Abstract
The current study describes the synthesis and characterization of dual environment-responsive semi-interpenetrating hydrogels of polyhydroxyethyl methacrylate (pHEMA) and methyl cellulose (MC). HEMA was polymerized using free radical polymerization technique. Different proportions of MC were incorporated within the pHEMA matrix to induce environment-sensitive property. The microstructures of the hydrogels were studied under bright field microscopy. The hydrogels were thoroughly characterized using XRD, FTIR, swelling, mechanical and electrical studies. The micrographs of the hydrogels suggested formation of biphasic system. At lower proportions of MC, the hydrogels were oil-in-water type of formulations. An increase in the MC content resulted in the formation of bicontinuous biphasic formulations. Mechanical analysis suggested viscoelastic solid nature of the hydrogels. An increase in the MC content resulted in the increase in the viscous component. The electrical studies suggested resistive dominant behavior of the hydrogels. Thermal studies suggested better moisture retention capacity of the MC containing hydrogels. The hydrogels were found to be biocompatible in nature.
1. Introduction
Hydrogels may be defined as the polymeric constructs having the ability to absorb and retain water within its structure. The polymeric constructs usually contain hydrophilic groups which allow positive interactions with the water molecules. This phenomenon helps holding the water molecules within the polymeric constructs.[Citation1] Hydrogels have been used for designing various biomedical products, e.g. drug delivery matrices,[Citation2] tissue engineering scaffolds,[Citation3] artificial skin grafts [Citation4], and cosmetic formulations. In the last decade, there has been a great interest in designing stimuli-responsive hydrogels.[Citation5] This is because of the fact that these hydrogels have shown a great potential in controlled delivery and tissue engineering applications.
Poly(2-hydroxyethylmethacrylate) (pHEMA) was first used for synthesizing hydrogels in the year of 1960, and has since been used for biomedical applications.[Citation6] pHEMA is a synthetic biocompatible polymer and has been greatly explored for making contact lenses.[Citation7] Apart from this, pHEMA-based hydrogels have been used as delivery matrices for the delivery of different categories of drugs (e.g. anticancer, antimicrobial). pHEMA has been used in conjunction with various other biopolymers (e.g. gelatin, dextrin, and collagen) for developing composite hydrogels.[Citation8–10]
Methyl cellulose (MC) is a semi-synthetic polysaccharide. It contains hydrophobic domains within its polymeric structure which helps in designing reversible physical gels. This property of the MC has been explored to design temperature-sensitive biomedical products (e.g. scaffolds and drug-delivery vehicles).[Citation11–14] In general, the aqueous solutions of MC have lower viscosities at low temperatures. In other words, a MC solution will show a lower viscosity at room temperature and will form a high-viscous solution (gels) at body temperature.[15]
In general, the celluloses (neutral in charge) have been used as enteric-coated polymers. This can be explained by the lower hydration of the celluloses at low pHs. An increase in the pH towards neutral pH results in the improved hydration of the celluloses. A further increase in the pH towards basic pHs has been reported to induce degradation of the celluloses.[Citation16] Since MC is a nonionic semi-synthetic cellulose, it is expected that incorporation of MC into the hydrogel matrices may also impart pH-sensitive behavior.
Taking inspiration from the above, we hypothesized that novel dual environment-responsive hydrogels can be obtained by preparing semi-IPN hydrogels of pHEMA and MC. Thus, an attempt was made to develop the dual environment-responsive hydrogels which may have wide biomedical applications, such as, controlled drug release,[17] glucose sensors,[Citation18] textile industry,[Citation19] and thermo-chemotherapy.[Citation20] HEMA was cross-linked using free radical polymerization, whereas MC was not cross-linked. The developed hydrogels were thoroughly characterized by FTIR spectroscopy, XRD, and mechanical studies. The environment-sensitive behavior of the semi-IPN hydrogels was studied by conducting swelling studies at different pH and temperature conditions. Further, to ascertain the biocompatible nature of the hydrogels cytocompatibility test was conducted using HaCaT cells.
2. Materials and methods
HEMA and MC were purchased from HiMedia laboratories Pvt. Ltd. (Mumbai, India). N,N,N,N-tetramethyl ethylenediamine (TEMED) and ammonium persulfate (APS) were procured from Sisco Researh Laboratories Pvt. Ltd. (Mumbai, India) and Rankem (New Delhi, India), respectively. Distilled water was used throughout the study.
2.1. Preparation of the MC solutions
A stock solution of 2% (w/v) MC was prepared by dissolving 1 g of MC in 40 ml of water (50 °C). The final volume was made up to 50 ml using water (50 °C). The MC solutions were diluted using sufficient amount of water so as to have 1.5, 1.0, and 0.5% (w/v) MC solutions. All the solutions were incubated at 4 °C for 30 min.
Thereafter, the solutions were stirred thoroughly in a magnetic stirrer (350 rpm) to obtain homogenous solutions. Freshly prepared MC solutions were used for making the composite hydrogels.
To prepare the hydrogels, HEMA (0.45 g) and MC solutions (1 g) were thoroughly mixed in a magnetic stirrer (100 rpm). Subsequently, 20% (w/v) APS (0.025 ml) and 40% (v/v) TEMED (0.025 ml) solutions were sequentially added dropwise to the homogenous mixture with continuous stirring. The above mixture was incubated for 24 h at 37 °C (under nitrogen environment) to allow cross-linking of HEMA. This resulted in the formation of the semi-IPN hydrogels. The compositions of the hydrogels have been tabulated in Table . The prepared hydrogels were stored at 4 °C for further studies. The sample HM1, which did not contain any MC, was used as the control. The properties of the MC-based hydrogel were compared with HM1.
Table 1. Composition of the hydrogels.
To calculate the percentage conversion of the monomers into insoluble polymer, prepared hydrogels were washed thoroughly in water to remove all the unreacted monomers. They were then dried in vacuum oven at 37 °C for 2 days. The percentage conversion was then calculated based on the ratio of the total weight of the xerogel after drying and weight of the monomer in the initial mixture.
2.2. Degree of polymerization
Fresh hydrogels were prepared as per the above-mentioned procedure in cylindrical shapes (diameter = 13.1 mm, height = 17.4 mm). These were then kept at 37 °C for 24 h to allow cross-linking of HEMA. The prepared hydrogels were used for calculating the average molecular weight of the polymer in between the crosslink points using the Flory–Rehner equation:[Citation21,22]
where is the specific volume of the hydrogel, M is the primary molecular mass, and Mc is the average molecular mass between cross-links and the network parameters. V is the molar volume of the solvent (water, 18 cm3/mol), v2,s is the polymer volume fraction in the swollen state determined as roughly the inverse of the equilibrium swelling ratio.
is the Flory parameter describing the polymer–solvent interaction.[Citation23]
The degree of polymerization (X) was determined as per the equation given below:
2.3. Microscopic studies
The microstructure of the hydrogels was visualized under a bright field microscope (LEICA- DM750 equipped with ICC 50-HD camera, Germany). For the analysis, one drop of the reaction mixture was put over a glass slide and covered with a cover slip. The slide was then put in a nitrogen chamber and the reaction was allowed to proceed as per the method described above. The thin films, so formed, were analyzed under the microscope.
2.4. XRD studies
The hydrogel were prepared as films for the XRD analysis. Films were converted into xerogels by drying the sample in a vacuum dryer (37 °C, −0.04 MPa). The XRD analysis was done in the 2θ range of 5°–50°. The source of the X-ray was Cu-Kα, which was operated at (40 kV, 40 mA).
2.5. FTIR spectroscopic studies
The chemical interactions between the functional groups of the pHEMA and MC were analyzed by FTIR studies. For the study, the hydrogel films were used. The FTIR spectrometer (Alpha-E, Bruker, Germany), attached with a ZnSe ATR cell, was used for the study. The analyses were done in the wavenumber range of 4000–500/cm. The original spectra were smoothened and the baseline was corrected using the OPUS software.
2.6. Swelling studies
The swelling study of the hydrogels was performed to understand the pH and temperature-sensitive nature of the hydrogels. The pHs of the swelling media were 1.2 (citrate buffer), 7.0 (phosphate buffer), and 9.0 (phosphate buffer). The studies were conducted at 4, 25, and 37 °C. Hydrogels of dimension (diameter = 19.5 mm and height = 1.5 mm) were used for the swelling studies. Hydrogels were accurately weighed in a highly sensitive weighing balance (Wi). The hydrogels were put in 12 ml of the swelling media, kept in 6-well plate. Subsequently, the well-plates were incubated at the preset temperature cabinets. At regular intervals of time, the hydrogels were taken out of the swelling media, wiped with Whatman paper, and weighed again (Wf). The change in the swelling was noted down. The study was conducted for 48 h.[Citation24]
2.7. Mechanical studies
The developed hydrogels were thoroughly characterized by cyclic compression, repetitive stress relaxation (SR), and repetitive creep studies. The samples for the mechanical studies were molded into cylindrical shape (diameter = 13.7 mm, height = 16.5 mm, stress area = 147.76 mm). A 30 mm flat probe was used for conducting the studies. All the studies were conducted for 10 cycles at a speed of 1 mm/s.[Citation25]
2.8. Electrical studies
The electrical properties of the developed hydrogels were studied using an in-house built I–V analyzer and an impedance analyzer. The hydrogels were made in cylindrical molds. Gold-plated copper electrodes were used for the electrical characterization of the hydrogels.[Citation26]
2.9. Thermal studies
The thermal measurements were done using DSC 200 F3 Maia (Netzsch Instruments Limited, Germany) instrument. The thermal properties associated with the different thermal events were determined by heating the samples and subsequently cooling the samples in the heating range of 20–200 °C. The rate of heating or cooling was 5 °C/min. The experiments were conducted under inert nitrogen environment.
2.10. In vitro cytocompatibility studies
The in vitro cytocompatibility of the samples were analyzed using HaCat (Human Keratinocytes) cells by leachate extraction method. In brief, the samples were powdered in a mortar and pestle after freezing in liquid nitrogen. One gram of the powdered samples was immersed in 10 ml of PBS (pH 7.2) at 37 °C and incubated in a shaker incubator for 24 h. The extracts were filtered using a microfilter (0.22 μm). Ten microliter of the filtered extract was added to a 96 well plate previously seeded with 1 × 104 cells/well and subsequently incubated for 24 h in a CO2 incubator. Dulbecco’s Modified Eagle Medium – 10% Fetal Bovine Serum was used as the cell culture medium. The cell viability was calculated using MTT assay method. All the assays were done in triplicates.
3. Results and discussion
3.1. Preparation of the hydrogels
The hydrogels, containing various proportions of MC, were prepared by free radical polymerization of HEMA. The free radical polymerization was initiated using APS-TEMED redox pair initiator. The percentage monomer conversion of HM1 was found to be 97 ± 2%. No attempts were made to cross-link MC. These types of hydrogels are often regarded as semi-IPN polymeric hydrogels.[Citation27] All the hydrogels were white in color. The white color of the pHEMA hydrogels have been attributed to the heterogeneous precipitation of pHEMA during bulk polymerization. HEMA is a water-soluble monomer but during bulk polymerization there is an increase in the hydrophobicity of the polymer, which in turn, results in the change in the solubility parameter. Usually pHEMA gels are transparent in nature if they are prepared in an aqueous environment containing lower proportion of water. Increase in the water content above 45% has been reported to induce phase separation of the polymer (a heterogenous phenomenon). This makes the hydrogel to appear as milky white opaque polymeric constructs.[Citation28] The whiteness of the hydrogels increased with the increase in the MC content. The apparent firmness of the hydrogels was lower when the MC content was higher. There was a corresponding increase in the stickiness of the hydrogels when the proportion of MC was increased. The pictographs of the hydrogels have been shown in the Figure . The hydrogels were soothing to touch and did not have any odor.
3.2. Degree of polymerization
Flory–Rehner theory is based on the equilibrium swelling theory that allows the determination of the degree of polymerization based on the swelling limits. This theory is based on the principle that a polymer will absorb its neighboring solvent until the solvent chemical potentials of the polymer are equal both inside and outside. Table represents the key parameters that have been used to solve the equation. Average molecular weight (Mc) after cross-linking was found to be 14.07 ± 0.32 g/mol and degree of polymerization (X) was 9.28 ± 0.16.
Table 2. Parameters used for the determination of the average molecular weight between polymer cross-links and the cross-linking density.
The Flory parameter () is based on the polymer–solvent interaction and is a function of both temperature and concentrations.
is often determined empirically. In hydrogel system, such as pHEMA, where the hydrogen bonding is significant,[Citation29,30] this empirically calculated
value becomes inaccurate and the calculation of degree of polymerization stands only as an approximation.
3.3. Microscopic studies
The microstructure of HM1 showed presence of interlocked globular structures (Figure ). Similar kind of observation has been reported by Das et al. while synthesizing acrylate-based hydrogels.[Citation31] Such kind of structural arrangement may be explained by the presence of hydrophobic backbone of pHEMA and hydrophilic hydroxyl groups. This imparts an ampiphilic character to pHEMA. Hence, during the preparation of the hydrogels, the hydrophobic domains try to form spherical droplets, such that, the hydrophobic domains form the core of the droplets. The hydrophilic hydroxyl groups appear onto the surface of these droplets. The fluorescent micrograph of the hydrogels (Supplementary material, Figure S1) suggested a hydrophobic nature of the spherical droplets. Incorporation of MC into the pHEMA matrices showed relatively distant globular particles in HM2, HM3, and HM4, respectively. A further increase in the MC content in HM5 resulted in the formation of a bicontinuous biphasic formulation. The bicontinuous formulations are categorized by the presence of both the phases as continuum phase.
3.4. XRD studies
The XRD profiles of the hydrogels were found to be similar (Figure (a)). A major broad peak at 19° 2θ followed by two broad peaks at nearly 31° and 43° 2θ, respectively, was observed in all the hydrogels. Apart from these prominent peaks, a shoulder peak at ~12° 2θ was also observed. Absence of any sharp peaks in the profile was an indicative of predominant amorphous nature of the hydrogels. The full width at half maximum (FWHM) of the peak at nearly 19° 2θ was calculated to have an understanding about the variation in the crystallinity of the hydrogel matrices. The FWHM of the hydrogels was in the order of HM1 ≈ HM3 > HM4 > HM2 > HM5. FWHM has been reported to be inversely related to the crystallinity of the formulations.[32] The results suggested that the incorporation of MC into the pHEMA matrix resulted in the increased crystallinity of the HM2. A further increase in the MC content resulted in the increase in the amorphosity of HM3. Thereafter, an increase in the MC content resulted in the subsequent increase in the crystallinity of the hydrogels.
3.5. FTIR spectroscopic studies
FTIR spectra of the raw materials and the hydrogels have been shown in Figure (b). MC showed a small hump-like structure at ~2900/cm because of CH stretching, associated with the ring methane hydrogen atoms.[Citation33] The FTIR spectrum of MC showed peaks at ~1100 and 1040/cm, corresponding to CO stretching vibrations in the polysaccharide skeleton.[Citation34] FTIR spectrum of HEMA showed characteristic peaks of stretching vibrations of OH, CH, and CO at ~3500, ~3000, and ~1735 cm−1, respectively.[Citation35] These peaks were conserved in the hydrogels, but their intensity was altered. This indicated that there was a change in the microenvironment of the functional groups. The result can be correlated with the XRD studies, which indicated changes in the crystallinity of the hydrogels as the composition was varied. The OH absorption bond was found to be broadened in the formulations. This may be because of the strong hydrogen bonding between pHEMA and MC.[Citation36]
3.6. Swelling studies
The swelling properties of the hydrogels were tested at three different temperatures using buffers of either pH 1.2, 7, or 9 (Figure ). An increase in the MC content resulted in the increase in the swelling capability of the hydrogels. This trend was observed under all the temperatures. The degree of swelling of the hydrogels at different pHs (varying the temperature) suggested that the degree of swelling was lower at pH 1.2. The degree of swelling increased when the pH of the swelling media was increased to 7. A further increase in the pH to 9 resulted in a corresponding decrease in the percentage swelling. This observation may be explained by the swelling/dissolution property of MC. The cellulose derivatives are usually soluble at higher pHs. At pH 1.2, the absorption of the buffer was lower since MC is poorly hydrated at lower pHs. An increase in the pH to 7 resulted in the increased hydrophilicity of MC, which in turn, resulted in the increase in the swelling of the hydrogels. A further increase in the pH to 9 resulted in the quick hydration and dissolution of the MC. This resulted in the diffusion of the MC out of the hydrogel matrix, thereby causing a decrease in the percentage swelling because of the elastic reconciliation of the pHEMA matrix towards the core.
As the temperature of the swelling media was increased from 4 to 37 °C, a corresponding decrease in the percentage swelling of the hydrogels was observed. This observation may be related to the temperature-sensitive property of MC. The aqueous solutions of MC undergo an increase in the viscosity as the temperature is increased,[Citation37] which may result in the formation of a gelled structure at 37 °C. The formation of the reversible gelled structure at higher temperatures resulted in the shrinkage of the MC phase with a corresponding decrease in the hydrophilicity. The reduction in the hydrophilicity of MC may explain the decrease in the percentage swelling of the hydrogels at higher temperatures.
3.7. Mechanical testing
The firmness of the hydrogels was predicted from the SR study by determining D20 values (Figure (a)). This parameter is used often in food industries to estimate the firmness of the semi-solid food products.[Citation38] D20 value is defined as the distance moved by the probe to attain a 20 g force. Longer the distance moved by the probe to attain a said force indicates softer nature of the hydrogels. In the present case, highest D20 value was observed in HM1 followed by HM4, HM5, HM3, and HM2, respectively. This suggested that the firmness of the pHEMA hydrogel (HM1) was lowest. As the concentration of the MC was increased in HM2–HM4, there was a corresponding increase in the D20 value. A subsequent increase in the MC content in HM5 resulted in the decrease in the D20 value. The observation suggested that an increase in the MC content resulted in the decrease in the firmness of the hydrogels with the exception of HM5. This exception may be explained by the change in the microstructural arrangement of the pHEMA and MC phases. The hydrogels showed a decrease in the D20 value when the hydrogels were subjected to cyclic SR studies. This may be explained by the bulk work hardening of the hydrogels when repetitive SR study was performed.
Figure 5. Mechanical properties of the hydrogels. (a) D20, (b) Cyclic SR, Cyclic creep study of: (c) HM1, (d) HM2, (e) HM3, (f) HM4, (g) HM5, and (h) Creep recovery.
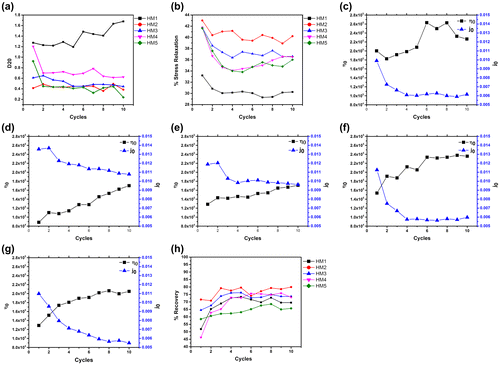
SR study of the hydrogels was calculated for 10 cycles. The results have been shown in Figure (b). The percent stress relaxation (%SR) of HM1 was found to be lowest. The increase in the MC content resulted in the corresponding decrease in the %SR values. %SR of the hydrogels showed an initial decrease followed by a base stationary phase as the hydrogels were subjected to repetitive SR studies. The results indicated an increase in the elastic component of the hydrogels when subjected to repetitive SR studies. %SR is a measure of viscoelastic property of the material. A %SR of 100% suggests fluid nature of the formulation, whereas, a %SR of 0% is an indicator of an elastic material. The hydrogels showed percent SR in between 0 and 100%. This is an indicative of viscoelastic nature of the prepared hydrogels. Amongst all the hydrogels, HM1 showed highest elastic nature, that is, the %SR was lowest in HM1. In general, addition of MC resulted in the increase in the fluid nature of the hydrogels. Amongst the MC containing hydrogels, the %SR was found to be in the order of HM2 > HM3 > HM4 ≈ HM5. This indicated a decrease in the elastic nature with the increase in the MC content.
Cyclic creep studies were conducted to have an understanding about the variation in the long-term viscoelastic property (creep compliance, creep indentation, and percent recovery) of the hydrogels (Figure (c–h)).[Citation39] The viscosity of HM1 was highest amongst all the hydrogels. Incorporation of MC into the hydrogel structure resulted in the decrease in the viscosity in HM2. An increase in the MC concentration (HM2–HM4) resulted in the increase in the viscous component of the hydrogels. Thereafter, a further increase in MC content resulted in a corresponding decrease in the initial viscous component. The initial viscosity was in the order of HM1 > HM4 > HM3 ≈ HM5 > HM2. On the contrary, an increase in the MC content resulted in the decrease in the creep compliance. The initial creep compliance value was in the order of HM2 > HM3 > HM4 > HM5 > HM1. Creep compliance provides information about the ability of a material to undergo immediate recovery to its original shape when an applied stress is removed from the formulations.[Citation39] A formulation having a higher elastic component usually has a lower viscous component. The viscous and the creep compliance values of the prepared hydrogels were in support of each other. Further, when the hydrogels were subjected to repetitive creep cycles, an increase in the viscosity with a corresponding decrease in the creep compliance was observed. The recovery of the hydrogels from the deformation, when the applied stress is withdrawn, provides information about the elastic component.[Citation39] If a material is elastic in nature, a 100% recovery is a usual phenomenon. On the contrary, a partial recovery of the deformation of the formulations was observed. This is because of the loss of the viscous component during the creep phase. The hydrogels, in general, showed an increase in the percent recovery during the initial cycles which attained a plateau phase during the later phase of the cycles. This indicated a decrease in the viscous component with a corresponding increase in the elastic component as the hydrogels were subjected to repetitive creep studies. Further, during the later phase of the creep cycles, a constant recovery was seen. This indicated complete loss of the viscous component. The recovery results are in support of the results obtained from the creep studies (viscosity and creep compliance). The percent recovery at the end of the 10th cycle (final cycle) was in the order of HM2 > HM3 ≈ HM4 > HM1 > HM5.
3.8. Conductivity studies
I–V characteristics of the hydrogels were determined using a sinusoidal signal of 10 kHz (Figure ). For the analysis, a variable current (Irms = 10–300 mA) was passed through the hydrogels and the corresponding voltage drop across the hydrogel samples was measured. A sinusoidal signal was used for the analysis because the I–V characteristics measured using DC current are affected with the polarization of the electrode–sample interface.[Citation26] This results in the erroneous measurement of the I–V characteristics. To avoid this phenomenon, a conscious attempt was made to record the I–V characteristics at 10 kHz. The hydrogels showed a linear I–V profile. This is an indicative of the resistive dominant behavior of the hydrogels. The slope of the I–V profile of the hydrogels provides information about the resistive component of the hydrogels. The slope of the hydrogels was in the order of HM2 >> HM3 > HM4 > HM1 > HM5. The results indicate that the incorporation of MC into the hydrogel matrix resulted in the increase in the resistive component of HM2. A further increase in the MC content resulted in the subsequent decrease in the resistive component of the hydrogels. This result may be explained by the changes in the microarchitecture of the hydrogels as the MC content was increased. An increase in the MC content showed an increase in the size of the globular dispersed droplets, which formed biphasic architecture at the highest MC concentration. An increase in the droplet size resulted in the reduction in the grain boundary resistive effect which could be manifested from the I–V profiles of the hydrogels.
The conductivity of the hydrogels was measured in the frequency range of 200 Hz–15 kHz. Incorporation of MC into the pHEMA matrix resulted in the marked decrease in the conductivity of the hydrogel. Subsequent increase in the MC content resulted in the increase in the conductivity. The conductivity profile of the hydrogels suggested capacitive dominant nature, characterized by lower conductivity at lower frequencies and a higher conductivity at higher frequencies. But the I–V characteristics of the hydrogels showed a linear profile indicating a resistive dominant character of the hydrogels. From the above observations, it may be concluded that the capacitive dominant nature, as observed from the conductivity profile, was mainly because of the electrode–sample interface polarization.[Citation26]
3.9. Thermal studies
HM1, HM3, and HM5 were selected as the representative samples for the thermal analysis (Figure ). All the hydrogels showed a broad endothermic peak, with a central peak position of nearly 90 °C. The broad endothermic peak during the heating cycle was because of the evaporation of the moisture content present in the hydrogel matrices. Increase in the MC concentration resulted in the shifting of the peak position of the endothermic peak towards the higher temperature. The change in the enthalpy and the entropy was calculated during the evaporation of the moisture. The result suggested that the change in the enthalpy and the entropy was highest in HM1 (control). Incorporation of MC resulted in decrease in these values. The results suggested that the moisture retention capacity of hydrogels was higher when the concentration of the MC was lower. The cooling endotherm did not show presence of any peaks.
3.10. In vitro cytocompatibility studies
The in vitro cytocompatibility of the hydrogels was analyzed using HaCat cells (Figure ). Since both pHEMA and MC are well-known biocompatible material, the cell viability index for all the samples was found to be within acceptable biocompatible range. The results showed that HM2 and HM3 had better cell viability index than the control (HM1), whereas HM4 and HM5 showed slightly lower cell viability index. In gist, incorporation of MC in lower proportions improved the cell viability. Subsequent increase in the MC content resulted in the decrease in the relative cell viability. Another interesting observation is that few cells turned into spheroids in all the samples (Supplementary material, Figure S2). Thirumala et al. (2013) also reported similar observations using adipose tissue derived stem cells in the presence of MC.[Citation40] This may explain the reduced cell viability index with the increase in the MC concentration.
4. Conclusion
The current study reports the successful development of novel dual environment sensitive acrylate-based hydrogels. The hydrogels were prepared as semi-IPN networks of pHEMA and MC. The microstructure of the hydrogels showed globular structures of pHEMA which formed self-assembled interlocked structures of three-dimensional polymer network. Incorporation of the MC resulted in the increase in the interglobular distance of the pHEMA globules. When the MC concentration was highest, the globular structures were completely eradicated. Instead, a bicontinuous structure was observed. Hence, it was expected that the properties of HM5 would vary from the normal trend of the properties of the other MC-containing hydrogels. The mechanical studies suggested viscoelastic nature of the hydrogels. When the hydrogels were subjected to repetitive mechanical stress, it was observed that the viscous component of the hydrogels was lost quickly. The thermal studies suggested improved moisture retention capacity of the MC containing hydrogels. The electrical properties of the hydrogels suggested resistive dominant nature of the hydrogels.
Conflict of interest
There was no conflict of interest among the authors.
Supplemental data
Supplemental data for this article can be accessed here. [http://dx.doi.org/10.1080/15685551.2015.1012626]
Acknowledgment
The authors are thankful to MHRD, Govt. of India for providing research facility and National Institute of Technology Rourkela for the logistic support.
References
- Hoffman AS. Hydrogels for biomedical applications. Adv. Drug Delivery Rev. 2002;54:3–12.10.1016/S0169-409X(01)00239-3
- Wen X, Wang T, Wang Z, Li L, Zhao C. Preparation of konjac glucomannan hydrogels as DNA-controlled release matrix. Int. J. Biol. Macromol. 2008;42:256–263.10.1016/j.ijbiomac.2007.11.006
- Kobayashi M. Development of the shields for tendon injury repair using polyvinyl alcohol? hydrogel (PVA-H). J. Biomed. Mater. Res. 2001;58:344–351.10.1002/(ISSN)1097-4636
- Stammen JA, Williams S, Ku DN, Guldberg RE. Mechanical properties of a novel PVA hydrogel in shear and unconfined compression. Biomaterials. 2001;22:799–806.10.1016/S0142-9612(00)00242-8
- Wichterle OL. Hydrophilic gels for biological use. Nature. 1960;185:117–118.10.1038/185117a0
- Trieu H, Qutubuddin S. Poly(vinyl alcohol) hydrogels: 2. Effects of processing parameters on structure and properties. Polymer. 1995;36:2531–2539.10.1016/0032-3861(95)91198-G
- Maddox YT, Bernstein HN. An evaluation of the bionite hydrophilic contact lens for use in a drug delivery system. Ann Ophthalmol. 1972;4:789–790.
- Santin M, Huang SJ, Iannace S, Ambrosio L, Nicolais L, Peluso G. Synthesis and characterization of a new interpenetrated poly(2-hydroxyethylmethacrylate)—gelatin composite polymer. Biomaterials. 1996;17:1459–1467.10.1016/0142-9612(96)89769-9
- Das D, Das R, Ghosh P, Dhara S, Panda AB, Pal S. Dextrin cross linked with poly(HEMA): a novel hydrogel for colon specific delivery of ornidazole. RSC Adv. 2013;3:25340–25350.
- Hoi M, KS, Korbelie Jqb P, Adam M. Poly(HEMA)-collagen composite as a biomaterial for hard tissue replacement. Clin. Mater. 1993;13:19–20.
- Peppas NA, Kelley BK, Madeline T-L, Anthony ML. Poly(ethylene glycol)-containing hydrogels in drug delivery. J. Controlled Release. 1999;62:81–87.
- Uhlich T, Ulbricht M, Tomaschewski G. Immobilization of enzymes in photochemically cross-linked polyvinyl alcohol. Enzyme Microb. Technol. 1996;19:124–131.10.1016/0141-0229(95)00190-5
- Matsumoto A, Ikeda S, Harada A, Kataoka K. Glucose-responsive polymer bearing a novel phenylborate derivative as a glucose-sensing moiety operating at physiological pH conditions. Biomacromolecules. 2003;4:1410–1416.
- Ichikawa H, Fukumori Y. A novel positively thermosensitive controlled-release microcapsule with membrane of nano-sized poly(N-isopropylacrylamide) gel dispersed in ethylcellulose matrix. J. Controlled Release. 2000;63:107–119.10.1016/S0168-3659(99)00181-9
- Liang H-F, Hong M-H, Ho R-M, Chung C-K, Lin Y-H, Chen C-H, Sung H-W. Novel method using a temperature-sensitive polymer (methylcellulose) to thermally gel aqueous alginate as a pH-sensitive hydrogel. Biomacromolecules. 2004;5:1917–1925.
- Knill CJ, Kennedy JF. Degradation of cellulose under alkaline conditions. Carbohydr. Polym. 2003;51:281–300.10.1016/S0144-8617(02)00183-2
- Verestiuc L, Ivanov C, Barbu E, Tsibouklis J. Dual-stimuli-responsive hydrogels based on poly(N-isopropylacrylamide)/chitosan semi-interpenetrating networks. Int. J. Pharm. 2004;269:185–194.
- Aguilar M, Elvira C, Gallardo A, Vázquez B, Román JS. Smart polymers and their applications as biomaterials. Top. Tissue Eng. 2007;3:1–27.
- Liu B, Hu J. The application of temperature-sensitive hydrogels to textiles: a review of Chinese and Japanese investigations. Fibres Text. Eastern Eur. 2005;13:45–49.
- Jaiswal MK, Pradhan A, Banerjee R, Bahadur D. Dual pH and temperature stimuli-responsive magnetic nanohydrogels for thermo-chemotherapy. Int. J. Nanosci. Nanotechnol. 2014;14:4082–4089.10.1166/jnn.2014.8662
- Comyn J. Polymer science dictionary. 2nd ed. London: Mark Alger Chapman & Hall/Elsevier. ISBN 0 412 60870 7.
- Thakur G, Muhammad AN, Dérick R, Kunal P, Analava M, Amit B. Gelatin-based emulsion gels for diffusion-controlled release applications. J. Biomater. Sci., Polym. Ed. 2012;23:645–661.10.1163/092050611X555830
- Peppas N, Bures P, Leobandung W, Ichikaw H. Hydrogels in pharmaceutical formulations. Eur. J. Pharm. Biopharm. 2000;50:27–46.10.1016/S0939-6411(00)00090-4
- Mallick S, Sai SS, Beauty B, Kunal P, Sirsendu SR. Gelatin-based emulsion hydrogels as a matrix for controlled delivery system. Mater. Manuf. Processes. 2012;27:1221–1228.10.1080/10426914.2012.663133
- Behera B, Sagiri SS, Singh VK, Pal K, Anis A. Mechanical properties and delivery of drug/probiotics from starch and non-starch based novel bigels: a comparative study. Starch-Stärke. 2014;66:865–879.10.1002/(ISSN)1521-379X
- Singh VK, Sagiri SS, Singh VK, Pal K, Anis A. Molecular and electrochemical impedance spectroscopic characterization of the carbopol based bigel and its application in iontophoretic delivery of antimicrobials. Int. J. Electrochem. Sci. 2014;9:5049–5060.
- Zhang J-T, Bhat R, Jandt KD. Temperature-sensitive PVA/PNIPAAm semi-IPN hydrogels with enhanced responsive properties. Acta Biomater. 2009;5:488–497.10.1016/j.actbio.2008.06.012
- Carone TW. Hydrogels for central nervous system regeneration: surface modulus and microtopographical effects on neuronal cell behavior. Ann Arbor (MI): ProQuest; 2008.
- Brannon-Peppas L, Peppas NA. Time-dependent response of ionic polymer networks to pH and ionic strength changes. Int. J. Pharm. 1991;70:53–57.10.1016/0378-5173(91)90163-I
- Barton AF. CRC handbook of solubility parameters and other cohesion parameters. Boca Raton (FL): CRC Press; 1991.
- Das A, Ghosh S, Ray AR. Unveiling the self-assembly behavior of copolymers of AAc and DMAPMA in situ to form smart hydrogels displaying nanogels-within-macrogel hierarchical morphology. Polymer. 2011;52:3800–3810.10.1016/j.polymer.2011.05.051
- Shah DK, Sai SS, Behera B, Kunal P, Krishna P. Development of olive oil based organogels using sorbitan monopalmitate and sorbitan monostearate: a comparative study. J. Appl. Polym. Sci. 2013;129:793–805.
- Su J-F, Huang Z, Yuan XY, Wang XY, Li M. Structure and properties of carboxymethyl cellulose/soy protein isolate blend edible films crosslinked by Maillard reactions. Carbohydr. Polym. 2010;79:145–153.
- Taleb MFA, El-Mohdy H, El-Rehim HA. Radiation preparation of PVA/CMC copolymers and their application in removal of dyes. J. Hazard. Mater. 2009;168:68–75.10.1016/j.jhazmat.2009.02.001
- Kiremitçi M, Çukurova H, Özkar S. Spectral characterization and thermal behaviour of crosslinked poly(hydroxyethylmethacrylate) beads prepared by suspension polymerization. Polym. Int. 1993;30:357–361.10.1002/(ISSN)1097-0126
- Castillo E, Koenig JL, Anderson JM, Lo J. Protein adsorption on hydrogels. Biomaterials. 1985;6:338–345.
- Haque A, Morris ER. Thermogelation of methylcellulose. Part I: molecular structures and processes. Carbohydr. Polym. 1993;22:161–173.10.1016/0144-8617(93)90137-S
- Engineering, B. [Internet] [cited 2015 Jan 2]; Available from: http://www.brookfieldengineering.com/education/applications/texture-butter-margarine-spreadability.asp.
- Yilmaz MT, Safa K, Mahmut D, Hasan Y, Ahmed K. Characterization of O/W model system meat emulsions using shear creep and creep recovery tests based on mechanical simulation models and their correlation with texture profile analysis (TPA) parameters. Int. J. Food Eng. 2012;108:327–336.
- Thirumala S, Gimble JM, Devireddy RV. Methylcellulose based thermally reversible hydrogel system for tissue engineering applications. Cells. 2013;2:460–475.10.3390/cells2030460