 h for RAFT systems. At first, various amounts of dried silica aerogel particles (from 1 to 5 wt.% relative to monomer) along with RAFT agent (3.05 mmol, 1.114 g, in RAFT polymerizations) in certain quantity of styrene monomer (0.3 mol, 35 mL) was dispersed and AIBN (0.6 mmol, 0.100 g) was added into a 250 mL lab reactor and mixed for 2 h. Then, the mixture was equally transferred into the glass vials by means of a syringe and was then degassed under the nitrogen atmosphere, sealed, and placed in an oil bath at 70 °C. The polymerizations were stopped by cooling the reaction mixture in ice water after distinct time periods. Cool methanol was used to precipitate polymer chains. After weight measurements, the samples were dried in a vacuum oven for a night. Nanocomposites were named as Fi and Ri samples in which F, R, and i denote free radical polymerization, RAFT polymerization, and content of particles, respectively.
2.5 Separation of polymer chains from particles
The prepared nanocomposites were dissolved in THF and the polymer chains were separated from particles by high-speed ultracentrifugation (10,000 rpm) for three times. The solution was passed through a 0.2 μm regenerated cellulose filter and then poured into methanol (Vmethanol/Vsolution was 10) to precipitate polymer chains. After filtration, the polymer was dried overnight in a vacuum oven at temperature 50 °C.
2.6 Characterization
FTIR spectra were recorded on a Bomem FTIR spectrophotometer, within a range of 500–4000 cm−1using a resolution of 4 cm−1. An average of 32 scans has been reported for each sample. The samples were prepared on a KBr pellet in vacuum desiccators under a pressure of 0.01 torr. Thermal gravimetric analyses were carried out with a PL thermogravimetric analyzer (Polymer Laboratories, TGA 1000, UK). The thermograms were obtained from an ambient temperature of 600 °C at a heating rate of 10 °C/min. A sample weight of about 10 mg was used for all the measurements, and nitrogen was used as the purging gas at a flow rate of 50 mL/min. The average molecular weights and molecular weight distributions were measured by gel permeation chromatography technique. A Waters 2000 ALLIANCE with a set of three columns of pore sizes of 10,000, 1000, and 500 Å was utilized to determine polymer average molecular weight and PDI. THF was used as the eluent at a flow rate of 1.0 mL/min, and the calibration was carried out using low polydispersity polystyrene standards. Transmission electron microscope (TEM), Philips EM 208, with an accelerating voltage of 80 kV was used to study the morphology of the nanocomposites; the samples of 70 nm thickness were prepared by Reichert-ultramicrotome (type OMU 3). Thermal analyses were carried out using a DSC instrument (NETZSCH DSC 200 F3, Netzsch Co, Selb/Bavaria, Germany). Nitrogen at a rate of 50 mL/min was used as the purging gas. Aluminum pans containing 3 mg samples were sealed using the DSC sample press. The samples were heated to an ambient temperature of 180 °C at a heating rate of 10 °C/min. Tg was obtained as the inflection point of the heat capacity jump. Spectroscopic characterization by H NMR was performed at room temperature with a Bruker 300 MHz instrument using CDCl3 as solvent. Materials porosity was characterized by N2 adsorption/desorption curves obtained with a Quntasurb QS18(Quntachrom) apparatus. Surface area value and pore size distribution were obtained with the corrected BET equation and Broekhoff and de Boermodels, respectively.
3 Results and discussion
3.1 Characteristics of silica aerogel particles
Since the morphology and topology of nanoparticles affects the polymerization kinetics directly [20–21]Citation20Citation21, we firstly investigated the characteristics of aerogel particles used in our work. As can be seen in Figure , SEM image of synthesized aerogel particles shows that majority of particles are shaped spherical with diameter about 20 nm. Also, aerogel structure formed from silica nanoparticles is spongy as expected. High specific surface area (∼857 m2 g−1) and nanoscale pore size (∼6 nm), which has been obtained by isothermal nitrogen adsorption–desorption indicate that structure is highly porous as expected. Also, TEM image of synthesized particles depicts a spongy structure formed from silica particles (see Figure ).
3.2 Polymerization kinetics
To investigate the effect of particles content on polymerization kinetics, bulk polymerization of styrene initiated with AIBN at 70 °C with different amounts of silica aerogels was carried out. In all experiments, the molar concentration of initiator (and DDMAT in RAFT polymerization experiments) was kept constant while various amounts of aerogel particles, including 0, 1, 3, and 5 wt.% relative to monomer, were loaded to the mixture. Figure depicts monomer conversion during free radical and RAFT polymerization systems. In both systems, the presence of aerogel particles has little effect on the kinetics in early stages of polymerization. The maximum polymerization rate is observed in samples with no aerogel particles, i.e. more aerogels results in lower polymerization rate and conversion. However, some researchers reported that silica nanoparticles slow down the polymerization rate because they absorb UV radiation in UV-curing systems.Citation[22] In other studies, silica nanoparticles have resulted in an increase the rate of polymerization which has been attributed to changes in propagation and termination rate constants.[23–25]Citation23Citation24Citation25 Also, modified nanoparticles as multifunctional sites form a three-dimensional network which leads to reduction in the mobility of chains and thus slow down the rate of polymerization.[24–25]Citation24Citation25 Due to the formation of aggregates, greater particle size leads to higher rate of reaction, which is mainly because of high medium viscosity and the occurrence of auto-acceleration phenomenon.[24–25]Citation24Citation25 On the other hand, in some studies, an optimum loading of silica nanoparticles has been reported in which the polymerization rate reaches the maximum value.[26–29]Citation26Citation27Citation28Citation29 In these studies, silica nanoparticles can improve the mobility and diffusion of propagating radicals, which lead to increase in the rate of polymerization. In this work, the reduction of polymerization rate at higher amount of particles has been attributed to less system stability due to the formation of aggregates.Citation[13] Also, the radicals have to move in the space contains aerogel particles for finding other free radicals and monomers and should overcome resistances from reagents and silica aerogel particles. Therefore, in a media with large amount of silica aerogels, the movement of macroradicals is suppressed leading to the reduction of polymerization rate and conversion. In some RAFT polymerization reactions, an induction period is observed. However, DDMAT as RAFT agent which contains appropriate leaving/reinitiator group results in quick reinitiation and the simultaneous growth of the polymer chains with no induction time.
Figure 3 Monomer conversion vs. free radical (A) and RAFT (B) polymerization time of styrene at 70 °C with different loadings of silica aerogel.
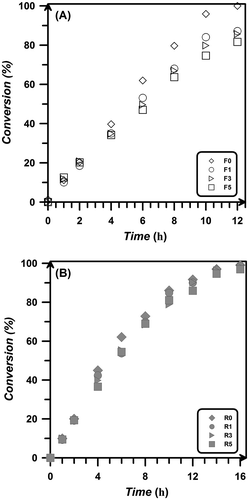
The number average molecular weight (Mn) and PDI values with conversion are shown in Figure . Although there is no significant effect of particle content on the Mn values, in samples with higher amount of aerogel particles, a decrease in molecular weight is observable. In a RAFT polymerization, theoretical Mn relates to the conversion via Equation (1):(1)
Figure 4 Molecular weights and PDI as a function of conversion for free radical (A) and RAFT polymerization (B).
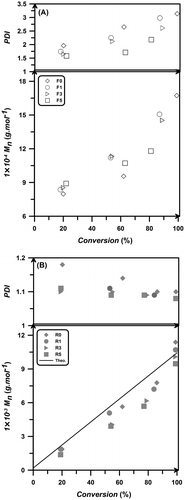
where [M]0 and [RAFT]0 are initial concentrations of the monomer and RAFT agent, X is the monomer conversion, and MWmonomer and MWRAFT are the molecular weights of monomer and RAFT agent. According to Equation (1), increase of Mn directly is affected by initial molar ratio of monomer to RAFT agent. Therefore, it is expected that in all these experiments, Mn does not change since the initial molar ratio of monomer to RAFT agent is constant. However, some deviations can be seen by increasing particles content. This may be ascribed to the little decrease in monomer concentration in bulk by physical absorption of monomer. Also, absorbing propagating radicals on the surface and into structure of silica aerogel particles leads to reduce the mobility of polymer chains and slows down the propagation reactions and consequently there is a decrease in the molecular weight values.
In free radical samples, PDI values increase by conversion due to physical phenomena. Increasing conversion leads to increase in viscosity of system which exerts a diffusion-controlled mechanism to polymerization media and consequently leading to increased PDI values. In RAFT systems, adding aerogel particles into the reaction media has no significant effect on PDI values and all values are less than 1.2. Also, by increasing the conversion, PDI values tend to reduce, which indicates the controlled behavior of chain’s growth.
3.3 Characterization of PS/silica aerogel nanocomposites
3.3.1 FTIR results
Figure illustrates FTIR spectra for F0, F3, R0, and R3 nanocomposites. In samples F3 and R3, the peak observed at around 460 cm−1 is attributed to Si–O–Si groups. In the spectra of all samples, the peaks around wave numbers 3080, 2850, 2920, 1600, 1490, 1060, 1020, 750, and 700 cm−1 are corresponding to polystyrene’s C–H aromatic stretching vibration, C–H asymmetric stretching vibration of CH2, C–H symmetric stretching vibration of CH2, C–C stretching frequency of ring in plane, C–H stretching vibration of ring in plane, C–H bending vibration of ring in plane, and C–H out-of-plane bending vibration of ring, respectively.
3.3.2 Thermophysical properties
As shown in Figure , TGA technique was used to study of thermal stability of prepared nanocomposites. According to the results, although aerogel nanoparticles better thermal stabilities of all the nanocomposites in both systems, but this effect is not significant. Assuming the weight loss between 100 and 140 °C is ascribed to the evaporation of water, samples prepared via free radical polymerization show one-stage degradation related to random chain scission, while degradation of nanocomposites prepared by RAFT method occurs in two steps. The first step of degradation is ascribed to the decomposition of thio moiety of RAFT agents which are attached to polymer chains and the second step of degradation is random chain scission. Some thermal properties of nanocomposites are summarized in Table . According to results, random chain scission occurs at 400–420 °C and increasing aerogel content up to 5 wt.% results in a slight increasing of degradation temperature. This little increase results from three-dimensional structure of aerogel particles. These particles are like agglomerated particles which cannot improve the thermal stability due to improper dispersion of particles. Also, thermal properties of free radical samples are better than RAFT nanocomposites which are attributed to lower molecular weight of chains prepared via RAFT polymerization. The degradation of RAFT moieties takes place at 165–185 °C, which is slightly delayed with increasing aerogels content.
Figure 6 TGA thermograms for PS/aerogel nanocomposites prepared via free radical (A) and RAFT (B) polymerization at 70 °C with different content of silica aerogel nanoparticles.
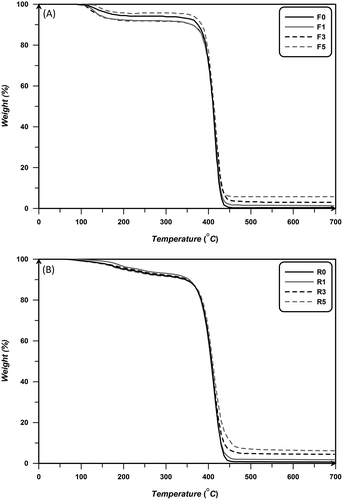
Table 1. Thermophysical properties of PS/silica aerogel nanocomposites prepared via free radical and RAFT polymerization at 70 °C in different contents of nanoparticles.
Effect of particles on chain confinement has been investigated by means of variation of glass transition temperature (Tg) as shown in Table . It can be observed that there is no obvious change in Tg values in free radical system, while the synthesized nanocomposites by RAFT method have slightly lower Tg values than neat polystyrene. Changes in molecular weight Citation[30] and interaction between the particles and polystyrene chains Citation[31] seem to be the most important parameters to see these trends. Since the polymer chains are polystyrene and nanoparticles are silica aerogel nanoparticles, it is concluded that the molecular weight of samples has the main effect on the Tg values. In free radical system, the molecular weight of samples reduces from 167,290 to 117,970 g/mol for F0 to F5. It has been reported the molecular weight in which Tg of polystyrene reaches its infinity value is nearly 100,000 g/mol.Citation[32] So, it is expected that the Tg of samples do not change in free radical samples. Also, this is the main reason why the Tg values of RAFT samples decrease by adding more aerogel particles (molecular weight decreases from 11,386 to 9469 g/mol for R0 to R5). On the other hand, the main effect of particles on Tg values releases from their effect on decreasing molecular weight of prepared samples.
4 Conclusion
To investigate the effect of silica aerogel loading on the polymerization kinetics, styrene was polymerized via free radical and RAFT polymerizations at 70 °C in the silica aerogel loadings of 0, 1, 3, and 5 wt.% with respect to monomer. Adding silica aerogel affects the kinetic of polymerization. In both free radical and RAFT polymerization, monomer conversion decreases with the increasing particle content. In RAFT samples, Mn increases linearly with conversion due to living characteristic of RAFT polymerization. Also, increasing silica aerogel contents leads to decreasing values of Mn in both systems. PDI values for free radical samples are much higher than RAFT samples. In RAFT system, all PDI values are smaller than 1.2, which shows that relatively good control is achieved on molecular weight distribution of polystyrene. The thermal stability of all nanocomposites is not much higher than neat polystyrene. In the case of free radical samples, one-stage degradation is observed, while degradation of RAFT nanocomposites occurred in two steps. Also, thermal properties of free radical samples are better than RAFT nanocomposites. Tg values for free radical nanocomposites did not show any variation by adding silica aerogel, while these values decrease by increasing silica aerogel for RAFT samples. Also, Tg values for free radical nanocomposites are higher than RAFT nanocomposites.
References
- Zeng M, Zhang X, Qi C. Microporous composites prepared by coagulation of a CS/PEG solution in NaOH. Int. J. Polym. Mater. 2010;60:213–222.
- Zhang Wg, Zhou S, Zhang R. Ethylene polymerization catalyzed by TiCl4/MgCl2/MCM-41 catalytic system. Des. Monomers Polym. 2007;10:469–475.
- Hrubesh LW. Aerogels: the world’s lightest solids. Chem. Ind. 1990;24:824–827.
- Bond GC, Flamerz S. Structure and reactivity of titania-supported oxides. Part 3: reaction of isopropanol over vanadia-titania catalysts. Appl. Catal. 1987;33:219–230.
- Mirshafiei-Langari SA, Haddadi-Asl V, Roghani-Mamaqani H, Sobani M, Khezri K. In situ atom transfer radical polymerization of styrene in the presence of nanoporous silica aerogel: kinetic study and investigation of thermal properties. J. Polym. Res. 2013;20:163.
- Mulder CAM, Van Lierop JG. Preparation, densification and characterization of autoclave dried SiO2 gels. In: Fricke J, editor. Aerogels. Berlin: Springer; 1986. p. 68–75.
- Roghani-Mamaqani H, Haddadi-Asl V, Salami-Kalajahi M. In situ controlled radical polymerization: a review on synthesis of well-defined nanocomposites. Polym. Rev. 2012;52:142–188.
- Sinirlioglu D, Muftuoglu AE. Synthesis of an inorganic–organic hybrid material based on polyhedral oligomeric silsesquioxane and polystyrene via nitroxide-mediated polymerization and click reactions. Des. Monomers Polym. 2011;14:273–286.
- Khezri K, Haddadi-Asl V, Roghani-Mamaqani H, Salami-Kalajahi M. Synthesis of well-defined clay encapsulated poly(styrene-co-butyl acrylate) nanocomposite latexes via reverse atom transfer radical polymerization in miniemulsion. J. Polym. Eng. 2012;32:111–119.
- Salami-Kalajahi M, Haddadi-Asl V, Rahimi-Razin S, Behboodi-Sadabad F, Najafi M, Roghani-Mamaqani H. A study on the properties of PMMA/silica nanocomposites prepared via RAFT polymerization. J. Polym. Res. 2012;19 . 9793.
- Rahimi-Razin S, Haddadi-Asl V, Salami-Kalajahi M, Behboodi-Sadabad F, Roghani-Mamaqani H. Properties of matrix-grafted multi-walled carbon nanotube/poly(methyl methacrylate) nanocomposites synthesized by in situ reversible addition-fragmentation chain transfer polymerization. J. Iran. Chem. Soc. 2012;9:877–887.
- Rahimi-Razin S, Salami-Kalajahi M, Haddadi-Asl V, Roghani-Mamaqani H. Effect of different modified nanoclays on the kinetics of preparation and properties of polymer-based nanocomposites. J. Polym. Res. 2012;19 . 9954.
- Salami-Kalajahi M, Haddadi-Asl V, Rahimi-Razin S, Behboodi-Sadabad F, Roghani-Mamaqani H, Hemmati M. Investigating the effect of pristine and modified silica nanoparticles on the kinetics of methyl methacrylate polymerization. Chem. Eng. J. 2011;174:368–375.
- Alavi Nikje MM, Mazaheri Tehrani Z. The effects of functionality of the organifier on the physical properties of polyurethane rigid foam/organified nanosilica. Des. Monomers Polym. 2011;14:263–272.
- Boday DJ, Keng PY, Muriithi B, Pyun J, Loy DA. Mechanically reinforced silica aerogel nanocomposites via surface initiated atom transfer radical polymerizations. J. Mater. Chem. 2010;20:6863–6865.
- Chen-Yang YW, Wang YL, Chen YT, Li YK, Chen HC, Chiu HY. Influence of silica aerogel on the properties of polyethylene oxide-based nanocomposite polymer electrolytes for lithium battery. J. Power Sources. 2008;182:340–348.
- Boday DJ, Stover RJ, Muriithi B, Keller MW, Wertz JT, DeFriend Obrey KA, Loy DA. Strong low-density nanocomposites by chemical vapor deposition and polymerization of cyanoacrylates on aminated silica aerogels. ACS Appl. Mater. Interface. 2009;1:1364–1369.
- Bhagat SD, Kim Y-H, Moon M-J, Ahn Y-S, Yeo J-G. A cost-effective and fast synthesis of nanoporous SiO2 aerogel powders using water-glass via ambient pressure drying route. Solid State Sci. 2007;9:628–635.
- Lai JT, Filla D, Shea R. Functional polymers from novel carboxyl-terminated trithiocarbonates as highly efficient RAFT agents. Macromolecules. 2002;35:6754–6756.
- Salami-Kalajahi M, Haddadi-Asl V, Behboodi-Sadabad F, Rahimi-Razin S, Roghani-Mamaqani H, Hemmati M. Effect of carbon nanotubes on the kinetics of in situ polymerization of methyl methacrylate. NANO. 2012;7 . 1250003.
- Salami-Kalajahi M, Haddadi-Asl V, Rahimi-Razin S, Behboodi-Sadabad F, Roghani-Mamaqani H, Najafi M. Effect of loading and surface modification of nanoparticles on the properties of PMMA/silica nanocomposites prepared via in-situ free radical polymerization. Int. J. Polym. Mater. Polym. Biomater. 2013;62:336–344.
- Xu GC, Li AY, Zhang LD, Wu GS, Yuan XY, Xie T. Synthesis and characterization of silica nanocomposite in situ photopolymerization. J. Appl. Polym. Sci. 2003;90:837–840.
- Li F, Zhou S, Gu G, You B, Wu L. Preparation and characterization of ultraviolet-curable nanocomposite coatings initiated by benzophenone/n-methyl diethanolamine. J. Appl. Polym. Sci. 2005;96:912–918.
- Li F, Zhou S, Wu L. Preparation and characterization of UV-curable MPS-modified silica nanocomposite coats. J. Appl. Polym. Sci. 2005;98:2274–2281.
- Li F, Zhou S, You B, Wu L. Kinetic investigations on the UV-induced photopolymerization of nanocomposites by FTIR spectroscopy. J. Appl. Polym. Sci. 2006;99:1429–1436.
- Cho JD, Kim YB, Ju HT, Hong J-W. The effects of silica nanoparticles on the photocuring behaviors of UV-curable polyester acrylate-based coating systems. Macromol. Res. 2005;13:362–365.
- Sangermano M, Malucelli G, Amerio E, Priola A, Billi E, Rizza G. Photopolymerization of epoxy coatings containing silica nanoparticles. Prog. Org. Coat. 2005;54:134–138.
- Cho JD, Ju HT, Hong J-W. Photocuring kinetics of UV-initiated free-radical photo-polymerizations with and without silica nanoparticles. J. Polym. Sci. Polym. Chem. 2005;43:658–670.
- Sadej-Bajerlain M, Gojzewski H, Andrzejewska E. Monomer/modified nanosilica systems: photopolymerization kinetics and composite characterization. Polymer. 2011;52:1495–1503.
- Dalnoki-Veress K, Forrest JA, Murray C, Gigault C, Dutcher JR. Molecular weight dependence of reductions in the glass transition temperature of thin, freely standing polymer films. Phys. Rev. E. 2001;63 . 031801.
- Rittigstein P, Torkelson JM. Polymer-nanoparticle interfacial interactions in polymer nanocomposites: confinement effects on glass transition temperature and suppression of physical aging. J. Polym. Sci. Polym. Phys. 2006;44:2935–2943.
- Claudy R, Letoffe JM, Camberlain Y, Pascault JR. Glass transition of polystyrene versus molecular weight. Polym. Bull. 1983;9:208–215.