Abstract
A new dialdehyde-containing silicon was prepared by a reaction of Williamson-type and structurally characterized. By its subsequent condensation with 2,5-bis(p-aminophenyl)-1,3,4-oxadiazole, a multifunctional polyazomethine-containing imine, phenylene, oxadiazole, ether, and carbosilane groups within the chain was prepared. The complexes of this polymer with Co(II), Cu(II), and Zn(II) ions formed on the basis of electron-donor ability of the oxadiazole and azomethine groups were prepared in a one-step approach by mixing the two precursors of polyazomethine (dialdehyde and diamine) with corresponding metal salt. The ligand and metal complexes were characterized by spectral (Fourier transform infrared (FTIR), 1H, and 13C NMR) methods. The thermal, optical, electrochemical, and dielectric properties were studied and the effects of the metal insertion in dependence on its nature over these properties were evaluated. All compounds are soluble in common organic solvents and show low glass transitions due to the flexible ether and Si–C bonds, are fluorescent, mainly due to the oxadiazole ring, are redox active and have high dielectric constant due to the presence of oxadiazole, azomethine metal units.
1. Introduction
Polyazomethines are a well-known class of polymers formed by condensation of diamines with dicarbonylic (dialdehydes or diketones) compounds. Polyazomethines exhibit interesting properties mainly derived from the presence of imine groups and conjugation occurring within the backbone (especially in the case of the aromatic ones): good thermal stability, mechanical strength, non-linear optical properties, semiconducting properties, environmental stability, and fiber-forming properties but also ability to form mesophases on heating or to chelate metal ions.[Citation1–Citation4] Moreover, polyazomethines as thermostable polymers are interesting candidates for the potential application in electronics, optoelectronics, and photonics.[Citation5,Citation6] Therefore, they were intensely explored and there is in literature an extremely high number of articles about polyazomethines, as well as metallic complexes derived from them. Combinations of different types of the bifunctional co-monomers having proper reactive groups have been used to design the desired polyazomethine structures (fully aromatic, aliphatic or mixed) or to confer them some wanted properties. Thus, polyazomethines in which phenylene rings were replaced with heterocycles, such as furan, thiophene, 1,3,4-thiadiazole, 1,3,4-oxadiazole, etc., synthesized from the diamines or dialdehydes containing these nuclei,[Citation2,Citation7,Citation8] have proven to have high aromatic character and extensive conjugation in the main chain. The thermo-oxidative stability or electric conductivity of these polyazomethines are comparable with those of fully aromatic systems or higher.[Citation9] Most of heterocycles are chromophores. One of them, 1,3,4-oxadiazole, for example, proved to confer to the systems in which it is incorporated good resistance in oxidative atmosphere and hydrolytic stability, improved mechanical properties, and blue light emission. The high polarizability of this cycle creates the premise for increasing dielectric constant of the derived compounds or materials.[Citation10,Citation11] Materials containing 1,3,4-oxadiazole ring, known for its electron-withdrawing character, are of high interest for using in opto-electronic devices.[Citation7,Citation12–Citation14] However, in spite of their properties of interest (semiconductivity, high thermal stability, etc.), conjugated polyazomethines-containing 1,3,4-oxadiazole rings present the disadvantage of low solubility.[Citation7,Citation9,Citation15] There are some possibilities to improve this behavior of the polyazomethines, one of the approaches consisting in the insertion of the bulky groups or flexible moieties either within the chain or in lateral position obstructing packaging polymer chains. We have previously published a number of studies on the synthesis and study of polyazomethines-containing flexible dimethylsiloxane fragments coming either from amine [Citation16–Citation18] or carbonyl [Citation19,Citation20] derivatives and some of their metal complexes.
In this paper, we chose to prepare an original polyazomethine containing both phenylene and oxadiazole rings within the chain spaced by imine and relatively flexible bis(ether)carbosilane groups. For this, we have gone from a completely new aldehyde, bis(formyl-p-phenoxymethyl)-dimethylsilane prepared by us and the already known diamine 2,5-bis(p-aminophenyl)-1,3,4-oxadiazole. It is expected that, in addition to the properties that might be induced by the presence of polar groups (azomethine, oxadiazole, and phenylene), e.g. redox activity, optical, and dielectric properties, etc., the insertion of dimehylsilane groups, known for their non-polar nature and inability to be involved in the formation of hydrogen bonds, to improve the solubility of the polyazomethine through disrupting the packing of the chains.[Citation21] This, as well as the slight flexibility of the Si–C and etheric C–O bond should also reduce the glass transition of the polyazomethine below the decomposition temperature allowing their thermal processing.[Citation11,Citation22] The derived complexes of this polyazomethine with Co(II), Cu(II), and Zn(II) ions were prepared in a one-step approach by mixing the two precursors of polyazomethine (dialdehyde and diamine) with corresponding metal salt. The ligand and metal complexes were deeply characterized and investigated from point of view of thermal, optical, redox properties, and electrical behaviors.
2. Experimental
2.1. Materials
2,5-bis(p-aminophenyl)-1,3,4-oxadiazole was prepared according to a published procedure [Citation7,Citation21,Citation23,Citation24] consisting in the reaction of p-aminobenzoic acid with hydrazine hydrate in polyphosphoric acid. Yield: 90%. mp = 260–262 °C, Fourier transform infrared (FTIR) νmax (KBr), cm−1: 3468w, 316w, 3321m, 3202m, 3036vw, 1622s, 1609vs, 1587s, 1558m, 1493vs, 1437s, 1371w, 1308s, 1296m, 1269m, 1178s, 1132w, 1107w, 1078m, 1011w, 991vw, 964w, 829m, 744m, 704m, 690w, 646m, 606w, 538w, 509w, 436vw, 393w (Figure 1S). 1H NMR (DMSO-d6, 400 MHz, ppm): δ 7.73–7.71 (d, 4H, H4, H4′); 6.71–6.68 (d, 4H, H3, H3′); 5.87 (s, 4H, NH2) (Figure 2S); 13C NMR (DMSO-d6, 400 MHz, ppm): δ 163.36 (C2), 151.93 (C6), 127.76 (C4, C4′), 113.54 (C3, C3′), 110.19 (C5) (Figure 3S). Single crystals of 2,5-bis(p-aminophenyl)-1,3,4-oxadiazole were obtained by recrystallization from DMF/THF/CHCl3 solvents mixture and the structure was confirmed by X-ray single crystal diffraction, the fitting parameters being those reported in CCDC data basis – CCDC 246608 [C14H12N4O, Space group Pbca, cell dimensions: a = 13.461(5), b = 7.937(3), c = 22.816(8), Volume: 2437.5(14)].[Citation25]
Bis(chloromethyl)dimethylsilane, (CH3)2Si(CH2)2Cl2, Fluka, purum, ≥97.0% (GC), bp = 159–160 °C, density = 1.075 g/ml.
p-Hydroxybenzaldehyde, 98%, mp 112–116 °C, Sigma–Aldrich.
Copper chloride dihydrate, CuCl2·2H2O, purity ≥ 98%, Sigma–Aldrich.
Cobalt chloride hexahydrate, CoCl2·6H2O, purity ≥ 98%, Sigma–Aldrich.
Zinc chloride, ZnCl2-anhydrous, purity 99.99%, Sigma–Aldrich.
Solvents: methanol, ethanol, tetrahydrofuran, dimethylformamide (DMF), and chloroform (Chemical Company).
2.2. Measurements
FTIR spectra were recorded using a Bruker Vertex 70 FTIR spectrometer. Registrations were performed in the transmission mode in the range 400–4000 cm−1 at room temperature with a resolution of 2 cm−1 and accumulation of 32 scans.
Far-IR spectra were recorded using a Bruker Vertex 70 FTIR spectrometer equipped with a FIR source, CsI beamsplitter, and standard DLaTGS detector. Registrations were performed in the transmission mode in the range 180–700 cm−1 at room temperature with a resolution of 2 cm−1 and accumulation of 64 scans.
The proton magnetic resonance (1H NMR) spectra were acquired in CDCl3 and DMSO-d6 at 25 °C with a Bruker Avance DRX 400 MHz spectrometer operating at 400.13 MHz for 1H. The spectrometer was equipped with a 5 mm four nuclei, direct detection z-gradient probe head. Chemical shifts are reported in ppm and are referenced to chloroform δ1H = 7.26 ppm and to dimethylsulfoxide (DMSO) δ1H = 2.51 ppm.
GPC measurements were made in DMF on a PL-EMD 950 Chromatograph – Evaporative Mass Detector. The calibration was performed with polystyrene standards.
The presence and ratio of metal and Si were evidenced using an Energy-Dispersive X-ray Fluorescence system EX-2600 X-Calibur SDD.
The electronic absorption spectra were measured with an Analytic Jena SPECORD 200 spectrophotometer in 10 mm optical path quartz cells fitted with polytetrafluoroethylene stoppers.
Fluorescence spectra were obtained by using a Perkin Elmer LS55 luminescence spectrometer in solution or in solid state.
Thermogravimetric measurements were conducted on a STA 449 F1 Jupiter device (Netzsch, Germany). About 10 mg of each sample was weighed and heated in alumina crucibles. Nitrogen was purged as inert atmosphere at a flow rate of 50 mL min−1. Samples were heated in the temperature range from 30 to 700 °C at a heating rate of 10 °C min−1.
Differential scanning calorimetry (DSC) measurements were conducted on a DSC 200 F3 Maia device (Netzsch, Germany). A mass of 10 mg of each sample was heated in pressed and pierced aluminum crucibles at a heating rate of 10 °C min−1. Nitrogen was used as inert atmosphere at a flow rate of 50 mL min−1. The temperature against heat flow was recorded. The baseline was obtained by scanning the temperature domain of the experiments with an empty pan. Temperature and sensitivity calibrations were performed with five different metals at various heating rates according to standard procedures.
Cyclic voltammetry (CV) was performed on a Bioanalytical System, Potentiostat-Galvanostat (BAS 100B/W). The electrochemical cell was equipped with three electrodes: a Pt working electrode (plate with 0.5 × 0.5 cm2 area), an auxiliary electrode (platinum wire), and a reference electrode (silver wire coated with AgCl). All potentials were reported with respect to Ag/AgCl electrode at room temperature under a nitrogen atmosphere. Ferrocene was used as an external reference for calibration ( = +0.521 V vs. Ag/AgCl).
Novoncontrol setup (Broadband dielectric spectrometer Concept 40, Germany), integrating an ALPHA frequency response analyzer and a Quatro temperature control system, was used to investigate the dielectric properties over a broad frequency window, 100–106 Hz, in the −140 to +50 °C temperature range. Samples compressed at 10 t/cm2 to form pellets with 13 mm diameter and 0.5 mm thickness were placed between gold plated round electrodes. Dielectric data were collected at constant temperature as a function of frequency at every 5 °C between −140 °C and +50 °C. The bias voltage applied across the sample was 1.0 V.
2.2.1. X-ray crystallography
Crystallographic measurements were carried out with an Oxford-Diffraction XCALIBUR E CCD diffractometer equipped with graphite-monochromated Mo-Kα radiation. The crystal were placed 40 mm from the CCD detector. The unit cell determination and data integration were carried out using the CrysAlis package of Oxford Diffraction.[Citation26] The structure was solved by direct methods using Olex2 [Citation27] software with the SHELXS [Citation28] structure solution program and refined by full-matrix least-squares on Fo2 with SHELXL-97.[Citation28] All atomic displacements for non-hydrogen were refined using an anisotropic model. Hydrogen atoms were placed in fixed, idealized positions, and refined as rigidly bonded to the corresponding atom. The Flack parameter has been refined to 0.01(1) in order to evidence the presence of an enantiopure compound. The molecular plots were obtained using the Olex2 program.[Citation27] The main crystallographic data together with refinement details are summarized in Table .
Table 1. Crystallographic data, details of data collection, and structure refinement parameters for DA.
2.3. Procedure
2.3.1. Synthesis of bis(formyl-p-phenoxymethyl)-dimethylsilane, DA
About 3.3338 g of (27.3 mmol) p-hydroxybenzaldehyde was solved in 10 mL dried DMF in a 50 ml round-bottom flask. Two milliliters of (13.6 mmol) bis(chloromethyl)dimethylsilane and 2.1580 g (15.64 mmol) anhydrous K2CO3 were added to the solution. The mixture was heated at 110 °C for 5 h, and subsequently at 130 °C for 7 h after that was cooled at room temperature and filtered to separate KCl formed as a reaction by-product. The filtrate was poured in water when the product precipitated. After separation by decantation and repeatedly washing with water, the product was extracted with chloroform. After the solvent removal from extract, the compound DA was obtained.
IR νmax (KBr), cm−1: 3503w, 3365w, 3074w, 3010w, 2962s, 2938m, 2903m, 2840s, 2739m, 1686vs, 1600vs, 1578vs, 1510vs, 1461s, 1426s, 1394m, 1315s, 1299s, 1261vs, 1215vs, 1181s, 1159vs, 1108s, 1028vs, 833vs, 811vs, 763m, 717w, 643s, 609s, 515s, 482w, 424w;
1H NMR (CDCl3, 400 MHz, ppm): δ 9.87 (s, 2H, H1, CHO), 7.83–7.81 (d, 4H, H2, H2′), 7.07–7.05 (d, 4H, H3, H3′), 3.86 (s, 4H, H4, Si–CH2), 0.33 (s, 6H, H5, Si–CH3);
13C NMR (CDCl3, 400 MHz, ppm): δ 190.74 (C1), 166.14 (C2), 131.87 (C3, C3′), 129.93 (C5), 114.47 (C4, C4′), 58.99 (C6), −1.13 (C7).
2.3.2. Synthesis of polyazomethine, PAZ
About 0.3000 g of (0.9 mmol) bis(formyl-p-fenoxymethyl)-dimethylsilane and 0.2268 g of (0.9 mmol) 2,5-bis(p-aminophenyl)-1,3,4-oxadiazole were dissolved in 30 mL 1:1 (v/v) CHCl3/DMF solvents mixture in a 100 ml round-bottom flask equipped with magnetic stirrer and reflux condenser protected with CaCl2 tube. The mixture was refluxed under stirring for 5 h and monitored by FTIR analysis, after which the solvent was freely evaporated. A yellow crystalline powder remained that was analyzed as such.
IR νmax (KBr), cm−1: 3437w, 2959m, 2934m, 2887w, 2856w, 2837w, 1624s, 1609s, 1595vs, 1574vs, 1510vs, 1483s, 1441m, 1418s, 1385s, 1367m, 1308s, 1256vs, 1196m, 1167s, 1097s, 1076s, 1030s, 962m, 883w, 860s, 835s, 818s, 802s, 748m, 721m, 708m, 683m, 638w, 631w, 606w, 565w, 546s, 515m, 465w, 388w, 372w.
Far IR νmax (KBr), cm−1: 685w, 631vw, 606vw, 565m, 546s, 515m, 388m, 339m, 311m, 193vs.
1H NMR (DMSO-d6, 400.13 MHz, δ, ppm): δ 8.60 (–CH=N–), 8.08–6.70 (aromatic protons from diamine and dialdehyde), 3.86(–Si–CH2–), 0.22–0.06 (–Si–CH3).
2.3.3. Synthesis of polyazomethine–metal complexes, PAZ-M
About 0.5000 g of (1.5 mmol) bis(formyl-p-fenoxymethyl)-dimethylsilane and 0.3850 g of (1.5 mmol) 2,5-bis(p-aminophenyl)-1,3,4-oxadiazole were dissolved in 50 mL 1:1 (v/v) CHCl3/DMF solvents mixture in a 100 ml round-bottom flask equipped with magnetic stirrer and reflux condenser protected with CaCl2 tube. The mixture refluxed under stirring for 5 h, after which the mixture was cooled at room temperature and stepwise added to a solution consisting of 1 g (6 mmol) CuCl2∙2H2O dissolved in 10 mL 1:1 (v:v) CH3OH/CHCl3 solvents mixture and heated at 60 °C. In the final, a drop of triethylamine was added and the system was kept at the same temperature for 1 h. The color was changed to dark green. The same color had the crystalline powder remained after the freely solvent evaporation at room temperature. This was repeatedly extracted with water to remove the excess of the metal salt and finally with petroleum ether and dried.
The same procedure and equivalent amounts of reactants were used to prepare the complex of the polyazomethine with Co(II) and Zn(II).
2.3.3.1. PAZ-Cu
IR νmax (KBr), cm−1: 3451m, 3352m, 3065w, 2963w, 1649s, 1599vs, 1493s, 1474s, 1433s, 1412s, 1385s, 1315m, 1286m, 1259s, 1173s, 1069s, 1015s, 893vw, 837s, 802s, 743m, 702m, 650w, 636vw, 557m, 538m, 442vw, 397w.
Far IR νmax (KBr), cm−1: 667vw, 644w, 636w, 608s, 548m, 538m, 532m, 513s, 488m, 457vw, 426m, 397vw, 370w, 326m, 299s, 280s, 266m, 257m, 245s, 224s, 212s, 195vs.
2.3.3.2. PAZ-Co
IR νmax (KBr), cm−1: 3410vs, 3400vs, 2961w, 1649s, 1599vs, 1510m, 1493m, 1437m, 1416m, 1373m, 1342w, 1315m, 1285m, 1254m, 1173s, 1105w, 1097w, 1072m, 1026m, 1013m, 964w, 837s, 818m, 744m, 721m, 700m, 652w, 638w, 561w, 546m, 530m, 424w.
Far IR νmax (KBr), cm−1:687s, 667w, 640w, 606w, 563m, 546vs, 517m, 465vw, 417w, 384s, 336w, 293w, 264w, 243m.
2.3.3.3. PAZ-Zn
IR νmax (KBr), cm−1: 3559s, 2961m, 2936w, 2839w, 2012vw, 1935vw, 1655vs, 1607vs, 1597vs, 1574s, 1510s, 1495s, 1468m, 1435m, 1418m, 1385m, 1373m, 1308m, 1256vs, 1196w, 1167s, 1097s, 1076s, 1028s, 964m, 883vw, 860s, 837s, 818s, 804s, 748m, 721w, 708m, 687w, 667w, 642w, 608w, 598w, 546m, 515m, 388w.
Far IR νmax (KBr), cm−1: 669m, 648s, 604s, 540vs, 515vs, 424m, 384s, 355m, 332s, 301m, 282s, 243s.
3. Results and discussion
A Williamson-type reaction was used to prepare a silicon-containing dialdehyde (DA) by treating bis(chloromethyl)dimethylsilane with p-hydroxybenzaldehyde in 1:2 M ratio, in DMF, in the presence of K2CO3 (Scheme ).
The X-ray single crystal study has confirmed the anticipated chemical composition and structure for silicon-containing dialdehyde (DA). Its molecular structure is depicted in Figure (a). The crystal structure is built up from the packing of dimolecular entities (Figure (b)), the formation of which occurs via π–π stacking interaction. The centroid-to-centroid distance between the interacting aromatic systems constitutes 3.687 Å.
Figure 1. (a) X-ray molecular structure of silicon-containing dialdehyde (DA); (b) π–π stacking interaction in the crystal structure DA. Hydrogen atoms are omitted.
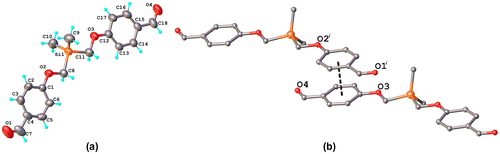
Figure 4. Absorption spectra in DMSO solution of polyazomethine derivatives: PAZ; PAZ-Cu; PAZ-Co; and PAZ-Zn.
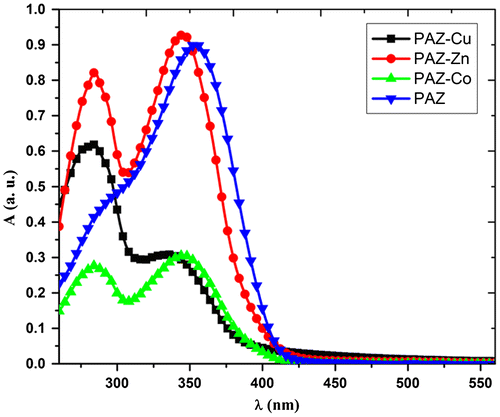
Figure 6. Fluorescence spectra of polyazomethine PAZ in DMSO solution at different excitation wavelengths: 320; 330; 340; 350; 360 nm.
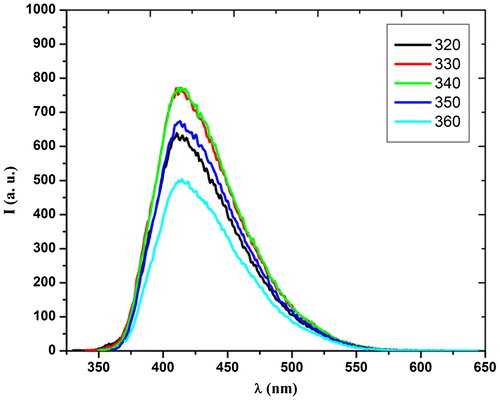
Figure 7. PAZ: (a) cyclic voltammogram recorded during the potential electrode scanning from 0.0 to 1.4 V range with 50 mV/s; (b) cyclic voltammogram recorded during the electrode potential scanning from 0.0 to −1.4 V range with 50 mV/s; (c) the multi-cyclic voltammograms recorded for PAZ on the positive range of potential; and (d) cyclic voltammograms of PAZ recorded at different scan rates (from 20 to 250 mV/s).
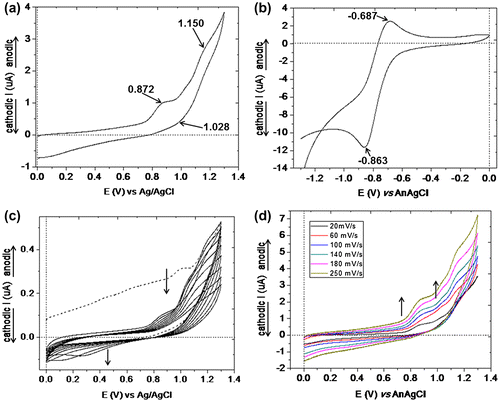
Figure 8. PAZ-Cu: (a) cyclic voltammogram recorded during the potential electrode scanning from 0.0 to 1.3 V range with 50 mV/s; (b) cyclic voltammogram recorded during the electrode potential scanning from 0.0 to −1.3 V range with 50 mV/s; (c) the multi-cyclic voltammograms (10 cycles) recorded for PAZ-Cu on the positive range of potential; and (d) cyclic voltammograms of PAZ-Cu recorded at different scan rates (from 20 to 250 mV/s).
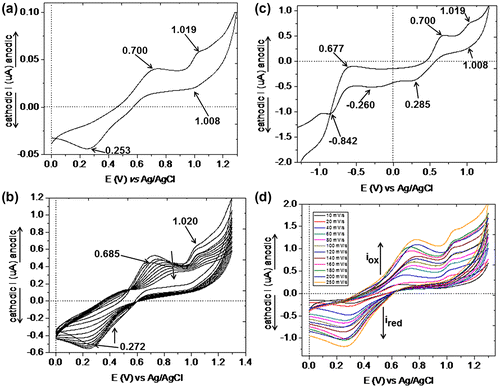
Figure 9. Frequency dependence of the ac conductivity σac, dielectric permittivity ε’, and dielectric loss ε” at room temperature for PAZ, PAZ-Cu, PAZ-Co, and PAZ-Zn.
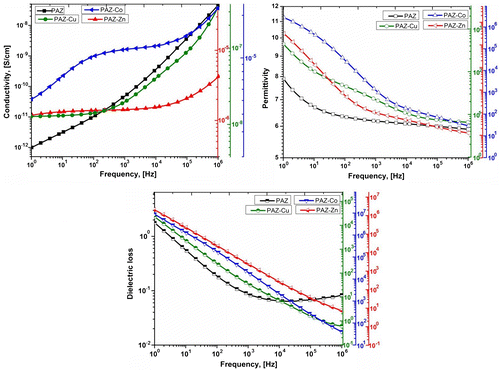
The formation of the presumed structure was first confirmed by the presence in FTIR spectrum (Figure 4S) of the absorption band at 1028 cm−1 characteristic for the new formed –CH2–O–Ar– ether linkage. There are also present other bands that support the structure: 1686 cm−1 (–HC=O), 1261 and 811 cm−1 (Si–CH3), 1600 and 833 cm−1 (aromatic ring). In 1H NMR spectrum (Figure 5S), the peaks for all specific protons can be found in the expected ratio of the intensities: –CH3:–CH2–:aromatic–H:–CHO: 3:2:4:1. In 13C NMR spectrum (Figure 6S), there are also peaks present corresponding to all carbon atoms according to the presumed structure: 190.74 (–CH=O), 166.14, 131.87, 129.93, and 114.47 (aromatic C), 58.99 (–O–CH2–Si), and −1.13 (CH3–Si).
3.1. Synthesis of polyazomethine, PAZ
The above-prepared dialdehyde, DA, was used as co-monomer in the polycondensation reaction with 2,5-bis(p-aminophenyl)-1,3,4-oxadiazole in 1:1 M ratio in CHCl3/DMF solvents mixture to prepare polyazomethine, PAZ (Scheme ).
The disappearance of the carbonyl absorption band at 1686 cm−1 and the presence of a new band at 1624 cm−1 confirm the polycondensation occurrence with the formation of polyazomethine (Figure 7S). The other main bands characteristic for the presumed structure are: 1609 cm−1 (C=N group from imidazole ring), 1595, and 835 cm−1 (aromatic C=C), 1076 cm−1 (C–O oxadiazole ring), 1030 cm−1 (–CH2–O–Ar–), 1256 and 818 cm−1 (Si–CH3). The absorption bands at 962 and 1097 cm−1 are assigned to the oxadiazole ring in plane bending. The weak absorption bands at 3065 and 3042 cm−1 are assigned to C–H of the phenylene rings, while those at 2959 and 2934 cm−1 are due to C–H bond from Si–CH3 group.
1H NMR spectrum of PAZ (Figure 8S) reveals the presence of the following peaks: 8.6 ppm (–CH=N–), 6.70–8.08 ppm (protons from aromatic rings derived from the two co-monomers), 3.86 ppm (–CH2–O–), 0.06–0.22 ppm (Si–CH3). Their presence and intensities ratio, –CH3: –CH2–:H aromatic: –CH=N–: 3:2:8:1, confirm the presumed structure.
The reaction product is soluble in polar aprotic solvents like DMF or DMSO. The good solubility is due to the flexible etheric and Si–C bonds but also due to the presence of dimethylsilane units that limits the formation of the intermolecular hydrogen bonds. The heterogeneity of the chain conferred by the co-existence of the aromatic and oxadiazole rings also contributes to improving solubility.
GPC measurements in DMF revealed an unimodal curve and permitted to estimate the molecular mass values that were: Mn = 7085, Mw = 8420 and polydispersity index, PDI = 1.2.
3.2. Complexation
The nitrogen atoms from azomethine and oxadiazole units in the main chain of polyazomethines are capable of protonation and complexation.[Citation5] In order to obtain some metal complexes of above described polyazomethine, a one-step procedure was applied by mixing together the two precursors of polyazomethine PAZ (dialdehyde and diamine) with metal salt in excess (Scheme ).
Scheme 3. A general reaction pathway leading to metal complexes of the polyazomethine PAZ; possible coordination sites are indicated.
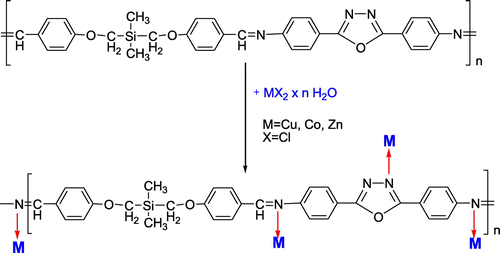
The occurrence of the complexation process was firstly proved by modifications appeared in FTIR spectra (Figures 9S–11S). The band from 1624 cm−1 assigned to the CH=N group in polymer shifted with 25–30 cm−1 to higher wave numbers as compared to the polyazomethine ligand (Figure 12Sa). This indicates coordination of the azomethine nitrogen to the metal ions as other references revealed.[Citation29–Citation31] There are many views on the position of vibration frequency of azomethine group, this being influenced by the physical state of the compound, the presence and position of substituents, hydrogen bonding and conjugation with either the C=N-carbon or the C=N-nitrogen, or both.[Citation32] The CH=N frequency of PAZ-Cu appears as a strong band at 1649 cm−1, shifted with 25 cm−1 from free polyazomethine, while those of PAZ-Co and PAZ-Zn are shifted with 30 cm−1 to higher frequency, suggesting that the azomethine group may coordinate to a metal ion by σ-donation, which implies a weakening effect of the π-back bonding. This will allow azomethine bond to pull electron density toward it, strengthening the metal C=N bond, which increases the frequency of the CH=N stretching vibration. Also, the imine group may coordinate by π-donation from the metal, which reduces the CH=N frequency. The CH=N stretching frequency also depends on the oxidation state, coordination number, and the electronegativity of the metal atom, as well as by the other ligands attached to the atom.[Citation32,Citation33]
The other characteristic bands for C=N group from oxadiazole ring shifted to lower wave number. Thus, in PAZ-Cu the absorption band at 1609 cm−1 in polyazomethine is shifted to 1599 cm−1, while in PAZ-Co is shifted at 1597 cm−1 and at 1601 cm−1 in PAZ-Zn. This suggests the participation of N from oxadiazole ring in coordination to the metal center. The absorption bands characteristic for the oxadiazole ring at 962 and 1097 cm−1 in the polyazomethine spectrum are not visible in the spectrum of the PAZ-Cu complex, while in the spectra of PAZ-Co and PAZ-Zn the band at 962 cm−1 is shifted to 964 cm−1, and that at 1097 cm−1 appears as a shoulder in spectrum of PAZ-Co and it is shifted at 1086 cm−1 in PAZ-Zn spectrum (Figure 12Sb). Other characteristic bands for polymeric complexes are 1583 and 839 cm−1 in PAZ-Cu, 1576 and 838 cm−1 in PAZ-Co, 1578 and 845 cm−1 in PAZ-Zn (aromatic C=C); 1069 cm−1 in PAZ-Cu, 1073 cm−1 in PAZ-Co, 1079 cm−1 in PAZ-Zn (C–O oxadiazole ring); 1029 cm−1 (–CH2–O–Ar–), 1260 and 803 cm−1 in PAZ-Cu, 1253 and 818 cm−1 in PAZ-Co, 1259 and 803 cm−1 in PAZ-Zn (Si–CH3) (Figure 12Sc). IR data revealed broad bands at 3451 cm−1 in PAZ-Cu, 3400 cm−1 in PAZ-Co and 3559 cm−1 in PAZ-Zn due to the coordinated water in the polymeric complexes.[Citation34]
The far infrared region includes vibrations arising from coordination of the metal to the ligand. In far-IR (Figures 13S–15S), it can be seen the appearance of the absorption bands characteristic for the new created metal–ligand bonds at 370 and 397 cm−1 (Cu–N), 384 and 417 cm−1 (Co–N) and 384 and 424 cm−1 (Zn–N) [Citation31,Citation34–Citation36] (Figure 12Sd). These observations support the hypothesis that metal ions are complexed through both nitrogen atom types from azomethine group and oxadiazole ring. The use of metal(II) chloride salts introduces the possibility of halide coordination. This aspect is supported by the appearance of new bands at 326, 245, and 224 cm−1 in the far infrared spectrum of PAZ-Cu, 336 and 243 cm−1 (PAZ-Co), 332 cm−1 (PAZ-Zn) that are assigned to M (Cu, Co, Zn)-Cl.[Citation32]
Analysis by XRF reveals different Si:M molar ratios in the three complexes: Si:Cu-1:2.65, Si:Co-1:2, and Si:Zn-2:1. These differences would be assigned to the different affinities of the three metals for the ligating groups and their coordination pattern.
The metal complexes are soluble in common solvents: methanol, THF, DMF, DMSO (and their mixture with chloroform), while the polyazomethine only in DMF and DMSO. In general, the metal complexation by polymeric ligands can be either inter- or intramolecular, depending on the nature and position of the ligand groups, backbone nature and flexibility, and the polarity of the reaction medium. Inter-chain coordination gives cross-linked, insoluble structures (usually, the case of complexation of hydroxy-functionalized polyazomethines).[Citation37,Citation38] Intramolecular complexation of metal ions by a polymer having donor atoms within the chain, which we assume that is our case (when nitrogen atoms from imine and oxadiazole units are alternated along the backbone), results in general in linear polymer–metal complexes with improved solubility. To this also contributes the fact that the involvement of the polar groups in complexation reduces their ability to participate in the formation of the physical intermolecular bonds, as was demonstrated in the literature.[Citation39]
3.3. Evaluation of the properties
3.3.1. Thermal behavior
Figures and 16S show the TGA and DTG curves of the obtained compounds. Some characteristics extracted from these curves are presented in Table along with the main DSC data.
Table 2. The main parameters evaluated from TGA, DTG, and DSC curves.
The results clearly reveal that the polymers exhibit similar degradation patterns which consist of three distinctive steps, excepting the Zn-complexed polymer, which exhibits four steps of thermal decomposition. It can be observed from Figure and Table that the simple polyazomethine possesses higher thermal stability as compared with the derived metal complexes. An explanation for the decrease in thermostability as a result of the complexation would be based on: the bulkiness of the used metals; coordination modes and the number of established coordinative bonds; and the nature of solvents used during the synthesis and separation procedures. These factors lead to the disruption of the symmetry of polyazomethinic chain, thus hindering their close packaging and disrupts the interchain hydrogen bonding or π–π interactions which leads to lower thermal stability.[Citation40]
On the other hand, other studies on the thermostability of a number of Schiff bases and derived metal complexes lead also to the conclusion that the thermostability of the complexes is lower as compared with simple polyazomethine ligand. The metal ions in the polychelates were stated to be responsible in catalyzing the thermal decomposition resulting in the molecular framework to undergo fragmentation.[Citation41–Citation43]
Based on the similar structures studied in literature,[Citation44] one may assume that, due to the high temperature, the azomethine linkage (–HC=N–) is the first breaking unit, in all cases. This process is attributed to the second thermal decomposition stages of PAZ, PAZ-Cu, and PAZ-Co samples and to the third stage in the case of PAZ-Zn sample. Similar weight loss values in the range 14.67–26.50% can be observed for the above-mentioned thermal decomposition stages. Two peaks on DTG curve appeared on the first stages of thermal decomposition for the PAZ-Cu (77.58 and 135.00 °C) and PAZ-Co (85.00 and 117.00 °C) samples corresponding to two overlapping thermal decomposition processes. The two processes are distinguished on the thermogram of the PAZ-Zn sample as individual mass loss stages. The two processes may be attributed to both solvent loss, DMF, which evolves in steps up to 200 °C and even higher, and coordinated water molecules. This explains the high similar weight loss values in the range 12.16–18.87% for the first stages of thermal decomposition of the Cu- and Co-complexed polymers, and the summed up mass loss values for first and second stage of thermal decomposition of the Zn-complexed polymer. The first stage of thermal decomposition of PAZ may correspond to residual solvent loss. Finally, the degradation of the polymer backbone mainly comprised of oxadiazole and para-substituted phenylene rings occurred in the Tonset ranges 442.74–499.41 °C,[Citation40] corresponding to each last stage of thermal decomposition. Similar weight loss values in the range 17.64–28.28% were exhibited for these stages. The high quantities of remained residues after complete degradation (39.90–50.58%) probably consist mainly of the highly thermostable SiO2 moieties and metal oxides.[Citation45] The high residue at high temperature is an advantage in a future use of such compounds in electronic devices. However, the residues in the case of the complexes are lower than would be expected given the presence of metal. This could be explained by partial evolving of the silicon derivatives formed as volatile fraction under the catalytic action of the metal.
The first DSC heating, cooling, and second heating traces of the simple polyazomethine PAZ, as well as the second heating curves of the complexed polymers are comparatively presented in Figure .
The glass transition temperature (Tg) value of the polyazomethine is around 48 °C. This aspect is in contrast with the known fact that polyazomethines are high Tg materials. This large depression of the Tg value resulted from the high heterogeneity of the chain, as well as from the flexibility of the ether and carbosilane groups. These moieties act as plasticizers for the polymer backbone. Similar observations were reported in the literature.[Citation46–Citation49] For example, a glass transition domain centered at 39 °C was observed for polyazomethine derived from 2,5-bis(n-dodecyloxy)terephthaldehyde and para-phenylene diamine.[Citation46] The Cu and Co polymeric complexes exhibited similar Tg values of 65.00 and 67.51 °C, higher than the Tg value of the starting polymer. This is due to the bulkiness of the metals which leads to weaker segmental motion of the chains owed to chain stiffness because of a reduction of free volume in the structure. Furthermore, the coordinative linkages may behave similar to chemical cross-linking phenomenon, generating network structures which lead to an increase in the rigidity of the complexed polymers with the disruption of hydrogen bonding capacity, thus lowering the thermal stability of the structures, as previously shown. The reduction of free volume between segmental chains is confirmed by the disappearance of the complex melting/crystallization profile (Tm/Tcr) of the complexed polymers (Figure and Table ). Only the Zn-complexed polymer exhibited a lower Tg value (41 °C) than that of the initial polymer, probably due to different coordination pattern. In addition, as XRF results reveal, in PAZ-Zn there is the lowest ratio Zn:Si. The DSC data was thus shown to be in good agreement with the TGA data.
3.3.2. Optical properties
The optical properties of PAZ and its metal complexes were investigated by electronic absorption and fluorescence spectroscopy by using their solutions in DMSO. The electronic absorption spectrum of PAZ in DMSO solution showed a broad and intense absorption band at about 354 nm and a shoulder at 297 nm (Figure ). The absorption band at higher wavelengths can be assigned to a π–π* transition involving the azomethine-phenol oxadiazole framework.[Citation11]
2,5-bis(p-aminophenyl)-1,3,4-oxadiazole exhibits two absorption bands in DMSO at around 342 and 286 nm, respectively. The UV absorption around 342 nm is more intense compared to that at 286 nm. The additional band at about 285 nm corresponds to the transition in the benzene rings. The bathochromic shift of the PAZ absorption band relating to the position of oxadiazole derivative band can be determined by the extension of π-electron delocalization length through π-conjugated polyazomethine main chain. The UV–vis absorption spectra of polyazomethine complexes with metal ions (Cu2+, Co2+, and Zn2+) are also illustrated in Figure . As a result of the complexation, the absorption band at about 354 nm in PAZ displays a blue shift in polyazomethine complexes.[Citation50] The greatest hypsochromic shift appears at 338 nm for Cu2+ complex.
The optical energy band gaps, , in solution were evaluated from the absorption spectra (Table ). The polyazomethine PAZ exhibited a lower value for energy gap, while higher values of energy gap were found for the azomethine complexes. Complex formation determines the increase of the Eg values due to the different planarity of azomethine structure in resulting complexes which can influence the conjugation length and the conformation of the polymer backbone.
Table 3. Spectral data of azomethine derivatives
Fluorescence emission spectrum of PAZ reveals an intense and broad band at about 410 nm under an excitation wavelength of 340 nm (Figure ). It was found that the maximum of emission band of azomethine complexes with Co(II) and Zn(II) under excitation of 340 nm was hypsochromically shifted in comparison with emission maximum of PAZ, while the emission band of copper complex presented a bathochromic shift (Figure ).
At the same time, the Stokes shift has the highest value for PAZ-Cu complex (Table ), but this is not significantly high, suggesting that the complexation exerts no significant influence on the electronic states in the visible range. The fluorescence of these polyazomethines is mainly due to the presence of 1,3,4-oxadiazole ring which is known to be a fluorescent unit.[Citation7] In fact, the emission band of 2,5-bis(p-aminobiphenyl)-1,3,4-oxadiazole is located at 404.5 nm under 340 nm excitation wavelength. For PAZ and its metal complexes no changes in the maximum of the emission bands or in the shape of emission spectra were observed at different excitation wavelengths indicating that there is one emitting species in system (Figure ).
The complexation process by coordination of metal ions with nitrogen atoms (Scheme ) causes an enhancement of fluorescence in the following order: PAZ-Zn > PAZ-Co > PAZ-Cu > PAZ. The emission intensities of PAZ-Zn and PAZ-Co have the highest values and enough near. The formation of metal–polymer complexes can reduce the nonradiative decay of the excited states leading to the fluorescence enhancement by the increase of the rigidity of conjugated polymer chain or increase of the planarity of conjugated polymer chain.[Citation51]
3.3.3. Cyclic voltammetry
The electrochemical behavior of the polyazomethine-containing oxadiazole groups and its metal complexes was investigated by recording the cyclic voltammograms in DMF solution containing tetrabutylammonium tetrafluoroborate (0.1 M) as supporting electrolyte. All the measurements were performed at room temperature (about 25 °C), under nitrogen atmosphere. The cyclic voltammograms were recorded on both negative and positive potential range, both at constant (50 mV/s) and increasing scan rate between 10 and 250 mV/s. Multi-cyclic voltammograms were also recorded for all samples in the positive range of potential.
In Figure are presented the cyclic voltammograms of PAZ. CVs diagrams recorded on the positive range of the potentials indicate two irreversible anodic peaks at Epa1 = 0.872 V and Epa2 = 1.150 V, with an onset of = 0.762 V, which can be assigned to the oxidation process of imine group from the polymer backbone. On the reverse scan, only one large cathodic peak can be observed at Epc = 1.028 V, which corresponds to reduction process of the oxidized species at the electrode–solution interface. On the negative potential region, the polyazomethine PAZ exhibits two semi-reversible peaks, located at Epa = −0.687 V (with an onset of
= −0.886 V) and Epc = −0.863 V (with an onset of
= −0.646 V) (Figure (b)). These peaks can be related to the oxidation and reduction processes of the oxadiazole groups. Oxadiazole groups act as electron-acceptor units which can form stable radical anions.
The current intensities of the anodic peaks are diminished as the number of the scans is increased (Figure (c)), and this is due to the instability of the oxidized species formed during the first scan or to the formation of non-electrochemically active species. In addition, by increasing scan rate from 20 to 250 mV/s, an increase of anodic current intensities can be observed, and the redox peaks become more pronounced (Figure (d)). An explanation for this can be related to the fast electron transfer reactions that occur between the polymer chains and the electrode.
On the positive range of the potential, polyazomethine PAZ-Cu exhibits two anodic peaks at Epa1 = 0.700 and Epa2 = 1.019 V which are due to the oxidation processes of the imine groups (Figure ). The cathodic peak at Epc1 = 1.008 V is attributed to the reduction process of the oxidized species. The complexation of imine segments and oxadiazole groups with Cu ions is reflected in the appearance of two new cathodic peaks at Epc2 = 0.285 and Epc3 = −0.260 V, respectively. These peaks can be attributed to the reduction process of the Cu(II) to Cu(I). By increasing the scanning rate of the applied working electrode potential, the current intensities of the anodic peaks are increased.
In the case of PAZ-Co, the peaks associated with the oxidation process of the polyazomethine backbone, shifted to more positive values as a result of the metal complexation and this can be correlated with the complexation processes of the imine groups and Co ions (Figure 17S). The polyazomethine PAZ-Co exhibits two anodic peaks at Epa1 = 1.010 and Epa2 = 1.399 V, and on the reverse scanning, only one cathodic peak appear at Epc = 1.167 V. By scanning on the negative potential range, it was observed that the peak current intensities of the redox couple assigned to oxadiazole groups are diminished. This can be attributed to the involvement of oxadiazole groups in the coordination processes with Co ions. A new cathodic peak can be observed in the CV of the PAZ-Co at Epc = −0.185 V which can be attributed to the reduction process of the Co ions, from Co(III) to Co(II).
The cyclic voltammograms of the PAZ-Zn complex revealed only one large anodic peak and one cathodic peak corresponding to the oxidation and reduction of the polyazomethine backbone, as for the previous cases (Figure 18S). These redox peaks are not stable, and after 10 cycles, their current intensities decreased, probably due to the formation of non-electroactive species (Figure 18Sc). In addition, the current intensity of the peaks increases gradually with the scan rate (Figure 18Sd). A new cathodic peak appears on the reverse scanning, at Epc2 = −0.200 V, which can be correlated with the reduction process of the Zn ions, from Zn(II) to Zn(I). The corresponding redox couple peaks attributed to oxadiazole groups appear at Epa = −0.784 and Epc = −0.927 V.
It can be seen that, the metal presence induces changes in the redox behavior of the polyazomethine, these being in dependence on the metal nature.
3.3.4. Dielectric measurements
It would be expected as the co-existence of the aromatic and oxadiazole moieties linked by azomethine groups to give rise to some interesting properties, especially electrophysical ones. More, by the metal attaching through complexation, this effect may be enhanced. The metal ion presence in the polymer may favor the appearance of electroconductivity, although almost all of the metal-containing polymers are insulators [Citation52] or operate in the range of semiconductors (σ = 10−8–1 S cm−1).[Citation53] This is due to the problems associated with matching the orbital energies of the conjugated organic components and metal centers.[Citation53] However, there are still enough reasons for taking into account their electrical properties. One of them is the ability of the metal to reversibly bind additional ligands resulting in a very sensitive modification of the conductivity of the system due to alteration of the energy levels of the metal-based orbitals. This would be the basis for a sensing mechanism to detect gaseous molecules. Small molecules, often analytes, for example a gas, CO2, CO, NO, etc. can be additional ligands.[Citation53]
The dielectric responses in dependence on frequency were registered at room temperature by using dielectric spectroscopy for the simple polyazomethine and derived metal complexes. Variation of these parameters with frequency at room temperature is presented in Figure . As can be seen, at low frequencies, the permittivity of the polyazomethine–metal complexes is higher (103-PAZ-Cu to 105–106-PAZ-Zn and PAZ-Co) as compared with that for simple polyazometine (around 8.00) but a significant decreasing occurs by rising frequency. Very closely values are attained for all samples at high frequency (between 5.9 (PAZ) – 28.7 (PAZ-Co) at 106 Hz). The dielectric loss also decreases as frequency increases. This behavior at lower frequencies is due to the mobility of charge carriers. AC conductivity derived from these data as σac = ε0 ω ε″ (where ε0 is the permittivity of free space, ε″ is the dielectric loss and ω is the angular frequency) [Citation54,Citation55] is also plotted. As it can be seen, while the dielectric parameters, permittivity and loss, decrease, the conductivity rises by frequency increasing. Almost linear conductivity-frequency dependence in the case of PAZ and PAZ-Cu is an indication for hopping type conduction.[Citation56] However, conductivity values so determined are situated at the bottom of the semiconductor field. The conductivity values ranges between 10−12 and 10−5 S/cm. The existence of the unconjugated –O–CH2–Si–CH2–O– sequence within the backbone, could be a reason for this behavior. The temperature dependence of the dielectric parameters registered in the range −100–50 °C for PAZ-Co and PAZ-Zn reveals in both cases, an increasing of the permittivity, dielectric loss, and conductivity as temperature rising with transitions around −60 and −30 °C regardless of the metal type (Figures 19S and 20S).
For temperatures bellow these values, the conductivity depends approximately linear on frequency, in logarithmic scale. On increasing temperature, conductivity is almost constant for the lower frequencies and, for the upper temperatures the conductivity is approximately equal for the entire frequency range. At lower temperatures, the movement of charge carriers is limited on short distances but at temperatures above the mentioned interval, a continuous network that permits electric conduction through the entire media is created.
4. Conclusions
A new polyazomethine containing many types of organic groups either functional or not (phenylene, ether, carbosilane, azomethine, oxadiazole) has been prepared based on 2,5-bis(p-aminophenyl)-1,3,4-oxadiazole and a new dialdehyde, bis(formyl-p-phenoxymethyl)-dimethylsilane. Each of these groups induces certain behaviors. Thus, the presence of ether linkages and Si–C bonds confer flexibility to the polymer chain reflected in low glass transition (Tg in the range 41–67.5 °C) and good solubility; oxadiazole ring confers fluorescence, but also redox activity and ability to form metal complexes as azomethine group also does. The metal presence leads to the increase in the dielectric constant and conductivity values. The most effective in this direction proved to be Co. Instead, the metal presence results in lowering of the thermostability of the polyazomethine.
Supporting information
CCDC 928195 contains the supplementary crystallographic data for this contribution. These data can be obtained free of charge via www.ccdc.cam.ac.uk/conts/retrieving.html (or from the Cambridge Crystallographic Data Centre, 12 Union Road, Cambridge CB2 1EZ, UK; fax: (+44) 1223-336-033; or [email protected]).
FTIR, 1H NMR, and 13C RMN spectra for 2,5-bis(p-aminophenyl)-1,3,4-oxadiazole and dialdehyde DA, FTIR, and 1H NMR spectra for polyazomethine PAZ, FTIR (Mid – and Far-IR) spectra for PAZ-Cu, PAZ-Co, and PAZ-Zn, comparative IR spectra details for polyazomethines and metal complexes, DTG curves of the polyazomethine-based structures, cyclic voltammograms of PAZ-Co and PAZ-Zn, temperature dependence of the ac conductivity σac, dielectric permittivity ε′ and dielectric loss ε″ of PAZ-Co and PAZ-Zn at different frequencies (in Hz).
Supplemental data
Supplemental data for this article can be accessed http://dx.doi.org/[10.1080/15685551.2014.907623].
TDMP_A_907623_suppl.pdf
Download PDF (1.4 MB)Acknowledgments
This research was financially supported by European Regional Development Fund, Sectoral Operational Programme ‘Increase of Economic Competitiveness’, Priority Axis 2 (SOP IEC-A2-O2.1.2-2009-2, ID 570, COD SMIS-CSNR: 12473, Contract 129/2010-POLISILMET).
References
- Burroughes JH, Bradley DDC, Brown AR, Marks RN, Mackay K, Friend RH, Burns PL, Holmes AB. Light-emitting diodes based on conjugated polymers. Nature. 1990;347:539–541.
- Grigoras M, Antonoaia NC. Synthesis and characterization of some carbazole-based imine polymers. Eur. Polym. J. 2005;41:1079–1089
- Sek D, Grucela-Zajac M, Krompiec M, Janeczek H, Schab-Balcerzak E. New glass forming triarylamine based azomethines as a hole transport materials: thermal, optical and electrochemical properties. Opt. Mater. 2012;34:1333–1346.
- Tunçel M, Özbülbül A, Serın S. Synthesis and characterization of thermally stable Schiff base polymers and their copper(II), cobalt(II) and nickel(II) complexes. React. Funct. Polym. 2008;68:292–306.
- Hussein MA, Abdel-Rahman MA, Geies AA. New heteroaromatic polyazomethines containing naphthyridine moieties: synthesis, characterization, and biological screening. J. Appl. Polym. Sci. 2012;126:2–12.
- Sek D, Jarzabek B, Grabiec E, Kaczmarczyk B, Janeczek H, Sikora A, Hreniak A, Palewicz M, Lapkowski M, Karon K, Iwan A. A study of thermal, optical and electrical properties of new branched triphenylamine-based polyazomethines. Synth. Met. 2010;160:2065–2076.
- Marin L, Damaceanu MD, Timpu D. New thermotropic liquid crystalline polyazomethines containing luminescent mesogens. Soft Mater. 2009;7:1–20.
- Sek D, Iwan A, Jarzabek B, Kaczmarczyk B, Kasperczyk J, Janeczek H, Mazurak Z. Characterization and optical properties of oligoazomethines with triphenylamine moieties exhibiting blue, blue-green and green light. Spectrochim. Acta, Part A. 2009;72:1–10.
- Saegusa Y, Koshikawa T, Nakamura S. Synthesis and characterization of 1,3,4-oxadiazole-containing polyazomethines. J. Polym. Sci., Part A: Polym. Chem. 1992;30:1369–1373.
- Cooper LL, Samulski ET, Scharrer E. Towards room temperature biaxial nematics. Mol. Cryst. Liq. Cryst. 2009;511:203–217.
- Marin L, Perju E, Damaceanu MD. Designing thermotropic liquid crystalline polyazomethines based on fluorene and/or oxadiazole chromophores. Eur. Polym. J. 2011;47:1284–1299.
- Wang CS, Jung GY, Hua YL, Pearson C, Bryce MR, Petty MC, Batsanov AS, Goeta AE, Howard JAK. An efficient pyridine- and oxadiazole-containing hole-blocking material for organic light-emitting diodes: synthesis, crystal structure, and device performance. Chem. Mater. 2001;13:1167–1173.
- Schulz B, Bruma M, Brehmer L. Aromatic poly(1,3,4-Oxadiazole)s as advanced materials. Adv. Mater. 1997;9:601–613.
- Janietz S, Barche J, Wedel A, Sainova D. n-Type copolymers with fluorene and 1,3,4-heterodiazole moieties. Macromol. Chem. Phys. 2004;205:187–198.
- Saegusa Y, Sekiba K, Nakamura S. Synthesis and characterization of novel 1,3,4-oxadiazole- or 1,3,4-thiadiazole-containing wholly conjugated polyazomethines. J. Polym. Sci., Part A: Polym. Chem. 1990;28:3647–3659.
- Cazacu M, Marcu M, Vlad A, Toth A, Racles C. Chelate polymers. III. New polyazomethines of 5,5′-methylene-bissalicylaldehyde with siloxane diamines and their divalent metal complexes. J. Polym. Sci., Part A: Polym. Chem. 2003;41:3169–3179.
- Cazacu M, Vlad A, Munteanu G, Airinei A. Multifunctional materials based on polyazomethines derived from 2,5-dihydroxy-1,4-benzoquinone and siloxane diamines. J. Polym. Sci., Part A: Polym. Chem. 2008;46:1862–1872.
- Vlad A, Cazacu M, Munteanu G, Airinei A, Budrugeac P. Polyazomethines derived from polynuclear dihydroxyquinones and siloxane diamines. Eur. Polym. J. 2008;44:2668–2677.
- Racles C, Cozan V, Cazacu M, Földes E, Sajo I. Poly(azomethine-ester-siloxane)s. Synthesis and thermal behavior. High Perform. Polym. 2002;14:397–413.
- Racles C, Silion M, Arvinte A, Iacob M, Cazacu M. Synthesis and characterization of poly(siloxane–azomethine) iron(III) coordination compounds. Des. Monomers Polym. 2013;16:425–435.
- Hamciuc E, Hamciuc C, Bruma M, Schulz B. Poly(1,3,4-oxadiazole-imide)s containing dimethylsilane groups. Eur. Polym. J. 2005;41:2989–2997.
- Bruma M, Schulz B. Silicon-containing aromatic polymers. J. Macromol. Sci., Polym. Rev. 2001;41:1–40.
- Siegrist AE, Maeder E, Duennenberger ME. 2,5-Diaryl-1,3,4-oxadiazoles. Swiss patent 383985. 1965.
- Hamciuc E, Hamciuc C, Cazacu M. Poly(1,3,4-oxadiazole-ether-imide)s and their polydimethylsiloxane-containing copolymers. Eur. Polym. J. 2007;43:4739–4749.
- Franco O, Orgzall I, Reck G, Stockhause S, Schulz B. Structure and high-pressure behavior of 2,5-di-(4-aminophenyl)-1,3,4-oxadiazole. J. Phys. Chem. Solids. 2005;66:994–1003.
- CrysAlis RED, O. D. L. Version 1.171.36.24; 2003.
- Dolomanov OV, Bourhis LJ, Gildea RJ, Howard JAK, Puschmann H. OLEX2: a complete structure solution, refinement and analysis program. J. Appl. Crystallogr. 2009;42:339–341.
- Sheldrick GM. A short history of SHELX. Acta Crystallogr., Sect. A: Found. Crystallogr. 2008;64:112–122.
- Soliman AA, Linert W. Investigations on new transition metal chelates of the 3-methoxy-salicylidene-2-aminothiophenol Schiff base. Thermochim. Acta. 1999;338:67–75.
- Mohamed GG, Omar MM, Hindy AH. Metal complexes of Schiff bases: preparation, characterization, and biological activity. Turk. J. Chem. 2006;30:361–382.
- Philip V, Suni V, Prathapachandra Kurup MR, Nethaji M. Copper(II) complexes derived from di-2-pyridyl ketone N(4),N(4)-(butane-1,4-diyl)thiosemicarbazone: crystal structure and spectral studies. Polyhedron. 2006;25:1931–1938.
- Nakamoto K. Infrared and Raman spectra of inorganic and coordination compounds. New York (NY): Wiley; 1986, 203–236.
- Asali KJ, Janaydeh HA. Reactivity of tungsten(o) and molybdenum(o) pentacarbonyl thiobenzoate anions: thiobenzoate as a cis-CO labilizing ligand. Transition Met. Chem. 2003;28:193–198.
- Wang G, Chang JC. Copper(II) and zinc(II) complexes of Schiff bases derived from amino acids and O-vanillin. Synth. React. Inorg. Met.-Org. Chem. 1994;24:623–630. G.
- Lever ABP, Mantovani E. Isotopic studies of the metal–ligand bond. Part III. The far infrared spectra of some tetragonal diamine complexes of cobalt(II) and nickel(II): studies of the metal–nitrogen bond, as a function of metal ion and of spin state. Can. J. Chem. 1973;51:1567–1581.
- Lever ABP, Mantovani E. Far-infrared and electronic spectra of some bis(ethylenediamine) and related complexes of copper(II) and the relevance of these data to tetragonal distortion and bond strengths. Inorg. Chem. 1971;10:817–826.
- Oriol L, Alonso PJ, Martinez JI, Pinol M, Serrano JL. Structural studies of copper(II)-chelated polymers derived from hydroxy-functionalized liquid crystalline homo- and copolyazomethines. Macromolecules. 1994;27:1869–1874.
- Kaya I, Oksuzgulmez S, Guzel H. Synthesis, characterization, thermal degradation and electrical conductivity of poly-4-[(pyridine-2-yl-imino)methyl]benzene-1,3-diol and polymer-metal complexes. Bull. Chem. Soc. Ethiop. 2008;22:237–246.
- Wang C, Shieh S, LeGoff E, Kanatzidis MG. Synthesis and characterization of a new conjugated aromatic poly(azomethine) derivative based on the 3′,4′-dibutyl-α-terthiophene building block. Macromolecules. 1996;29:3147–3156.
- Al-Ghamdi RF, Fahmi MM, Mohamed NA. Thermal stability and degradation behavior of novel wholly aromatic azopolyamide-hydrazides. Polym. Degrad. Stab. 2006;91:1530–1544.
- Samal S, Das RR, Dey RK, Acharya S. Chelating resins VI: chelating resins of formaldehyde condensed phenolic Schiff bases derived from 4,4′-diaminodiphenyl ether with hydroxybenzaldehydes’ synthesis, characterization, and metal ion adsorption studies. J. Appl. Polym. Sci. 2000;77:967–981.
- Samal S, Das RR, Sahoo D, Acharya S, Panda RL, Rout RC. Chelating resins. III. Synthesis, characterization, and capacity studies of formaldehyde-condensed phenolic Schiff bases derived from 1,2-diamines and hydroxy benzaldehydes. J. Appl. Polym. Sci. 1996;62:1437–1444.
- Samal S, Mohapatra NK, Acharya S, Dey RK. Chelating resins VII: studies on chelating resins of formaldehyde and furfuraldehyde-condensed phenolic Schiff base derived from 4,4′-diaminodiphenylsulphone and o-hydroxyacetophenone. React. Funct. Polym. 1999;42:37–52.
- Kaya İ, Koyuncu S, Çulhaoğlu S. Synthesis and characterization of novel polyazomethines containing perylene units. Polymer. 2008;49:703–714.
- Varganici CD, Durdureanu-Angheluta A, Rosu D, Pinteala M, Simionescu BC. Thermal degradation of magnetite nanoparticles with hydrophilic shell. J. Anal Appl. Pyrol. 2012;96:63–68.
- Park SB, Kim H, Zin WC, Jung JC. Synthesis and properties of polyazomethines having flexible (n-alkyloxy)methyl side chains. Macromolecules. 1993;26:1627–1632.
- Liu CL, Chen WC. Fluorene-based conjugated poly(azomethine)s: synthesis, photophysical properties, and theoretical electronic structures. Macromol. Chem. Phys. 2005;206:2212–2222.
- Cerrada P, Oriol L, Piñol M, Serrano JL, Alonso PJ, Puértolas JA, Iribarren I, Muñoz Guerra S. Influence of hydroxy functionalization and metal cross-linking on fiber properties of liquid-crystalline polyazomethines. Macromolecules. 1999;32:3565–3573.
- More AS, Sane PS, Patil AS, Wadgaonkar PP. Synthesis and characterization of aromatic polyazomethines bearing pendant pentadecyl chains. Polym. Degrad. Stab. 2010;95:1727–1735.
- Jung SH, Lee TW, Kim YC, Suh DH, Cho HN. Synthesis and characterization of fluorene-based poly(azomethines). Opt. Mater. 2003;21:169–173.
- Song F, Ma X, Hou J, Huang X, Cheng Y, Zhu C. (R,R)-salen/salan-based polymer fluorescence sensors for Zn2+ detection. Polymer. 2011;52:6029–6036.
- Murav'ev AA, Pribysh SV, Baidarovtsev YP, Savost'yanov V, Ponomarev AN. Electrophysical properties of metal nitrate complexes with polyacrylamide. Russ. Chem. Bull. 1994;43:809–811.
- Whittell GR, Manners I. Metallopolymers: new multifunctional materials. Adv. Mater. 2007;19:3439–3468.
- Diaham S, Locatelli ML, Lebey T. Conductivity spectroscopy in aromatic polyimide from 200 to 400 °C. Appl. Phys. Lett. 2007;91:122913–122914.
- Jonscher AK. Dielectric relaxation in solids. J. Phys. D: Appl. Phys. 1999;32:R57–R70.
- Sreejith KP, Menon CS, Sudarsanakumar C. Electrical conductivity studies on carbazole thin films. Vacuum. 2008;82:1291–1295.