Abstract
Five new polymers were synthesized by Suzuki coupling reaction using 9,9-dihexylfluorene-2,7-diboronic acid and 3,3′-dibromo-4,4′-dialkoxybiphenyl as the starting materials. The synthesized alternating copolymers were characterized using gel permeation chromatography, 1H and 13C NMR, FT-IR, optical absorption and emission spectroscopic techniques. The thermal behavior of these polymers was analyzed using thermal gravimetric analysis and temperature modulated differential scanning calorimetric studies. The thermal studies show all the polymers were stable up to 300 °C and the glass transition temperature depends on the alkyl chain length. All the polymers have absorption around 330 nm in solution as well as in film, whereas the polymers have emission around 390 nm in both solution and film. Absorption and emission properties of the synthesized materials are not significantly different in solution, but are dramatically different as thin films at different annealing temperatures.
1. Introduction
Synthesis of new conjugated polymers is the focus of research over the past two decades due to their diverse applications in electronic devices such as, field effect transistors,[Citation1] photovoltaic devices,[Citation2] nonlinear optical devices,[Citation3] rechargeable batteries,[Citation3] and light-emitting diodes (LEDs).[Citation4] Homopolymers [Citation5] and copolymers (block copolymers, alternating copolymers, grafted copolymers, and random copolymers) [Citation6–8] have been synthesized and extensively studied. Among the homopolymers, polyfluorenes (PFs) are promising blue-light emitting materials due to their thermal stability and high photoluminescence (PL) quantum efficiency, but it has drawbacks of low color purity and poor color stability.[Citation9] Attempts have been made to improve the optical characteristics of PFs by copolymerization, introducing spirobifluorene groups and introducing substituents at the 9-position of fluorene to induce steric hindrance.[Citation8,10–13] Lee et al. have substituted naphthyl groups in poly(phenylene vinylene) (PPV) and observed better thermal stability and negligible structural defects.[Citation14] Park et al. have synthesized alternating copolymer comprising 9,9-dialkylfluorene and 2,6-dialkoxy naphthalene moieties and observed that the intermolecular interaction was found to be minimum in their PLED devices.[Citation15]
Copolymers with biphenyl building blocks have advantages over phenyl groups due to steric hindrance.[Citation13,15,16] These copolymers with biphenyl moieties are ‘biphenylenevinylene’ systems, comprising unsubstituted biphenyl group with linkage through 4,4′-position exhibit poor solubility in common organic solvents. Lim et al. have synthesized number of alternating copolymers with 9,9-dihexylfluorene and a series of biphenyl groups substituted at their 2,2′-position.[Citation13] They have concluded that substitution at 9-position suppressed the green emission significantly. Similarly, Kwon et al. have synthesized block copolymers with substituted biphenyls for their application in sensing.[Citation17] Deniz et al. reported on the synthesis of copolymers with biphenyl building block with thiophene-benzotriazole-thiophene block. They have studied the luminescence characteristics as a blend with poly(9-vinylcarbazole).[Citation18] Liu et al. have reported the studies on polymers with (biphenylenevinylene butoxyoctyloxy phenylenevinylene) building blocks and observed electroluminescence (EL) at around 530 nm.[Citation19] Very recently, Li and coworkers have reported the synthesis of donor–acceptor polymer with alternating biphenyl–thienyl system and diketopyrrolopyrrole building units for their application in organic photovoltaics.[Citation20]
Introducing alkoxy groups in the biphenyl group and linkage through 3,3′-position may improve the solubility and blue-shifted emission of the polymer.[Citation21] It is fairly well established that the alkyl chain substitution in the polymer backbone alters the glass transition temperature (Tg), thermal characteristics, and film characteristics of the polymers.[Citation22,23] Hence, large number of research groups have attempted introducing alkyl side chain with varying lengths, studied their optical and thermal properties.
Talaie et al. synthesized PPV polymers with varying size of ring in the side chain and the length of side chain.[Citation24] They have reported that longer the chain length with small size of ring showed good efficiency in photoluminescence. Pang et al. synthesized PPV having 1,4-phenylene-alt-1,5-phenylene with different alkyl chain length and demonstrated the active role of side chain length in the optical properties of polymers in ‘cis’ and ‘trans’ form.[Citation25] Egbe et al. synthesized PPE-PPV type polymers, in which they studied the effect of alkyl chain substitution.[Citation26,27] In the present study, series of alternating copolymers with 9,9-dihexylfluorene and 4,4′-dialkoxybiphenyl blocks having different side chain length like octyl, hexyl, butyl, sec-butyl, and isopropyl linked through the 2,7-position of fluorene and 3,3′-position of biphenyl group were synthesized and characterized. The effect of side chain length on the optical properties in thin film at ambient, annealing at different temperature have been studied.
2. Experimental
2.1. Materials
The starting materials, 9,9-dihexylfluorene-2,7-diboronic acid, 4,4′-dihydroxybiphenyl, palladium chloride, 1-bromohexane, 1-bromooctane, and phenyl boronic acid were obtained from Sigma-Aldrich India Private Ltd., India and used as received. N,N-dimethylformamide (DMF), tetrahydrofuran (THF), 1-bromobutane, 2-bromobutane, 2-bromopropane, potassium carbonate were purchased from Sisco Research Laboratories Limited, India. Bromobenzene and tetrabutylammonium bromide were purchased from Merck India Pvt. Ltd., India. Pd(PPh3)4 was synthesized and purified in our laboratory according to reported procedure. All the solvents used in this study were purified by standard procedures.
2.2. Characterization
Thermal gravimetric analysis of alternating copolymers was carried out using instrument SDT Q600 V8-3 Build 101 at heating rate of 20 °C/min in nitrogen atmosphere. Glass transition of polymers was measured using Mettler Toledo DSC (USA) at 5 °C/min in nitrogen atmosphere. Micro-analytical measurements of the monomers were carried out using CHNS Elemental Analyzer-elementar Model Vario EL-III (Elementar Analysensysteme GmbH, Germany). FT-IR spectra of the compounds were measured by Bruker spectrophotometer (Model Tensor 27, Germany). UV–visible absorption spectra of the alternating copolymers in THF and coating polymer films on quartz plate were recorded in Varian Cary 500 Scan UV–vis-NIR Spectrophotometer. The photoluminescence spectra (PL) of the alternating copolymers in solution and as thin films on quartz plate were recorded using Varian Cary Fluorescence Spectrometer. The fluorescence quantum yields in THF were estimated using Quinine sulfate (φf = 0.546) in 0.1 M H2SO4 as the standard. 1H NMR and 13C NMR spectra of monomers and polymers were recorded in CDCl3 as solvent with TMS as internal reference using BRUKER-400 MHz multiprobe NMR spectrometer (Switzerland). The molecular weights of the synthesized polymers were measured by gel permeation chromatography (GPC) using HPLC-GPC systems (Shimadzu model No LC-20AD, Japan). Single-layer device of PLED was fabricated as per the reported procedure.[Citation27] ITO-coated glass substrate was patterned by masking and etching of ITO then washed with DO water, acetone, boiling trichloroethylene, and isopropanol. The washed substrate then dried under vacuum and PEDOT:PSS that filtered through 0.2 μm filter was coated by spin coating at 1000 rpm for 30 s. After the coating, the substrate was dried at 100 °C for 1 h in vacuum. 15 mg/ml solution of polymer in chlorobenzene was then coated by spin coating at 1000 rpm for 1 min and dried at 120 °C for 1 h in vacuum. Aluminum metal was deposited as cathode by thermal evaporation at a pressure below 10−6 torr. The I–V characteristics were studied using Keithley high precision voltmeter (Model 617). Potential was impressed across the electrodes and current was monitored. EL spectrum was measured using Ocean optics HR-2000 having an array of fiber optics probe.
2.3. Synthesis of monomers
Compounds D1–D5 were synthesized using 4,4′-dihydroxybiphenyl and the corresponding alkyl halides (1-bromooctane, 1-bromohexane, 1-bromobutane, 2-bromobutane, and 2-bromopropane) by adapting the same procedure.
In a 250 ml RB flask, 4,4′-dihydroxybiphenyl (9.73 g, 52.2 mmol) in n-butanol was charged. Sodium hydroxide (4.18 g, 104.4 mmol) was added and stirred well for 30 min under a blanket of nitrogen. Alkyl halide (114.8 mmol) was added to the mixture and stirred under reflux for 24 h. After the tenure of the reaction the solution was cooled, filtered, and the solvent was distilled off. The crude product was then crystallized in methanol.
2.3.1. Synthesis of-4,4′-bis(octyloxy)biphenyl (D1)
Yield 73% as white flakes. Melting point 117.0 ± 1.0 °C.
Anal. Calcd for C28H42O2: C, 81.90; H, 10.31. Found: C, 81.54; H, 10.20.
FT-IR (KBr, vmax/cm−1):3044, 2928, 2859, 1606, 1495, 1391, 1259, 1035, 817, 722.
1H-NMR (CDCl3) δ ppm: 7.46–7.5 (d, 4H, J = 8.4 Hz), 6.94–6.97 (d, 4H, J = 8.4 Hz), 4.0 (t, 4H, J = 6.4 Hz), 1.8 (m, 4H), 1.4–1.5 (m, 4H), 1.3 (m, 16H), 0.9 (t, 6H, J = 7.2 Hz). 13C-NMR (CDCl3) δ ppm: 156.31, 127.36, 126.72, 114.44, 67.81, 31.56, 29.10, 29.02, 28.96, 25.79, 22.40, 13.81.
2.3.2. Synthesis of 4,4′-bis(hexyloxy)biphenyl (D2)
Yield 68% as white flakes. Melting point 126.0 ± 1.0 °C Anal. Calcd for C24H34O2: C, 81.31; H, 9.61. Found: C, 81.14; H 9.55 FT-IR (KBr, vmax/cm−1): 3041, 2939, 2866, 1605, 1490, 1391, 1252, 1030, 816, 728. 1H-NMR (CDCl3) δ ppm: 7.46–7.5 (d, 4H, J = 8.4 Hz), 6.94–6.97 (d, 4H, J = 8.4 Hz), 4.0 (t, 4H, J = 6.4 Hz), 1.7–1.8 (m, 4H), 1.481.57 (m, 4H), 1.3–1.4 (m, 8H),0.9–1.0 (t, 6H, J = 7.2 Hz). 13C-NMR (CDCl3) δ ppm: 158.37, 132.85, 127.61, 114.69, 67.59, 31.57, 29.25, 25.71, 22.58, 13.53.
2.3.3. Synthesis of 4,4′-dibutoxybiphenyl (D3)
Yield 68% as white flakes. Melting point 137.0 ± 1.0 °C Anal. Calcd for C20H26O2: C, 80.50; H, 8.78. Found: C, 80.34; H, 8.72FT-IR (KBr, vmax/cm−1): 3042, 2949, 2873, 1606, 1496, 1391, 1247, 1039, 818, 735.1H-NMR (CDCl3) δ ppm: 7.46–7.5 (d, 4H, J = 8.4 Hz), 6.94–6.97 (d, 4H, J = 8.4 Hz), 4.0 (t, 4H), 1.7–1.8 (m, 4H), 1.481.57 (m, 4H), 0.9–1.0 (t, 6H, J = 7.2 Hz). 13C-NMR (CDCl3) δ ppm: 157.97, 133.05, 127.39, 114.47, 67.49, 31.13, 19.03, 13.64.
2.3.4. Synthesis of 4,4′-di-sec-butoxybiphenyl (D4)
Yield 60% as white powder. Melting point 56.5 ± 1.0 °C Anal. Calcd for C20H26O2: C, 80.50; H, 8.78. Found: C, 80.29; H, 8.69. FT-IR (KBr, vmax/cm−1): 3041, 2964, 2870, 1603, 1492, 1375, 1239, 1034, 819.1H-NMR (CDCl3) δ ppm: 7.48–7.5 (d, 4H, J = 8.4 Hz), 6.96–7.0 (d, 4H, J = 8.4 Hz), 4.33–4.4 (m, 2H), 1.63–1.87 (m, 4H), 1.35–1.37 (d, 6H, J = 6.0 Hz).1.02–1.06 (t, 6H, J = 7.2 Hz) 13C-NMR (CDCl3) δ ppm: 157.0, 133.0, 127.4, 115.8, 74.9, 29.0, 19.1, 9.6.
2.3.5. Synthesis of 4,4′-diisopropoxybiphenyl (D5)
Yield 60% as white flakes. Melting point 98.0 ± 1.0 °C Anal. Calcd for C18H22O2: C, 79.96; H, 8.20. Found: C, 79.84; H, 8.14. FT-IR (KBr, vmax/cm−1): 3042, 2977, 2927, 1604, 1492, 1377, 1241, 1029, 823. 1H-NMR (CDCl3) δ ppm: 7.49 (d, 4H, J = 8.4 Hz), 6.98 (d, 4H, J = 8.4 Hz), 4.62 (m 2H), and 1.40 (d 12H, J = 6.0 Hz). 13C-NMR (CDCl3) δ ppm: 157.0, 133.3, 127.7, 116.1, 70.0, 22.1.
2.3.6. Synthesis of 3,3′-dibromo-4,4′-bis(octyloxy)biphenyl (M1)
Compound D1 (20.5 g, 50 mmol) was dissolved in 200 ml glacial acetic acid and stirred well to get a clear solution. Bromine (5.5 ml, 110 mM) was added to the solution and stirred for 3 h. At the tenure of reaction, the mixture was poured to cold water to give crude product. The crude product was washed repeatedly with sodium bicarbonate solution and crystallized in acetone give 3,3′-dibromo-4,4′-bis(octyloxy)biphenyl as a white solid with yield of 92%. Melting point 81.0 ± 1.0 °C. Anal. Calcd for C28H40Br2O2: C, 59.16; H, 7.09. Found: C, 58.97; H, 7.02 FT-IR (KBr, vmax/cm−1): 3040, 2924, 2857, 1602, 1464, 1385, 1257, 1038, 798, 731, 686. 1H-NMR (CDCl3) δ ppm: 7.715–7.20 (d, 2H, J = 2.4 Hz), 7.38–7.41 (dd, 2H, J = 8.4, 2.4 Hz), 6.94–6.96 (d, 2H, J = 8.8 Hz), 4.0–4.1 (t, 4H, J = 6.4 Hz), 1.85–1.9 (m, 4H), 1.51–1.58 (m, 4H), 1.3–1.38 (m, 16H), 0.9 (t, 6H, J = 7.2 Hz). 13C-NMR (CDCl3) δ ppm: 154.48, 132.98, 131.11, 126.21, 112.98, 112.36, 69.08, 31.52, 29.03, 28.96, 28.79, 25.71, 22.40, 13.85.
Compounds M2–M5 were synthesized using corresponding compounds (D2–D5) adapting the same procedure as above.
2.3.7. Synthesis of 3,3′-dibromo-4,4′-bis(hexyloxy)biphenyl (M2)
Yield 90% as pale white solid. Melting point 79.0 ± 1.0 °C. Anal. Calcd for C24H32Br2O2: C, 56.27; H, 6.30. Found: C, 56.14; H, 6.34 FT-IR (KBr, vmax/cm−1): 3040, 2944, 2855, 1599, 1470, 1382, 1255, 1033, 795, 732, 685. 1H-NMR (CDCl3) δ ppm: 7.71–7.72 (d, 2H, J = 2.4 Hz), 7.3–7.4 (dd, 2H, J = 8.4, 2.4 Hz), 6.92–6.94 (d, 2H, J = 8.8 Hz), 4.0–4.1 (t, 4H, J = 6.4 Hz), 1.83–1.9 (m, 4H), 1.53–1.56(m, 4H), 1.3–1.38 (m, 8H), 0.9 (t, 6H, J = 7.2 Hz). 13C-NMR (CDCl3) δ ppm: 154.76, 133.26, 131.38, 126.46, 113.27, 112.63, 69.34, 31.47, 29.02, 25.61, 22.54, 13.98.
2.3.8. Synthesis of 3,3′-dibromo-4,4′-dibutoxybiphenyl (M3)
Yield 90% as pale white solid. Melting point 68.0 ± 1.0 °C. Anal. Calcd for C20H24Br2O2: C, 52.65; H, 5.30. Found: C, 52.64; H, 5.18 FT-IR (KBr, vmax/cm−1): 3039, 2949, 2855, 1599, 1462, 1378, 1256, 1034, 796, 732, 686.1H-NMR (CDCl3) δ ppm: 7.71–7.72 (d, 2H, J = 2.4 Hz), 7.38–7.4 (dd, 2H, J = 8.4, 2.4 Hz), 6.92–6.94 (d, 2H, J = 8.4 Hz), 4.0 (t, 4H, J = 6.4 Hz), 1.81–1.89 (m, 4H), 1.52–1.59 (m, 4H), 0.9–1.0 (t, 6H, J = 7.2 Hz). 13C-NMR (CDCl3) δ ppm: 153.89, 133.01, 131.13, 126.22, 113.00, 112.35, 68.76, 30.89, 18.97, 13.58.
2.3.9. Synthesis of 3,3′-dibromo-4,4′-di-sec-butoxybiphenyl (M4)
Yield 73% as pale white solid. Melting point 55.5 ± 1.0 °C. Anal. Calcd for C20H24Br2O2: C, 52.65; H, 5.75. Found: C, 52.43; H, 5.62 FT-IR (KBr, vmax/cm−1): 3085, 2971, 2928, 1599, 1476, 1375, 1261, 1034, 802, 678.1H-NMR (CDCl3) δ ppm: 7.72–7.73 (d, 2H, J = 2.4 Hz), 7.38–7.4 (dd, 2H, J = 8.4, 2.4 Hz), 6.93–6.96 (d, 2H, J = 8.4 Hz), 4.3–4.4 (m, 2H), 1.70–1.86 (m, 4H), 1.36–1.39 (d, 6H, J = 6.0), 1.0–1.07 (t, 6H, J = 7.2 Hz) 13C-NMR (CDCl3) δ ppm: 153.78, 113.04, 131.28, 126.18, 115.01, 113.63, 76.85, 28.88, 19.00, 9.47.
2.3.10. Synthesis of 3,3′-dibromo-4,4′-diisopropoxybiphenyl (M5)
Yield 70% as pale white solid. Melting point 67.5 ± 1.5 °C. Anal. Calcd for C18H20Br2O2: C, 50.49; H, 4.71. Found: C, 50.36; H, 4.72. FT-IR (KBr, vmax/cm−1): 3057, 2976, 2925, 1598, 1473, 1375, 1250, 1035, 809, 678. 1H-NMR (CDCl3) δ ppm: 7.73–7.74 (d 2H, J = 2.4 Hz), 7.41 (dd 2H, J = 8.4, 2.4 Hz), 6.98 (d 2H, J = 8.4 Hz), 4.61 (m, 2H), and 1.44 (d, 12H, J = 6.0 Hz). 13C-NMR (CDCl3) δ ppm: 153.8, 133.5, 131.5, 126.4, 115.8, 114.1, 72.3, 22.1.
2.4. Synthesis of polymers
2.4.1. Poly(3,3′-(4,4′-dioctyloxybiphenyl) alt (2,7-(9,9-dihexylfluorene))) (P1)
The polymers were synthesized using palladium-catalyzed Suzuki coupling reaction. 9,9-dihexylfluorene-2,7-diboronic acid (0.85 g, 2.0 mmol), 3,3′-dibromo-4,4′-bis(octyloxy) biphenyl (1.136 g, 2 mmol), and 2 M solution of K2CO3 was stirred in 100 ml anhydrous THF under nitrogen atmosphere. The catalyst Pd(PPh3)4 (0.110 g) was added to the stirred solution and heated at 80 °C for 12 h. After 12 h, bromobenzene (0.05 equivalent) and phenylboronic acid (0.05 equivalent) with small amounts of catalyst were added to the stirred solution for end capping, after 3 h, the reaction mixture was concentrated and poured in anhydrous methanol gives polymer as gray–white powder. Purification of the polymer was achieved by repeated dissolution in THF and precipitation with methanol.
Yield 80% as pale white solid. FT-IR (KBr, vmax/cm−1): 3026, 2925, 2857, 1604, 1460, 1376, 1235, 1037, 821, 726. 1H-NMR (CDCl3) δ ppm: 7.7 (2H), 7.6–7.7 (4H), 7.5 (4H), 7.1 (2H), 4.0 (4H), 2.0 (4H), 1.7–1.8 (4H), 1.4 (4H), 1.3 (16H), 1.1 (16H), 0.8 (6H), 0.7 (6H).
Other four polymers (P2–P5) were also synthesized by same procedure.
2.4.2. Poly(3,3′-(4,4′-dihexyloxybiphenyl) alt (2,7-(9,9-dihexylfluorene))) (P2)
Yield 80% as pale white solid. FT-IR (KBr, vmax/cm−1): 3036, 2930, 2859, 1602, 1465, 1379, 1242, 1017, 813, 732. 1H-NMR (CDCl3) δ ppm: 7.8(2H), 7.6–7.7 (4H), 7.5 (4H), 7.1 (2H), 4.0 (4H), 2.0 (4H), 1.7–1.8 (4H), 1.4 (4H), 1.3 (8H), 1.1 (16H), 0.89 (6H), 0.76 (6H).
2.4.3. Poly(3,3′-(4,4′-dibutoxybiphenyl alt (2,7-(9,9-dihexylfluorene))) (P3)
Yield 80% as pale white solid. FT-IR (KBr, vmax/cm−1): 3031, 2924, 2856, 1605, 1461, 1355, 1238, 1028, 815, 735. 1H-NMR (CDCl3) δ ppm:7.8(2H), 7.6–7.7 (4H), 7.5 (4H), 7.1 (2H), 4.0 (4H), 2.0 (4H), 1.7–1.8 (4H), 1.4 (4H), 1.1 (16H), 0.89 (6H), 0.76 (6H).
2.4.4. Poly((3,3′-(4,4′-di-sec-butoxy)biphenyl) alt (2,7-(9,9-dihexylfluorene))) (P4)
Yield 80% as pale white solid. FT-IR (KBr, vmax/cm−1): 3032, 2926, 2858, 1606, 1470, 1371, 1244, 1037, 921, 817, 750. 1H-NMR (CDCl3) δ ppm: 7.8 (2H), 7.6–7.7 (4H), 7.5 (4H), 7.1 (2H), 4.3 (2H), 2.0 (4H), 1.7–1.8 (4H), 1.3 (6H), 1.1 (16H), 0.9 (6H), 0.76 (6H).
2.4.5. Poly((3,3′-(4,4′-diisopropoxy)biphenyl) alt (2,7-(9,9-dihexylfluorene))) (P5)
Yield 80% as pale white solid. FT-IR (KBr, vmax/cm−1): 3037, 2925, 2859, 1602, 1470, 1373, 1243, 1011, 945, 813, 732. 1H-NMR (CDCl3) δ ppm: 7.8(2H), 7.6–7.7 (4H), 7.5 (4H), 7.1 (2H), 4.5 (2H), 2.0 (4H), 1.3 (12H), 1.1 (16H), 0.76 (6H).
3. Results and discussion
3.1. Synthesis and characterization of polymers
Synthesis of monomers 3,3′-dibromo-4,4′-dialkoxybiphenyl was carried out in two steps (Scheme ). In the first step, 4,4′-dihydroxybiphenyl was alkylated by Williamson ether synthesis using sodium hydroxide as base and 1-butanol as solvent, using suitable alkyl bromide as the reagent. 1H and 13C NMR spectra of D4 are presented in Figures and . 4,4′-Dialkoxybiphenyl was then brominated using bromine in acetic acid gave the corresponding 3,3′-dibromo-4,4′-bis(alkoxy)biphenyl functional monomers (M1–M5) in good yield. These monomers were characterized using NMR (1H and 13C) spectral measurements and the purity was checked using HPLC. 1H and 13C NMR spectra of M4 are presented in Figures and , whereas 13C NMR spectra of M1 are shown in Figure . The NMR data, FT-IR, and C, H, and N analysis confirmed the formation of the functional monomers D1–D5 and M1–M5. Polymerization of these monomers was carried out by Suzuki reaction with 9,9-dihexylfluorene-2,7-diboronic acid under argon atmosphere using anhyd. THF as solvent and Pd(PPh3)4 as catalyst (Scheme ). The polymers formed during the coupling reaction (P1–P5) were precipitated from the THF solution by methanol and purified by repeated dissolution in THF followed by precipitation.
Figure 13. Optical absorption and photoluminescence spectra of pristine and annealed thin films at 50 and 70 °C of the synthesized polymers (A) P1 and (B) P4.
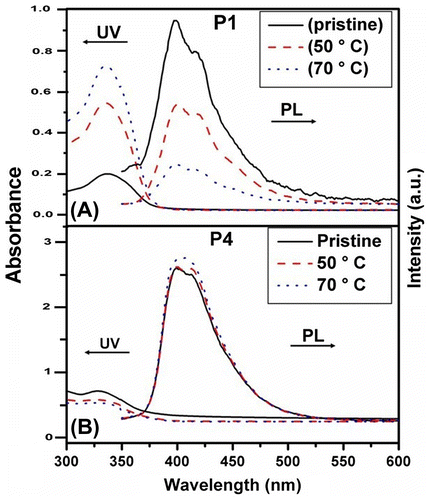
Figure 14. Photoluminescence spectra of pristine and annealed thin films of the synthesized polymers P1–P5.
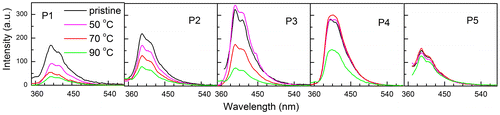
Figure 15. (A) Electroluminescence spectra of P5 using a single-layer cell with PEDOT:PSS-coated ITO anode and aluminum cathode and (B) the I–V characteristics of the PLED device.
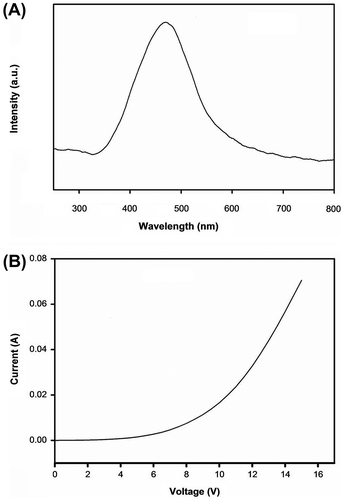
NMR spectrum of P1 is presented in Figure . It can be seen from the figure that aromatic protons corresponding to 9,9-dihexylfluorene and 4,4′-dialkoxybiphenyl blocks and alkyl chain were observed indicating the formation of the polymer. Further, all these peaks were found to broaden due to the polymer formation. The molecular weight of the polymers was estimated by GPC using THF as solvent and polystyrene as the standard. The molecular weights of these polymers were in the range of 15,600–4600 Daltons with the polydispersity between 1.38 and 2.07 (Table ). FT-IR spectrum of P2 is shown in Figure and can be seen that boronic acid functional group of the 9,9-dihexylfluorene is absent. Further, the peak at 3031 cm−1 corresponds to aromatic C–H bond vibration, 2923 cm−1 and 2855 cm−1 correspond to aliphatic C–H asymmetric and symmetric stretching.
Table 1. Physical results of the synthesized polymers.
3.2. Thermal properties of the polymers
The thermal properties of the synthesized polymers were studied under N2 atmosphere. The thermograms of P1 and P4 are presented in Figure . It can be seen from the figure that the weight loss for these materials was found to be <1% at 200 °C and <2% at 300 °C. Further, there is a major weight loss from 350 to 500 °C. The weight loss below 200 °C may be due to the loss of adsorbed organic solvents in the polymer and the thermal process from 350 to 500 °C is due to thermal degradation of the polymers into volatile components. Similar characteristics were observed for other polymers (P2, P3, and P5). Thermal modulated DSC was measured to estimate the glass transition (Tg) of the synthesized polymers. P1 has the onset of Tg at around 60.5 °C and glass transition temperatures vary as P1 < P2 < P3 < P4 < P5 (Table ). Tg of the polymers decreases with increase in the chain length,[Citation22] further, it can be seen that Tg value increases for the branched alkyl chain bearing polymers than the linear alkyl chain. From the thermal studies, it can be concluded that these synthesized polymers are stable up to 300 °C and the Tg varies as a function of the alkoxy group in the biphenyl group.
3.3. Optical properties of polymers in solution and as thin films
The optical characteristics, namely, UV–vis absorption as well as photoluminescence spectra of the polymers was measured in solution as well as thin films on quartz substrate. Absorption spectra of the polymers in THF solution are presented in Figure . Dialkoxybiphenyl biphenyls give rise to absorption at around 292 and 257 nm, whereas 9,9-dihexylfluorene exhibits absorption at around 298 and 250 nm. It can be seen from the figure that the synthesized polymers materials have a broad absorptions in the range of 250–290 nm and 310–365 nm with the maxima at around 330 nm, which is red shifted when compared with the corresponding monomers, indicating extended conjugation of ‘biphenyl–fluorene’ chromophore and the absorption is due to the π–π* transition. It may be noted that similar systems reported by Donat-Bouillud et al. using poly 4,4′ biphenyl–fluorene gave rise to absorption at 365 nm and emission at higher wavelength at around 424 and 450 nm.[Citation28] All the synthesized polymers exhibit similar absorption characteristics with marginal blue shift in the case of P4 and P5. PL spectra of these polymers in THF solution are presented in Figure . The synthesized polymers have broad emission spectra between 350 and 475 nm with maxima at around 390 nm and tailing in the red region. The emission essentially arises due to the ‘biphenyl–fluorene’ chromophore. The monomers, 9,9-dihexylfluorene and 4,4′-dibutoxybiphenyl, have emission maxima at around 318 and 350 nm, respectively. The red-shifted emission in the polymer again indicates the extended π–π interaction between fluorene and biphenyl building blocks in the polymer. The quantum yield of emission for these polymers was measured and found to be high, ca. 0.75 (Table ). There is marginal change in the PL spectra among the synthesized polymers. Though the emission characteristics of the synthesized polymers are similar, a close observation of Figure reveals that emission of P1 is red shifted among P1–P5. The prominent shoulders of P1 at around 415 and 430 nm are nearly absent for the other polymers (in branched chain polymers P4 and P5, there is prominent shoulder at around 360 nm). This observation suggests there may be weak interchain interaction in the case of linear alkyl chain-bearing materials. However, the degree of such interaction is minuscule as the change in the emission maxima was found to be insignificant. However, the absorption as well as emission was observed to be dependent on the nature of alkyl substituent in the thin film of the polymers. Octyl chains in the polymer network seem to shift the absorption and emission maxima to higher wavelength. This may arise due to interchain interaction in P1. Similar behavior has been observed in the annealed film of P1, P2, and P3.
Table 2. Optical properties of the synthesized polymers.
3.4. Annealing effect of the polymer thin films on their photophysical properties
Thin films of the synthesized materials were spin coated on optically clear quartz plate using THF solution. The thin films thus obtained were allowed to dry at ambient condition overnight. The pristine films thus obtained were annealed at different temperatures and the optical properties were studied. The Optical absorption spectra of pristine films of P1–P5 are shown in Figure . A closure look of the figure indicates the following. Firstly, the absorption maximum of the thin films of P1 is at around 336 nm, slightly red shifted than the solution spectra, wherein the other polymers did not show significant shift. Figure depicts the emission spectra of the pristine thin film of polymers (P1–P5). As in the case of absorbance spectra of the thin film, the PL spectra also exhibit red-shifted emission when compared with the solution spectral characteristics. However, the absorption spectra as well as the emission spectra showed significant differences on annealing the film. Figure depicts the optical absorbance and emission spectra of P1 and P4. The intensity of absorbance value in thin film of P1 was found to increase on annealing from ambient to 70 °C. It is worth to recall that the Tg value of P1 is at around 60 °C; hence, annealing above the glass transition temperature the film may become more compact. The thickness of the film may decrease and hence, the increase in the absorbance value. Similar trend has been observed for P2 and P3 thin films as well. However, the thin films of P4 and P5 that has sec-butyl and isopropyl chains in the polymer behave differently. The absorbance values did not experience significant increase, indicating these films did not undergo considerable changes on annealing. These observations may indicate that the linear alkyl chains may facilitate the decrease the thickness of the film on annealing, whereas the branched chain containing polymers do not. The decrease in the thickness of the film may result in the increase in the interchain interaction and hence, manifest in the PL of the annealed thin films of these materials. The PL intensity of P1 decreased with the increase in the annealing temperature drastically. When the film was annealed at about 70 °C, the PL intensity was around 15% of the original intensity, whereas the absorbance value was found to increase. Similar observations were made for P2 and P3 thin films annealed at high temperature. Hence, it may be clear that polymers with linear side chain the thickness of the film decreased on annealing and the PL intensity decreased dramatically due to enhanced interchain interaction. However, this is in contrary to the polymers having branched chains (P4 and P5) in that the absorbance values did not experience significant increase on annealing and also the PL intensity did not decrease on annealing up to 70 °C. This behavior may also take place due to the lower glass transition temperature of the polymers bearing linear chains. Hence, the thin films were annealed at 90 °C above the Tg of these polymers (Figure ). It was noted that P1 the PL intensity dropped to almost 10% of the original value and P2 and P3 also showed significant decrease in the PL intensity. There was marginal decrement of PL for the annealed (90 °C) film of P4, whereas P5 thin films did not experience significant changes on annealing. Hence, it can be concluded that the optical properties of thin films of polymers with linear chain were adversely affected on annealing. The polymer materials were extracted from the films annealed at 70 °C and the optical absorbance and emission of the extracts did not experience significant change. Hence, it may be concluded that the polymers did not degrade during annealing.
3.5. EL studies
The EL single-layer devices using PEDOT:PSS/ITO-coated glass as the anode and aluminum as the cathode with either P1 or P5 as the active layer were constructed. It was noted that the EL device with P1 as the active layer showed very poor emission characteristics, wherein the device with P5 as the active material showed EL characteristics (Figure ). The I–V characteristics of the device indicate the turn-on onset voltage is around 5.0 V and with an impressed voltage of 9.0 V the EL of the device was significant. This material gave rise to broad EL at 473 nm. EL device with P4 material also exhibits EL with the similar emission maxima with lower intensity. In the case of P2 and P3, the emission intensity is very feeble. This observation is in conformity to the PL studies on the annealed thin films of these polymers. It may be noted that P1, P2, and P3, the Pl efficiency dropped to 10% of the pristine film and hence, EL also is very feeble. However, the similar behavior of I–V characteristics of these devices indicates the formation of hole and electron in all these cases. Due to interchain interaction, the emission was not observed for P1–P3. In the case of P5, there is significant emission in PL and same is observed in EL as well.
4. Conclusion
Polymers with 9,9-dihexylfluorene and 4,4′-dialkoxy-3,3′-biphenyl moieties as building blocks with varying alkyl chains in the biphenyl group were synthesized and characterized using NMR, thermal, and optical methods. All the polymers have absorbance at around 330 nm and PL emission at around 390 nm in solution. The pristine films exhibit absorbance at around 336 nm and PL at around 400 nm as thin films. Both absorbance and PL emission did not show considerable shift with in the series of polymers in solution and pristine thin film. The absorbance and emission studies of the annealed films indicate the nature of alkyl chain in the polymers have significant effect on the optical properties. In linear alkyl chain polymer, the absorbance values increased on annealing and the PL emission intensity decreased. However, in the case of branched chain polymer, there were no significant changes on the optical properties on annealing of their films. The thermal and optical properties of the polymers have shown polymers with branched chains are promising materials for blue emission in polymer LEDs and further process on device fabrication is under progress.
Acknowledgment
One of the authors, V.S. thanks CSIR, New Delhi for the Senior Research Fellowship and Tini, CSIR-CECRI for the Research Internship. The authors thank Director, CSIR-Central Electrochemical Research Institute, Karaikudi for his constant encouragement and permission to publish this work. The work is sponsored (NWP 025) by the Council of Scientific and Industrial Research, India.
References
- Sirringhaus H, Tessler N, Friend RH. Integrated optoelectronic devices based on conjugated polymers. Science. 1998;280:1741–1744.10.1126/science.280.5370.1741
- Son HJ, Wang W, Xu T, Liang Y, Wu Y, Li G, Yu L. Synthesis of fluorinated polythienothiophene-co-benzodithiophenes and effect of fluorination on the photovoltaic properties. J. Am. Chem. Soc. 2011;133:1885–1894.10.1021/ja108601g
- Rehan HH. A new polymer/polymer rechargeable battery: polyaniline/LiClO4(MeCN)/poly-1-naphthol. J. Power Sources. 2003;113:57–61.10.1016/S0378-7753(02)00485-8
- Grimsdale AC, Leok Chan K, Martin RE, Jokisz PG, Holmes AB. Synthesis of light-emitting conjugated polymers for applications in electroluminescent devices. Chem. Rev. 2009;109:897–1091.10.1021/cr000013v
- Mori T, Kijima M. Synthesis and optical properties of blue luminescent poly(2,6-naphthalene)s. J. Polym. Sci., Part A: Polym. Chem. 2008;46:4258–4263.10.1002/(ISSN)1099-0518
- Tan Y, Gu Z, Tsuchiya K, Ogino K. Synthesis and luminescent properties of block copolymers based on polyfluorene and polytriphenylamine. Polymer. 2012;53:1444–1452.10.1016/j.polymer.2012.02.021
- Pu K-Y, Chen Y, Qi X-Y, Qin C-Y, Chen Q-Q, Wang H-Y, Deng Y, Fan QL, Huang YQ, Liu SJ, Wei W, Peng B, Huang W. Synthesis of grafted poly(p-phenyleneethynylene) with energy donor–acceptor architecture via atom transfer radical polymerization: towards nonaggregating and hole-facilitating light-emitting material. J. Polym. Sci., Part A: Polym. Chem. 2007;45:3776–3787.10.1002/(ISSN)1099-0518
- Li Y, Ding J, Day M, Tao Y, Lu J, D’Iorio M. Synthesis and properties of random and alternating fluorene/carbazole copolymers for use in blue light-emitting devices. Chem. Mater. 2004;16:2165–2173.10.1021/cm030069g
- Millard IS. High-efficiency polyfluorene polymers suitable for RGB applications. Synth. Met. 2000;111–112:119–123.10.1016/S0379-6779(99)00321-5
- Lu J, Tao Y, D’Iorio M, Li Y, Ding J, Day M. Pure deep blue light-emitting diodes from alternating fluorene/carbazole copolymers by using suitable hole-blocking materials. Macromolecules. 2004;37:2442–2449.10.1021/ma030535n
- Klärner G, Davey MH, Chen W-D, Scott JC, Miller RD. Colorfast blue-light-emitting random copolymers derived from di-n-hexylfluorene and anthracene. Adv. Mater. 1998;10:993–997.10.1002/(ISSN)1521-4095
- Yu WL, Pei J, Huang W, Heeger AJ. Spiro-functionalized polyfluorene derivatives as blue light-emitting materials. Adv. Mater. 2000;12:828–831.10.1002/(ISSN)1521-4095
- Lim S-F, Friend RH, Rees ID, Li J, Ma Y, Robinson K, Holmes AB, Hennebicq E, Beljonne D, Cacialli F. Suppression of green emission in a new class of blue-emitting polyfluorene copolymers with twisted biphenyl moieties. Adv. Funct. Mater. 2005;15:981–988.10.1002/(ISSN)1616-3028
- Lee NHS, Chen Z-K, Huang W, Xu Y-S, Cao Y. Synthesis and characterization of naphthyl-substituted poly(p-phenylenevinylene)s with few structural defects for polymer light-emitting diodes. J. Polym. Sci., Part A: Polym. Chem. 2004;42:1647–1657.10.1002/(ISSN)1099-0518
- Park J-W, Park SJ, Kim Y-H, Shin D-C, You H, Kwon S-K. Pure color and stable blue-light emission-alternating copolymer based on fluorene and dialkoxynaphthalene. Polymer. 2009;50:102–106.10.1016/j.polymer.2008.10.056
- Ma D, Hummelgen IA, Li RWC, Gruber J. Charge injection and transport in poly(4,4′- biphenylenevinylene). J. Phys. D: Appl. Phys. 2000;33:1376–1379.10.1088/0022-3727/33/11/317
- Kwon NY, Jang G, Kim J, Kim D, Lee TS. Oligonucleotide-mediated aggregation of a cationic conjugated polymer for fluorescent detection of mercury ions in an aqueous medium. J. Polym. Sci., Part A: Polym. Chem. 2013;51:2393–2400.10.1002/pola.26624
- Deniz TK, Apaydin DH, Ozelcaglayan AC, Toppare LK, Cirpan A. Photo-and electroluminescence behavior of biphenyl containing conjugated polymer. Turk. J. Chem. 2013;37:538–546.
- Liu Z, Yuan Y, Wen X, Zhang J, Lei G, Zhang P. Synthesis, characterization, photoluminescent, and electroluminescent properties of poly(biphenylenevinylene-alt-methoxyoctyloxyphenylenevinylene). Polym. Bull. 2013;70:1221–1235.10.1007/s00289-012-0843-6
- Li WW, Furlan A, Roelofs WSC, Hendriks KH, van Pruissen GWPV, Wienk MM, Janssen RAJ. Wide band gap diketopyrrolopyrrole-based conjugated polymers incorporating biphenyl units applied in polymer solar cells. Chem. Commun. 2014;50:679–681.10.1039/c3cc47868h
- Xu JM, Ng SC, Chan HSO. Polymers containing alternating 1,4-bis(3-octyl-2,5-thienylene)phenylene and 2,5-pyridyl, 4,4′-biphenylene or phenylene repeating units. Acta Mater. 2003;51:1743–1754.10.1016/S1359-6454(02)00573-6
- Kunisada H, Yuki Y, Kondo S, Miyatake J, Maeda C. Synthesis and side-chain crystallization of new comb-like polymers from 2-amino-4-(N-alkylanilino)-6-isopropenyl-1,3,5-triazines. Polym. J. 1990;22:559–566.10.1295/polymj.22.559
- Doi S, Kuwabara M, Noguchi T, Ohnishi T. Organic electroluminescent devices having poly(dialkoxy-p-phenylene vinylenes) as a light emitting material. Synth. Met. 1993;57:4174–4179.10.1016/0379-6779(93)90577-J
- Talaie A, Lee YK, Huh G, Kim KM, Jeong HY, Choo DJ, Lee JY, Jang J. Macromolecular architecture of new polymers via construction of new side groups within PPV chemical structure to enhance its properties for flat-panel displays. Mater. Sci. Eng. B. 2001;85:177–181.10.1016/S0921-5107(01)00606-7
- Liao L, Pang Y. Poly[(1,4-phenylenevinylene)-alt-(1,3-phenylenevinylene)]s with different length of side chain: their synthesis and optical properties. Synth. Met. 2004;144:271–277.10.1016/j.synthmet.2004.04.001
- Egbe DAM, Carbonnier B, Ding L, Mühlbacher D, Birckner E, Pakula T. Supramolecular ordering, thermal behavior, and photophysical, electrochemical, and electroluminescent properties of alkoxy-substituted yne-containing poly(phenylene−vinylene)s. Macromolecules. 2004;37:7451–7463.10.1021/ma0488111
- Tekin E, Egbe DAM, Kranenburg JM, Ulbricht C, Rathgeber S, Birckner E. Effect of side chain length variation on the optical properties of PPE-PPV hybrid polymers. Chem. Mater. 2008;20:2727–2735.10.1021/cm703312u
- Donat-Bouillud A, Lévesque I, Tao Y, D’Iorio M, Beaupré S, Blondin P. Light-emitting diodes from fluorene-based π-conjugated polymers. Chem. Mater. 2000;12:1931–1936.10.1021/cm0001298