Abstract
Polymer–metal complex nanoparticles-containing cisplatin (cis-dichlorodiammineplatinum(II)), an anticancer drug, was prepared by a ligand-exchange reaction of cisplatin with core-shell-type poly(citric acid)-b-poly(caprolactone)-b-poly(citric acid) (PCA–PCL–PCA). The resulting polymer–platinum complex nanoparticles showed a high loading capacity (up to 44.2% w/w). The TEM observation confirmed that the nanoparticles with spherical shape were formed by the mixing of PCA–PCL–PCA copolymer and cisplatin. According to TEM and DLS, polymer–cisplatin complex nanoparticles showed larger diameters compared with empty block copolymer attributed to some intermolecular crosslinkings through polymer–platinum (II) complex formation. The release profile of the platinum (II) complexes in phosphate-buffered saline medium at 37 °C indicated sustained manner of drug release. In vitro cytotoxicity evaluated by MTT assay, demonstrated that the PCA–PCL–PCA block copolymer does not exhibit apparent cytotoxicity. PCA–PCL–PCA–cisplatin complex nanoparticles showed greater cytotoxicity to HeLa cell line contrasted with free cisplatin, attributed to existence of poly (citric acid) moiety.
1. Introduction
Cisplatin is one of the most potent anticancer agents available today and is widely used in the treatment of many malignancies, including testicular, ovarian, bladder, head and neck, small cell and nonsmall cell lung cancers [1,2]Citation1Citation2 because of its potent activity to cross-link DNA upon entering the cells. It preferentially binds to the N7 atoms of guanine bases in DNA double-helix strands, thereby preventing the strands from uncoiling and separating. This prohibits the division of the cells and ultimately results in cellular apoptosis.[3–5]Citation3Citation4Citation5
However, its clinical use is limited due to its significant toxic side effects, such as acute nephrotoxicity and chronic neurotoxicity. cis-dichlorodiammineplatinum(II) (CDDP) shows a rapid distribution over the whole body and high glomerular clearance within 15 min after intravenous injection. A total of 90% of the cisplatin is bound to plasma proteins in the blood and, thus, does not enter the cells; leading to less therapeutic efficacy. Therefore, many efforts have been devoted to develop a DDS aimed at increasing the blood circulation period and accumulation in solid tumors.[6–8]Citation6Citation7Citation8
The association of cisplatin with long-circulating nano-sized carriers like polymer conjugates, liposomes, micelles and dendrimers alters drug pharmacokinetics and results in increased drug accumulation in tumors, based on the ‘enhanced permeability and retention’ (EPR) effect. The EPR effect is a result of leaky capillaries adjacent to solid tumors and a lack of a lymphatic system for the drainage of drugs back to the systemic circulation.[9–12]Citation9Citation10Citation11Citation12 Advantages of such nanocarriers lie in their ability to improve drug solubility, prolong systemic drug half-life, provide means for sustained and environmentally responsive drug release, enable tumor-specific delivery, reduce immunogenicity and systemic side effects, suppress development of drug resistance and deliver simultaneously two or more drugs for combination therapy.[13–16]Citation13Citation14Citation15Citation16
Polymeric micelles, which are self-assemblies of block copolymers, have gained increasing popularity as nanocarriers for chemotherapeutic agents since their critical features, including size and drug loading and release, can be modulated by engineering block copolymers. Polymeric micelles carrying chemotherapeutic agents like cisplatin can selectively and effectively accumulate in the solid tumors, thereby leading to enhanced antitumor activity.[17–20]Citation17Citation18Citation19Citation20 It was reported that the chloride ligands in cisplatin were substituted by a variety of ligand groups depending on the concentration of chloride ion in the surroundings. This ligand substitution is an important feature affecting its antitumor activity and toxic side effects. Particularly, when the chloride ligands in cisplatin are substituted with carboxylates, the newly formed carboxylic ligands are able to undergo further substitution due to fairly low nucleophilicity, the resultant complexes still show good antitumor activity. The property of carboxylate ligand as a good leaving group has been exploited to design cisplatin delivery systems based on carboxylate-containing polymers to achieve selective delivery to tumor sites with prolonged periods of cytotoxic action due to sustained release from carrier polymers through ligand-exchange reaction.[21–23]Citation21Citation22Citation23 Polymer–drug complex micelles were spontaneously formed on mixing of cisplatin with PEO-poly(aspartic acid) or PEO-poly(glutamic acid) block copolymers in an aqueous solution. The resultant micelles incorporating cisplatin were shown to have favorable pharmacokinetics, tumor-specific accumulation, improved antitumor activity and reduced nonspecific side effects, relative to the free drug.[6,24,25]Citation6Citation24Citation25 Polymer micelles with cross-linked ionic cores formed from poly (ethylene oxide)-b-poly methacrylate anions (PEO-b-PMA) were employed for the preparation of polymer–cisplatin conjugates. The drug content in the cross-linked micelles was determined to be 22% (w/w). Slow release of platinum complexes was observed in sustained manner from the cisplatin-loaded cross-linked micelles in physiological saline.Citation[26]
The metal–ligand coordination was also utilized to complex cisplatin to the hydrophilic poly acrylic acid blocks, with abundant carboxylic functional groups, in triblock copolymer micelles PEG-b-PCL-b-PAA. The size of the loaded micelles was about 13 nm, and the drug content was determined to be 10% (w/w).Citation[27] In other study, a core-shell-type star polymer consisting of hyperbranched polystyrene (hydrophobic core) and arms of poly(acrylic acid) (hydrophilic shell) was reported for cisplatin conjugation, in which hydrophilic shell with high density of functional groups – carboxylate ions was able to reversibly exchange ligands with cisplatin. The conjugates were exhibited high drug payload of 45% (w/w) and lower cytotoxicity compared with the free agent.Citation[23]
We have recently reported new nanosized amphiphilic ABA type poly(citric acid)-b-poly(caprolactone)-b-poly(citric acid) (PCA–PCL–PCA) block copolymers containing a linear polycaprolactone block (B) and a branched block bringing both hydrophilicity and carboxylic acid functional groups along the backbone, namely a poly (citric acid) block (A).Citation[28] These polymers seem to be a suitable carrier system for cisplatin, since these polymers are biodegradable and can be easily conjugated to platinum through the ligand-exchange reaction of cisplatin from the chloride to the carboxylate groups of poly(citric acid) blocks in an aqueous medium. It is hoped that the resultant conjugates can overcome the reduced uptake of cisplatin in cell lines by sequestering cisplatin (i.e. masking via the linkage process) and supplying the cell’s energy needs with the citric acid (a key component in the synthesis of adenosine triphosphate),Citation[29] utilized in the synthesis of the PCA–PCL–PCA copolymer. Therefore, we have designed a new cisplatin–polymeric conjugate based on PCA–PCL–PCA block copolymer aiming to afford high drug payload and controlled release of the active platinum moiety in vitro using these nanosized carriers. Here, we report synthesis, characterization, and therapeutic activity of the resultant conjugate in vitro toward HeLa cancer cell lines.
2 Experimental
2.1 Materials
The ϵ-caprolactone from Merck was dried over molecular sieves before use. 1,5-pentanediol from Merck was distilled under reduced pressure. Sn(II)2-ethylhexanoate [Sn(Oct)2] was purchased from Merck and used without further purification. Toluene from Merck was dried and distilled from metallic sodium and benzophenone. Anhydrous citric acid (Merck) was used as received. Tetrahydrofuran (THF), dichloromethane (CH2Cl2), and n-hexane (Merck) were dried before use. Pyrene (Merck) was recrystallized twice from absolute ethanol, and then, it was stored in dark and vacuum. cis-diamminedichloroplatinum(II) (cisplatin) was purchased from Aldrich Chemical Co., Inc., WI, USA. Phosphate-buffered saline (PBS) was purchased from Sigma. For in vitro cytotoxicity test, amniotic epithelial (AE) cells were obtained from elective Cesarean. HeLa cells were obtained from Pasteur Institute (Tehran, Iran). Dulbecco’s modified eagle’s medium (DMEM)/F12, RPMI 1640 and fetal bovine serum (FBS) were obtained from GIBCO Invitrogen Corporation. 3-(4,5 dimethylthiazol-2-yl)-2,5-diphenyl tetrazolium bromide (MTT), dimethyl sulfoxide (DMSO), and epidermal growth factor (EGF) were purchased from Sigma.
2.2 Synthesis of poly(citric acid)-block-polycaprolactone-block-poly(citric acid)
PCA–PCL–PCA was synthesized by a previously reported procedure Citation[28] as follows: firstly, poly(ϵ-caprolactone) was synthesized by ring-opening polymerization catalyzed by Sn(Oct)2. Predetermined amounts of ϵ-caprolactone and 1,5-pentanediol (co-initiator) and 0.02 mol% of Sn(Oct)2 were introduced into a preheated (150 °C) 50-mL three-neck round-bottomed flask. The reaction was carried out at 150 °C for 5 h under argon atmosphere. After cooling to room temperature, the obtained hydroxyl telechelic polycaprolactone was purified by dissolution in dichloromethane, filtration, two washes with acidified water, the removal of residual water with anhydrous MgSO4, and precipitation in n-hexane. Secondly, predetermined amounts of hydroxyl telechelic polycaprolactone and citric acid were added to a dried polymerization ampoule equipped with vacuum inlet. The flask was then immersed into an oil bath, and the temperature was gradually raised to 140–150 °C with continuous stirring to melt the mixture. Following melting, the mixture was polymerized at 140–150 °C for 2 h to create PCA–PCL–PCA copolymer. Vacuum valve was opened during this period to remove the water. Then, the polymerization ampoule was left at room temperature to cool down, and ampoule contents were dissolved in THF, and the product was precipitated in n-hexane. After washing with water for several times, the resulted polymer was collected and dried in a vacuum oven for 48 h. Table illustrates the feed ratio and composition of synthesized polymers.
Table 1. Molecular weights and compositions of synthesized polymers.
2.3 Preparation of cisplatin–polymeric conjugate
In a typical procedure, cisplatin was added to an aqueous solution of PCA–PCL–PCA block copolymer (1 mg/ml) at pH 8.5 at a molar ratio of cisplatin to carboxylate groups 1:1. The mixture was gently stirred for 48 h at room temperature under dark. Polymer–cisplatin conjugate was purified by dialysis against deionized water for 2 days using membrane with molecular weight cutoff size: 2000 and the concentration of unbound cisplatin was determined using inductively coupled plasma (ICP Integra XL) calibrated with platinum standard solutions. According to the methods reported previously,[24,30]Citation24Citation30 drug-loading content can be measured by determination of the unbound cisplatin concentration. After dialysis, the sample inside the dialysis bag was lyophilized and kept at −20 °C for further analyses. Amount of drug in polymer–cisplatin nanoparticles was determined based on the loading content measured by ICP.
The drug-loading content and the loading efficiency were calculated based on the following formulas:
2.4 In vitro drug release measurements
The release of the Pt(II) complexes from the polymeric carrier in phosphate-buffered saline (PBS, pH 7.4, 0.14 M NaCl) at 37 °C was evaluated by dialysis method using a membrane with molecular weight cutoff size: 2000. A measured amount of the polymer–cisplatin complex nanoparticles (10 mg) was dissolved in PBS (10 mL). The solution was transferred to the dialysis bag and dialyzed against PBS (200 ml). Aliquots of the solution (10 mL) outside of the dialysis bag were sampled at a defined time period, and fresh PBS solution of the same volume was added. The concentrations of released platinum present in the dialysate aliquots were determined using ICP spectrometer calibrated with platinum standard solutions. The concentration of Pt(II) released from the copolymer was expressed as a accumulative percentage of the total Pt(II) available and plotted as a function of time.
2.5 Cell culture
Human amniotic membrane was prepared using fresh human placenta as described previously.[31,32]Citation31Citation32 Briefly, the amnion was mechanically peeled of the chorion and washed several times with the PBS. To isolate the AE cells, the amniotic membrane was incubated at 37 °C with 0.15% trypsin-EDTA. Trypsin was inactivated with the FBS and the solution centrifuged at 2500 rpm for 12 min. Cells were washed with the PBS and cultured in the DMED/F12 containing 10% FBS, 10 ng/ml EGF, 2 mM L-glutamine, 1% nonessential amino acid, 55 μM 2-mercaptoethanol, 1 mM sodium pyruvate, and 100 U/ml penicillin/streptomycin solution. HeLa cells were cultured in 24-well plates at density of 5 × 104 per well in prepared RPMI (Chemicon) medium (20% FBS and 1% penicillin/streptomycin) and incubated at 37 °C with 5% CO2, about 48 h prior to adding the test substances.
2.6 In vitro cytotoxicity assay
Cytotoxicities of cisplatin–polymeric conjugate and free cisplatin were measured by MTT assay. The method is based on the biotransformation of the yellow tetrazolium dye MTT to a violet formazan product via the mitochondrial succinate dehydrogenase in viable cells. The Hela or AE cells were seeded at density of 5 × 104 per well in gelatin-coated 24-well plate and incubated overnight in incubator (37 °C, 5% CO2). The next day, AE cells were treated with blank PCA–PCL–PCA copolymer micelle at concentration of 1 mg/mL and incubated at temperature 37 °C for 24 h. HeLa cells were treated with copolymers, drug-loaded nanoparticles and free drug at concentration of 100 μg/mL and incubated at 37 °C for 24 h. The cultures without treatment were used as control group. For cytotoxicity assay, 40 μL of MTT solution (5 mg/mL) was added to each culture medium, and the plates were incubated for 4 h. The MTT formazan crystals were then dissolved with 1 ml DMSO at ambient temperature. The optical density (OD) was measured at 570 nm with a spectrophotometer (CE7500, Cecil, UK). The blank well containing only medium material was used for zero adjustment. The viable rate was calculated by the following equation:
where OD (control) was obtained in the absence of polymers, and OD (treated) was obtained in the presence of polymers. The data are given as mean diameter ± SD based on three independent measurements.
2.7 Characterization
The chemical structures of hydroxyl telechelic polycaprolactone and PCA–PCL–PCA copolymer were determined by using a proton nuclear magnetic resonance (NMR) instrument (Bruker spectrometer 400 MHz), employing tetramethylsilane as internal reference, in CDCl3 and d6-DMSO at 25 °C. The molecular weight and molecular weight distributions were determined by gel permeation chromatography (GPC) (Agilent 1100) with a PL-gel 10-μm column connected to a refractive index detector, with tetrahydrofuran as the mobile phase at the flow rate of 1 mL/min and 30 °C. Monodispersed polystyrene standards were used to generate the calibration curve. The critical micelle concentration (CMC) was determined fluorospectrometrically using pyrene as a fluorescence probe. Pyrene, a hydrophobic molecule, was preferentially distributed in the micelle core, causing changes in the photophysical properties. In short, a stock solution of the sample was first prepared at a concentration of 1 g/L and then diluted to different concentrations. Each sample was then prepared by mixing copolymer solution with a saturated aqueous solution of pyrene (12 × 10−7 mol/L) and stirring overnight in the dark. Fluorescence spectra of the samples were recorded with a Shimadzu spectro Fluorophotometer RF-540 (λem = 393 nm) at room temperature. The CMC was determined from the signal intensity ratio at 336 and 333 nm (I336/I333) vs. logarithm of concentration curve. Hydrodynamic mean diameter was determined at a 90° angle by dynamic laser scattering (DLS) using differential size distribution processor intensity analysis (Malvern Instruments, DTS Ver. 4.20, Serial Number: MAL1008078). Particles size was measured at 25 °C in water after filtration through a 0.22 mm pore size filter. Size measurements were taken in triplicate. The zeta potential was analyzed by laser Doppler anemometry using the same instrument in appropriate settings. Transmission electron microscopy (TEM) was carried out on a Philips CM 10 electron microscope, operating at an accelerating voltage of 100 kV. Specimens were prepared by dipping a copper grid (200 mesh) into aqueous solutions of copolymers at a concentration of 2 g/L. The grid was then left to stand on a piece of filter paper and air-dried before measurements. The morphology of polymers was observed by atomic force microscopy (AFM; C26-DME). The sample for AFM test was prepared by spin coating a drop of the cisplatin–polymer complex solution onto silicon wafer. The released Pt from cisplatin–polymeric conjugate was measured by an inductively coupled plasma spectrometer (ICP Integra XL).
3 Results and discussion
The synthesis of PCA-b-PCL-b-PCA amphiphilic triblock copolymer was carried out by two steps as reported previously Citation[28]. Firstly, the hydroxyl telechelic poly (ϵ-caprolactone) was synthesized by a ring opening polymerization of ϵ-caprolactone in which 1,5-pentanediol was used as the co-initiator and Sn(Oct)2 as the catalyst. Secondly, PCA-b-PCL-b-PCA was obtained by melt polycondensation reaction of citric acid and hydroxyl telechelic poly(ϵ-caprolactone). In the copolymer synthesis, polycaprolactone reacts with the excess amounts of citric acid molecules at 140–150 °C. In this temperature range, (below melting temperature of citric acid), polycaprolactone is molten and can act as reaction phase. Similar structures have been reported previously by the reaction of polyethylene glycol and excess amounts of citric acid molecules.Citation[33] The condensation reaction occurs between terminal hydroxyl groups of polycaprolactone chains and carboxylic acid group of citric acid molecules. Therefore, polycaprolactone chain bears two citric acid units at the chain ends, containing carboxylic acid functional groups. Then, polycondensation reaction was carried out between carboxylic acid groups attached to the polymer chain end and hydroxyl groups of citric acid molecules to create a branched structure named poly(citric acid) block. The resulted copolymers are fully soluble in THF, DMF, and DMSO. Some aggregates shown in TEM image can be attributed to the drying process during TEM measurements. The incorporation of citric acid blocks into the polycaprolactone chain ends was confirmed by the elimination of resonance from the hydroxyl terminations of PCL at 3.6 ppm and appearance of a new resonance from the CH2 of citryl units located in terminal groups of CH2–COOH at 2.6–3 ppm in the 1H NMR spectrum of copolymer. Compositions of the block copolymers were determined by 1H NMR in d6-DMSO from peak intensity ratios of methylene protons of terminal citryl units (CH2–COOH: a quartet at 2.62–2.77 ppm) and methylene protons next to the oxygen of PCL chain (CH2–O: a triplet at 4 ppm). From 1H NMR of PCA-b-PCL-b-PCA in D2O, complete loss of characteristic resonance originating from CL was found due to the suppressed molecular motion of the aggregated hydrophobic PCL chains in the inner cores which offered one of the evidences that PCA–PCL–PCA copolymers can associate to form micellar structure in the water.Citation[28] It was proposed that the micelle core was composed of PCL, and the PCA blocks were placed in the outer shell of the micelles due to its excellent flexibility in water at room temperature. GPC showed Mn value of 3300 g/mol for PCL segment and 7500 g/mol for PCA–PCL–PCA copolymer, and the results of H NMR and GPC indicated the PCA content of about 55%w in the block copolymer structure (Table ).
Cisplatin was coordinated poly (citric acid) segments of PCA–PCL–PCA copolymer by substituting its chloride ligands with carboxylate ligands through mixing of drug with aqueous copolymer solution. Figure illustrates the proposed structures for PCA–PCL–PCA–cisplatin complex. The complexation provides potentially interaction between cisplatin and polymer that reduce the burst-effect, prolong the release of cisplatin and further reduce the systemic toxicity of cisplatin.Citation[34] As reported previously, carboxylic acid is exchanged with water or chloride forming aqua-platinum and chloroplatinum, respectively. The absolute exchange rate of carboxylic groups with water molecules is slow due to the more favorable carboxylate interaction and multivalency of the carboxylate polymer–platinum interaction. The appropriate conditions for cisplatin immobilization in terms of pH, concentration of cisplatin, and molar ratio of cisplatin to carboxylate groups were selected based on a few preliminary experiments and on the results reported by other researchers working in this field.[21,23,24,26]Citation21Citation23Citation24Citation26 At pH value of 8.5, ionization of the carboxylic functions in poly (citric acid) blocks occurred, and cisplatin was complexed with poly(citric acid) residues in PCA–PCL–PCA copolymer. Polymer–platinum (II) complex nanoparticles thus prepared was purified by dialysis method, and the concentration of unbound cisplatin was determined using inductively coupled plasma (ICP). Unbound cisplatin rapidly reached equilibrium with the dialysis medium, while the absence of chloride ions prevented release of chemically attached cisplatin. In this case, 47% of the initial drug concentration was found to release over 2 days. Therefore, based on the ICP data, the loading efficiency of cisplatin in PCA–PCL–PCA was determined to be approximately 53% of initial drug load at pH 8.5. This was due to the cisplatin being able to effectively interact with the carboxylate ions of the polymer when the experimental condition of pH 8.5 was employed. By measuring the loaded drug concentration using ICP, the corresponding amount of the immobilized cisplatin at pH 8.5 was determined to be 44.2% of the mass of the loaded particles. From a pharmaceutical point of view, such a remarkably high capacity of drug loading might be considered as a valuable precondition for the development of a cisplatin delivery system.
Generally, micelle formation occurs as a result of two forces. One is an attractive force that leads to the association of molecules, while the other one is a repulsive force that prevents unlimited growth of the micelles. Therefore, the micelle size is mainly determined by the relative magnitude and balance of these two forces.Citation[35] As shown in Figure , formed polymer–platinum (II) complex nanoparticles had a unimodal size distributed with the particle size around 200 nm. Furthermore, according to the TEM observation, the PCA–PCL–PCA–cisplatin nanoparticles have spherical shapes with a particle size of around 45 nm, as shown in Figure . The size of the particles is smaller than that determined by DLS in water, presumably arising from the dehydration and shrinkage of the micelles during drying in the TEM measurement.Citation[36]
Figure 2 Particle size distribution of PCA–PCL–PCA–cisplatin nanoparticles at 25 °C in water measured by DLS.
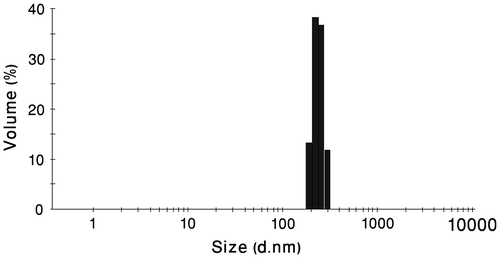
As can be observed from Table , upon formation of polymer–platinum (II) complex nanoparticles, an increase in the particles size was seen as contrasted with the blank PCA–PCL–PCA micelle, reported previously.Citation[28] This phenomenon can be explained by intermolecular crosslinking through the formation of –COO–Pt–OOC– bridges between two different copolymer molecules Citation[23] as illustrated in structure B of Figure . Intermolecular crosslinking is also confirmed by TEM image that demonstrates the aggregation tendency of nanoparticles. Also, the observed aggregates may be caused from drying process during TEM measurements. As shown in Figure , the AFM result shows clearly that the nanoparticles are spherical with a number-averaged height of 8 nm and a diameter of about 100 nm. However, the values are smaller than the result (200 nm) measured by DLS (shown in Figure ), because the particles were shrunken for AFM measurements.Citation[37]
Table 2. Parameters of the prepared nanoparticles.
Formulation stability is of key importance, and zeta potential measurements are instrumental in developing stable dispersions. High absolute value of the zeta potential suggests high surface charge of the nanoparticles, which leads to strong repellent interactions among the nanoparticles in dispersion and thus high stability.Citation[38] The surface zeta potential of the blank PCA–PCL–PCA nanoparticles was −48 mV (Figure ). We further found that the surface zeta potential of the polymer–platinum (II) complex nanoparticles had a decrease to −20.2 mV (Figure ). It was suggested that conjugation of cisplatin to the PCA–PCL–PCA copolymer led to decrease in the surface negative charge of the polymer nanoparticles due to neutralization of the PCA segments resulting from the binding of cisplatin to carboxylate groups. To monitor the formation of PCA–PCL–PCA–cisplatin complex micelles in aqueous media, we used fluorescence spectrophotometer to measure the fluorescence intensity ratio of pyrene probe.
Figure 5 (a) Surface zeta potential of blank PCA–PCL–PCA micelles measured by DLS. (b) Surface zeta potential of PCA–PCL–PCA–cisplatin nanoparticles measured by DLS.
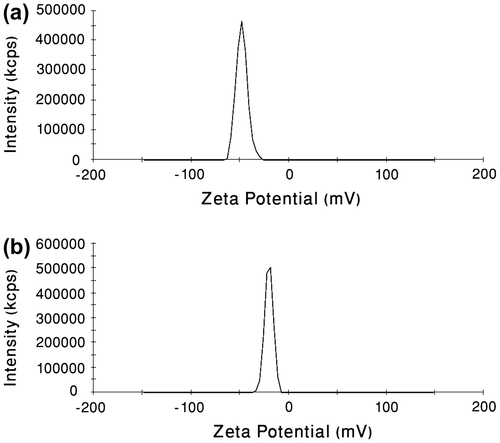
Polymeric micelles can be formed only when the polymer concentration is higher than the CMC which characterizes the micelle stability. Steady-state fluorescence, using pyrene as hydrophobic fluorescence probe, is well used as technique to show the formation of micelles. The polarity of the surrounding environment of the probe molecules affects some vibrational bands in the fluorescence excitation spectrum. It has been shown previously that with increasing concentrations of amphiphilic polymers in aqueous pyrene solution, there is a shift of the (0, 0) band from 333 to 336 nm in the excitation spectra of pyrene.[39,40]Citation39Citation40 The changes in the relative intensity of the first and the third vibrational bands (I336/I333) have been suggested to examine the polarity of the microenvironment. The CMC of micelles can be determined by this method. After micellar formation, pyrene partitions into both the micellar phase and the water phase. Since the core of the micelle is hydrophobic, the intensity ratio of I336/I333 is changed. The extrapolation of tangent of the major change in the slope of the fluorescence intensity ratio leads to CMC.Citation[41] As illustrated in Figure , polymer–platinum (II) complex micelle exhibits a CMC about 3.4 × 10−2 mg/mL.
Figure 6 (a) Excitation spectra of pyrene as a function of copolymer concentration at room temperature in water (λem = 393) for PCA–PCL–PCA–cisplatin. (b) Plot of the intensity ratio I336/I333 (from pyrene excitation spectra) vs. log (C) for PCA–PCL–PCA–cisplatin.
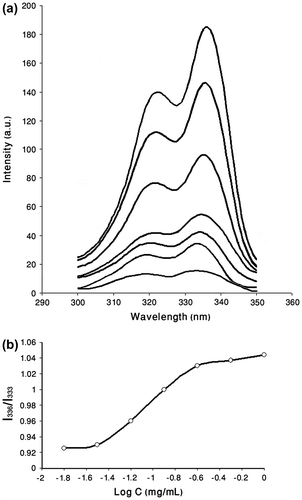
In vitro release of cisplatin from polymer–platinum (II) complex nanoparticles was carried out by dialysis method in phosphate-buffered saline (PBS, pH 7.4, 0.14 M NaCl) at 37 °C. Cisplatin solubility in the PBS medium was 1 mg/mL confirming the maintenance of sink conditions during the release experiments given the release volume (200 mL) and drug amounts in the nanoparticles (4.4 mg drug/10 mg drug-loaded nanoparticles determined by ICP). As shown in Figure , release of Pt (II) complexes was observed in sustained manner from polymer–platinum (II) complex nanoparticles. Only 52% of total cisplatin loading was released over 3 d in PBS, implying the PCA–PCL–PCA–cisplatin complex nanoparticles might be useful as a slow release system for this anticancer drug. This controlled release of cisplatin in neutral condition is very important in preserving a stable bond until reaching the target site (tumor) and therefore prevents unwanted and nonspecific toxicities resulting from out of place cisplatin release in the blood circulation. Nearly, no CDDP was released in distilled water, indicating that the presence of chloride ions in the physiological solution was essential for the release process, affording conditions for the ligand substitution of cisplatin from the carboxyls of PCA blocks. For the drugs that are chemically linkaged into the polymer carrier the mechanism of release is multistep process.Citation[42] In this case, it should involve, first, the penetration of chloride ions into the membrane-containing PCA–PCL–PCA–cisplatin, followed by exchange between chloride ions and carboxylic groups in Pt(II) complexes, and finally, diffusion of Pt(II) species out of the complexes. It was pointed earlier [22,24,26]Citation22Citation24Citation26 that the presence of chloride ions in the physiological solution was essential for the release process, affording conditions for the inverse ligand substitution reaction. The formation of aqua or hydroxo platinum (II) complexes is also possible. As shown in the release profile, only 52% of total cisplatin loading was released over 3 d in PBS, indicating that cisplatin had formed strongly bound complexes with the polymeric ligand. Platinum complexes may be bound to one or two carboxylate units on the PCA–PCL–PCA copolymer. Two carboxylate ligands would form the strongest bound complexes as illustrated in Figure . The slow release of Pt(II) in PCA–PCL–PCA–cisplatin system can be corresponded to theses strong bonds, which prevents the complete release of Pt(II) species over 3 d in PBS.
Figure 7 Cisplatin release profile from PCA–PCL–PCA–cisplatin nanoparticles in phosphate-buffered saline (PBS, pH 7.4, 0.14 M NaCl) at 37 °C.
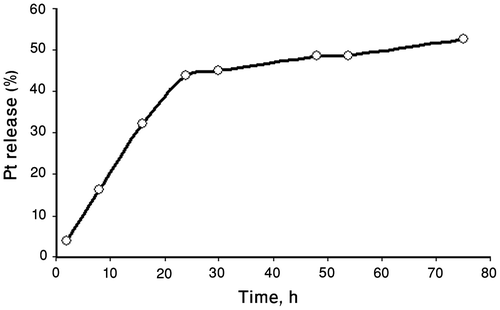
Biocompatibility is a vital necessity of polymer materials using for drug delivery applications. In this study, we performed preliminary evaluation on the cytotoxicity of the blank PCA–PCL–PCA copolymer against normal cells (here the AE cells) by the MTT assay. As shown in Figure , the viability of the AE cells after 24-h incubation with block copolymers up to 1 mg/mL has little changes compared with the untreated cells. This result demonstrates that the PCA–PCL–PCA block copolymer does not exhibit apparent cytotoxicity.
The in vitro cytotoxicity of PCA–PCL–PCA–cisplatin nanoparticles was evaluated with HeLa cell lines by MTT assay. Figure illustrates the growth inhibition of HeLa cells by PCA–PCL–PCA–cisplatin nanoparticles and free cisplatin at 37 °C, showing the effects of treatments with PCA–PCL–PCA–cisplatin nanoparticles on the growth of HeLa cells after 24 h. The results demonstrated that PCA–PCL–PCA–cisplatin nanoparticles showed higher cytotoxicity than free cisplatin. The cell viability of free cisplatin was 47%, after 24 h of incubation at a drug concentration of 100 μg/mL. By contrast, cell viability was around 33%, when the cells were treated with PCA–PCL–PCA–cisplatin at the concentration of 100 μg/mL (containing 44 μg/mL of drug determined by ICP). On the other word, drug–polymer nanoparticles with 44 μg/mL of drug content had cellular cytotixicity against Hela cancer cells higher than 100 μg/mL free cisplatin. This can be attributed to the higher uptake of PCA–PCL–PCA–cisplatin by HeLa cells, contrasted with the free cisplatin, due to the poly (citric acid) moiety of the nanoparticles together with the greater demands of cancer cells for such energy sources which is a prerequisite for growth during the time of incubation. Similar reasons have been proposed for some polymer–drug conjugates thus far.Citation[29] (Noted here, the empty micelles do not exhibit apparent cytotoxicity at the same time.)
4 Conclusion
Polymer–platinum (II) complex nanoparticles were fabricated from PCA–PCL–PCA and cisplatin. The carboxylate groups of branched poly (citric acid) shell allows for formation of complex by ligand-exchange reaction with cisplatin. A high drug payload of 45%w was achieved, determined by ICP. As demonstrated with DLS and TEM, the particle size of polymer–cisplatin complex nanoparticles was larger than blank PCA–PCL–PCA micelles, attributed to intermolecular crosslinking through the formation of –COO–Pt–OOC– bridges between two different copolymer molecules. Slow release of platinum complexes was observed in sustained manner from the PCA–PCL–PCA–cisplatin complex nanoparticles in phosphate-buffered saline. The blank PCA–PCL–PCA micelles exhibited no apparent cytotoxicity to the cultured cells. Compared with free cisplatin, PCA–PCL–PCA–cisplatin complex nanoparticles showed increased cytotoxicity to HeLa cell line attributed to poly (citric acid) moiety and greater demands of cancer cells to access this molecule for the supply of energy which is a prerequisite for growth. All these confirm that PCA–PCL–PCA–cisplatin complex nanoparticles may offer a very promising approach for cancer therapy.
Acknowledgments
The financial support of University of Tabriz is gratefully acknowledged.
References
- Mattheolabakis G, Taoufik E, Haralambous S, Roberts ML, Avgoustakis K. In vivo investigation of tolerance and antitumor activity of cisplatin-loaded PLGA-mPEG nanoparticles. Eur. J. Pharm. Biopharm. 2009;71:190–195.
- Boulikas T, Vougiouka M. Recent clinical trials using cisplatin, carboplatin and their combination chemotherapy drugs. Oncol. Rep. 2004;11:559–595.
- Aryal S, Hu CMJ, Zhang L. Polymer–cisplatin conjugate nanoparticles for acid-responsive drug delivery. ACS Nano. 2010;4:251–258.
- Wang D, Lippard SJ. Cellular processing of platinum anticancer drugs. Nat. Rev. Drug Discovery. 2005;4:307–320.
- Galanski M, Arion VB, Jakupec MA, Keppler BK. Recent developments in the field of tumor-inhibiting metal complexes. Curr. Pharm. Des. 2003;9:2078–2089.
- Osada K, Christie RJ, Kataoka K. Polymeric micelles from poly(ethylene glycol)–poly(amino acid) block copolymer for drug and gene delivery. J. R. Soc. Interface. 2009;6:S325–S339.
- Casolaro M, Cini R, Del Bello B, Ferrali M, Maellaro E. Cisplatin/hydrogel complex in cancer therapy. Biomacromolecules. 2009;10:944–949.
- Gianasi E, Wasil M, Evagorou EG, Keddle A, Wilson G, Duncan R. HPMA copolymer platinates as novel antitumour agents: in vitro properties, pharmacokinetics and antitumour activity in vivo. Eur. J. Cancer. 1999;35:994–1002.
- Gryparis EC, Hatziapostolou M, Papadimitriou E, Avgoustakis K. Anticancer activity of cisplatin-loaded PLGA-mPEG nanoparticles on LNCaP prostate cancer cells. Eur. J. Pharm. Biopharm. 2007;67:1–8.
- Kim J, Yoon HJ, Kim S, Wang K, Ishii T, Kim YR, Jang WD. Polymer–metal complex micelles for the combination of sustained drug releasing and photodynamic therapy. J. Mater. Chem. 2009;19:4627–4631.
- Xiao H, Zhou D, Liu S, Zheng Y, Huang Y, Jing X. A complex of cyclohexane-1,2-diaminoplatinum with an amphiphilic biodegradable polymer with pendant carboxyl groups. Acta Biomater. 2012;8:1859–1868.
- Li X, Li R, Qian X, Ding Y, Tu Y, Guo R, Hu Y, Jiang X, Guo W, Liu B. Superior antitumor efficiency of cisplatin-loaded nanoparticles by intratumoral delivery with decreased tumor metabolism rate. Eur. J. Pharm. Biopharm. 2008;70:726–734.
- Oberoi HS, Laquer FC, Marky LA, Kabanov AV, Bronich TK. Core cross-linked block ionomer micelles as pH-responsive carriers for cis-diamminedichloroplatinum(II). J. Controlled Release. 2011;153:64–72.
- Zhang L, Gu FX, Chan JM, Wang AZ, Langer RS, Farokhzad OC. Nanoparticles in medicine: therapeutic applications and developments. Clin. Pharmacol. Ther. 2008;83:761–769.
- Zhu W, Li Y, Liu L, Chen Y, Xi F. Supramolecular hydrogels as a universal scaffold for stepwise delivering Dox and Dox/cisplatin loaded block copolymer micelles. Int. J. Pharm. 2012;437:11–19.
- Davis ME, Chen ZG, Shin DM. Nanoparticle therapeutics: an emerging treatment modality for cancer. Nat. Rev. Drug Discovery. 2008;7:771–782.
- Kulthe SS, Choudhari YM, Inamdar NN, Mourya V. Polymeric micelles: authoritative aspects for drug delivery. Des. Monomers Polym. 2012;15:465–521.
- Baba M, Matsumoto Y, Kashio A, Cabral H, Nishiyama N, Kataoka K, Yamasoba T. Micellization of cisplatin (NC-6004) reduces its ototoxicity in guinea pigs. J. Controlled Release. 2012;157:112–117.
- Xiong XB, Binkhathlan Z, Molavi O, Lavasanifar A. Amphiphilic block co-polymers: preparation and application in nanodrug and gene delivery. Acta Biomater. 2012;8:2017–2033.
- Kim JH, Li Y, Kim MS, Kang SW, Jeong JH, Lee DS. Synthesis and evaluation of biotin-conjugated pH-responsive polymeric micelles as drug carriers. Int. J. Pharm. 2012;427:435–442.
- Wang C, Gong Y, Fan N, Liu S, Luo S, Yu J, Huang J. Fabrication of polymer-platinum(II) complex nanomicelle from mPEG-g-α, β-poly[(N-amino acidyl)-DL-aspartamide] and cis-dichlorodiammine platinum(II) and its cytotoxicity. Colloids Surf., B. 2009;70:84–90.
- Nishiyama N, Yokoyama M, Aoyagi T, Okano T, Sakurai Y, Kataoka K. Preparation and characterization of self-assembled polymer-metal complex micelle from cis-dichlorodiammineplatinum(II) and poly(ethylene glycol)-poly(α, β-aspartic acid) block copolymer in an aqueous medium. Langmuir. 1999;15:377–383.
- Kowalczuk A, Stoyanova E, Mitova V, Shestakova P, Momekov G, Momekova D, Koseva N. Star-shaped nano-conjugates of cisplatin with high drug payload. Int. J. Pharm. 2011;404:220–230.
- Nishiyama N, Kataoka K. Preparation and characterization of size-controlled polymeric micelle containing cis- dichlorodiammineplatinum(II) in the core. J. Controlled Release. 2001;74:83–94.
- Nishiyama N, Okazaki S, Cabral H, Miyamoto M, Kato Y, Sugiyama Y, Nishio K, Matsumura Y, Kataoka K. Novel cisplatin-incorporated polymeric micelles can eradicate solid tumors in mice. Cancer Res. 2003;63:8977–8983.
- Bontha S, Kabanov AV, Bronich TK. Polymer micelles with cross-linked ionic cores for delivery of anticancer drugs. J. Controlled Release. 2006;114:163–174.
- Zhu W, Li Y, Liu L, Zhang W, Chen Y, Xi F. Biamphiphilic triblock copolymer micelles as a multifunctional platform for anticancer drug delivery. J. Biomed. Mater. Res. 2011;96A:330–340.
- Gheybi H, Entezami AA. Nanosized micelles self-assembled from amphiphilic poly(citric acid)–poly(ϵ-caprolactone)–poly(citric acid) copolymers. Polym. Bull. 2013;70:1875–1894.
- Haririan I. Shafiee Alavidjeh M, Khorramizadeh MR, Shafiee Ardestani M, Zarei Ghane Z, Namazi H. Anionic linear-globular dendrimer-cis-platinum (II) conjugates promote cytotoxicity in vitro against different cancer cell lines. Int. J. Nanomed. 2010;5:63–75.
- Haxton KJ, Burt HM. Hyperbranched polymers for controlled release of cisplatin. Dalton Trans. 2008; 5872–5875.
- Nabid MR, Rezaei SJT, Sedghi R, Niknejad H, Entezami AA, Oskooie HA, Heravi MM. Self-assembled micelles of well-defined pentaerythritol-centered amphiphilic A4B8 star-block copolymers based on PCL and PEG for hydrophobic drug delivery. Polymer. 2011;52:2799–2809.
- Rezaei SJT, Nabid MR, Niknejad H, Entezami AA. Multifunctional and thermoresponsive unimolecular micelles for tumor-targeted delivery and site-specifically release of anticancer drugs. Polymer. 2012;53:3485–3497.
- Naeini AT, Adeli M, Vossoughi M. Poly(citric acid)-block-poly(ethylene glycol) copolymers–new biocompatible hybrid materials for nanomedicine. Nanomed. Nanotechnol. Biol. Med. 2010;6:556–562.
- Yan X, Gemeinhart RA. Cisplatin delivery from poly(acrylic acid-co-methyl methacrylate) microparticles. J. Controlled Release. 2005;106:198–208.
- Lee J, Cho EC, Cho K. Incorporation and release behavior of hydrophobic drug in functionalized poly(D, L-lactide)-block-poly(ethylene oxide) micelles. J. Controlled Release. 2004;94:323–335.
- Yang L, Zhao Z, Wei J, El Ghzaoui A, Li S. Micelles formed by self-assembling of polylactide/poly(ethylene glycol) block copolymers in aqueous solutions. J. Colloid Interface Sci. 2007;314:470–477.
- Wang H, Chen X, Pan C. Linear poly(ethylenimine)-graft-poly(ethylene glycol) copolymers: Their micellization and secondary assembly. J. Colloid Interface Sci. 2008;320:62–69.
- Ma Y, Huang L, Song C, Zeng X, Liu G, Mei L. Nanoparticle formulation of poly(ϵ-caprolactone-co-lactide)-D-α-tocopheryl polyethylene glycol 1000 succinate random copolymer for cervical cancer treatment. Polymer. 2010;51:5952–5959.
- Benahmed A, Ranger M, Leroux JC. Novel polymeric micelles based on the amphiphilic diblock copolymer poly(n-vinyl-2-pyrrolidone)-block-poly(D, L-lactide). Pharm. Res. 2001;18:323–328.
- Bian Q, Xiao Y, Lang M. Thermoresponsive biotinylated star amphiphilic block copolymer: synthesis, self-assembly, and specific target recognition. Polymer. 2012;53:1684–1693.
- Luo L, Ranger M, Lessard DG, Le Garrec D, Gori S, Leroux JC, Rimmer S, Smith D. Novel amphiphilic diblock copolymer of low molecular weight poly(N-vinylpyrrolidone)-block-poly(D, L-lactide): synthesis, characterization, and micellization. Macromolecules. 2004;37:4008–4013.
- Kim JO, Nukolova NV, Oberoi HS, Kabanov AV, Bronich TK. Block ionomer complex micelles with cross-linked cores for drug delivery. Polym. Sci. Ser. A. 2009;51:708–718.