Abstract
Then polystyrene supramolecular networks were prepared by radical copolymerization of styrene with monomers containing donor–acceptor (DA) moieties methacrylamide (MAAM) or 2-methacrylamidopyridine (MAP). The molar percentages of MAAM and MAP in the copolymers were, respectively, 2–20% and 6–40%. The glass transition temperatures (Tgs) of these copolymers have been modeled by the Kwei equation showing secondary interactions due to intermolecular hydrogen bonds. The DA-AD association constants (K) have been calculated by 1H NMR spectroscopy of MAAM and MAP. These constants were, respectively, 6.75 and 16.75 M−1. Dynamic mechanical analyses by frequency and temperature sweep tests, were carried out in the molten state. Styrene–MAP or styrene–MAAM copolymers had higher moduli than neat polystyrene but the MAP influences the materials’ properties distinctively from the MAAM. MAP copolymers had a second G′ plateau that appeared at 150 °C, thus showing the formation of a supramolecular network.
1. Introduction
Supramolecular chemistry is defined as the ‘chemistry of the non-covalent and intermolecular bonds.’ In contrast to the covalent bonds, they have lower binding strength,[Citation1] but endow materials with reversible interactions typically dependent on temperature, solvent, and pH.[Citation2–4] Chemical moieties are able to form host–guest systems in dimers by single or multiple interactions distinguished by homodimers and heterodimers.[Citation5] Different kinds of interactions can be involved in supramolecular chemistry such as hydrogen bonds,[Citation6–8] ionic attractions,[Citation9] amphiphilic interactions,[Citation10] metal coordinations [Citation11], or π–π stackings.[Citation12]
Supramolecular polymers by hydrogen bonding association are extensively used to build polymer backbones by assembling non-covalent moieties [Citation13] or polymer networks through intermolecular interactions between the chains. The dimers can be linked to the backbone or located at the end chains.[Citation14] The total energy difference, ΔEHB, between the hydrogen-bonding system at equilibrium and the total energy of the free components [Citation1,15,16] can reach 120 kJ M−1 with a single hydrogen bond, almost half the value of covalent bond strength such as σ carbon–carbon bond, close to 348 kJ M−1.[Citation17] Although hydrogen bonds are not the strongest non-covalent interactions, they are prominent in supramolecular chemistry due to their versatility and directionality. The determination of association constants K is required to measure the reversible binding strength in supramolecular polymers (Equation (Equation1(1) )).[Citation18,19]
With heterodimer systems specifically, K is obtained by Rose-Drago model which establishes a relationship between δ and the host/guest mol ratio [Citation18] (Equation (Equation1(1) )). It is generally in the range of 10–105 M−1
(1)
where [H]0 and [G]0 are, respectively, the initial concentrations of host and guest; δ is the observed chemical shift of the interacting host/guest; δh is the chemical shift of the free host; δc is the chemical shift of the fully complexed host that is calculated by the equation and K.
Chen and Rosenberger [Citation20], based on the work of Horman and Dreux [Citation21] applied to caffeine, developed a model for homodimer systems (Equation (Equation2(2) )) working with single hydrogen bonds up to quadruple DADA-ADAD pairs.[Citation22,23]
(2)
where δm is the free moiety’s chemical shift; δd is the moiety’s chemical shift that is fully dimerized; δobs is the observed chemical shift; K and C are, respectively, the association constant and the homodimer concentration.
Several works concern systems with multiple hydrogen bonds which greatly enhance the variety of dimers as well as the association constants.[Citation24–27] Polymers with double hydrogen bonds based on amides could be achieved by radical copolymerizations [Citation28–31] or polycondensation.[Citation32] These copolymers have a good water solubility due to the amide groups and the strength of intermolecular hydrogen bonds which have been qualitatively [Citation28] or quantitatively [Citation32] studied by NMR at different temperatures. 2-Aminopyridine derivatives are also other kinds of double hydrogen bonding units. The N–H located in the ortho position of the pyridine ring also gives an array of the DA double hydrogen bonding homodimers. K were calculated from 1 to 100 M−1 [Citation22,32] and were sufficient to increase the storage modulus in the molten state [Citation32] although they are close to single hydrogen bonding K.
There are many specific multiple hydrogen-bond systems. Among them, ureidopyrimidone (UPy) derivatives [Citation24,26,33–35] present four hydrogen bonds with strong associations. UPy can be in equilibrium between its two tautomers, AADD or ADAD patterns,[Citation36] both of which are self-associated in homodimers. These high hydrogen-bonding motifs exhibit high binding constants around 107–108 M−1 [Citation14] which lead to physical networks with high rubbery modulus.[Citation34]
Feldman prepared model networks from polymers bearing Upy moieties.[Citation37] Butyl acrylate and 6-(tert-butoxycarbonylamino)hexyl acrylate were copolymerized by ATRP, with pendant butoxycarbonylamino groups to finally graft onto the Upy moieties. Subramani et al. [Citation38] prepared a triple hydrogen bonding moiety grafted onto a polystyrene backbone. Styrene and p-(chloromethyl) styrene were first copolymerized by radical polymerization with AIBN. The pendant chloromethyl groups were modified to obtain a diaminotriazine group as DAD moiety.
By another approach, a monomer bearing supramolecular moieties was first prepared and then copolymerized with another monomer. McKee et al. [Citation39] reported the synthesis of the UPy methacrylate. This functional monomer was copolymerized with methyl methacrylate or 2-ethylhexyl methacrylate by free radical polymerization. Park and Zimmerman [Citation40] described the supramolecular network based on immiscible poly(butyl-methacrylate) (PBMA) and polystyrene blends. Two functional vinyl monomers, urea of guanosine (UG) with ADDA moiety and 2,7-diamido-1,8-naphthyridine (DAN) with DAAD moiety were prepared by HEMA esterification and a substitution of 4-vinylbenzyl chloride. P(BMA-co-UG) and P(S-co-DAN) were, respectively, copolymerized and then mixed together in order to study the strong supramolecular associations at the interface between the immiscible PS and PBMA. Methacrylate UPy [Citation41] was also studied through a similar method synthesizing P(BMA-co-UPy). Both dimerizations of UPy and its association with DAN-St were investigated.
These studies where strong interactions develop with K between 106 and 108 are based on relatively complicated synthetic methods but such strong interactions are a good opportunity to obtain reversible cross-linked materials with good viscoelastic and mechanical properties.
It is often necessary to improve the mechanical properties of polystyrenic derivative blends by compatibilization. This is the case when these blends are designed for a specific use and also for waste recycling. The addition to PS waste blends of a small part of new PS developing supramolecular interactions will improve the mechanical properties of the blends. In addition, since the used supramolecular interactions are reversible, the compatibilized blends processing can be easily realized and repeated several times if necessary. The aim of this study is to determine if double hydrogen bonding motifs, available commercially or easy to prepare, can reach high enough constant bindings. To answer these questions, DA moieties are chosen among amide and aminopyridine derivatives from, respectively, methacrylamide (MAAM) and 2-methacrylamidopyridine (MAP). These monomers will be comparatively copolymerized with some styrene. Structure-property relationships of these copolymers will be emphatically investigated and presented with spectroscopic analyses by IR and 1H NMR. The influence of DA concentration in the copolymer chain will be analyzed by thermal and viscoelastic studies.
2. Experimental
2.1. Reagents
2-Aminopyridine (AP), methacryloyl chloride (MC), methacrylamide (MAAM), Styrene (St), dimethyl sulfoxide (DMSO), triethylamine (TEA), and 2,2′-Azobis(2-methylpropionitrile) (AIBN) were purchased from Aldrich. 2-Acetamidopyridine (AcAP) was purchased from TCI Europe. Chloroform (CHCl3), anhydrous toluene, tetrahydrofuran (THF), ethanol, and acetone were purchased from CARLO ERBA REAGENTS. Styrene was distilled under vacuum (0.2 mbar) at room temperature and stored at −23 °C before its use. The other reagents were used as received.
2.2. 2-Methacrylamidopyridine (MAP) synthesis: monomer with DA moiety
MAP was synthesized through a method adapted from the work reported by Coskun [Citation30,31]: Methacryloyl chloride (10 g, 0.064 mol) in 5 mL toluene was added dropwise to 2-aminopyridine (5 g, 0.054 mol) and triethylamine (10.91 g, 0.108 mol) in 60 mL toluene in a three-neck flask under argon atmosphere within 30 min at 0–5 °C and magnetically stirred for an additional hour. The reactive system was continuously mixed for 12 h gradually raising the temperature to room temperature (around 20 °C). The precipitated side product was filtered off and abandoned. Filtrate was dried by distillation at room temperature under vacuum (0.2 Mbar) to remove the toluene and the methacryloyl chloride excess. The yielding dark-orange viscous MAP was dried under vacuum at 30 °C for 5 h, with a yield of 40%.
2.3. Copolymerization of P(MAAM-co-St) and P(MAP-co-St)
2.3.1. MAAM and styrene copolymerization in THF
Copolymers with different MAAM feeding ratio from 0 to 60 mol% were prepared by solution copolymerization with styrene. 20 g of the comonomers was added in a three-neck flask with 30 mL of THF. 1 mol% of AIBN was added with total comonomers’ amount in reference. The mixture was refluxed under argon atmosphere for 8 h. Then, the products containing 0, 15 and 30 mol% of MAAM were precipitated with 200 mL of ethanol in beakers, and washed twice with ethanol. For copolymers containing more than 30 mol% of MAAM, the precipitation occurred in an acetone-water mixture with a 1:1 volume ratio. Finally, the copolymers were dried under vacuum at 140 °C for 4 h. All the samples are stable till this temperature since moduli remain constant in time, measured by dynamic rheology and time sweep tests at low strain.
Real MAAM ratios were calculated by NMR. Yields were between 80 and 96%. The determination of the copolymers composition has been calculated by 1H NMR after reprecipitation and drying under vacuum and heating as it was already explained in the synthesis section. The MAAM or MAP content incorporated in the copolymer backbone has been calculated using the methyl resonance of MAAM or MAP comonomer and the aromatic protons resonances of styrene units.
2.3.2. MAP and styrene bulk copolymerization
Copolymers with different MAP feeding ratios from 0 to 20 mol% were prepared by bulk copolymerization with styrene. The MAP-St mixtures were placed in a two-neck flask under argon atmosphere, and 1 mol% AIBN was added with the comonomer amount in reference. The reactive system was heated at 70 °C with a silicone oil bath for 6 h. The obtained copolymers were dried in an oven under vacuum (0.2 Mbar) at 140 °C for 3 h. Yields were between 92 and 98%. The same determination method to calculate the copolymer composition was applied than the one described for the MAAM/styrene copolymerization.
2.4. Characterizations
2.4.1. Nuclear magnetic resonance (NMR) spectroscopy
The 1H NMR analyses of P(MAAM-co-St) were performed with a Brucker Advance II spectrometer operating at 250 MHz and 300 K for 64 scans in DMSO-d6 with TMS as an internal reference.
For binding constants determinations, 1H and 13C NMR analyses were, respectively, performed with a Brucker DRX400 spectrometer operating at 400 and 100.6 MHz with a BBPO + probe. These samples were acquired with 256 scans in CDCl3 at 300 K with TMS. Dilutions from 0.09 to 0.002 mol L−1 were operated between each acquisition.
AcAP CDCl3 solution at 0.03 mol L−1 was also analyzed by 1H NMR spectroscopy at different temperatures with the same spectrometer. Spectra were recorded with the same parameters mentioned above but at 300 and 315 K, respectively, in order to determine K.
2.4.2. Size-exclusion chromatography (SEC)
Number average molecular weight (Mn), weight number average molecular weight (Mw), and polydispersity index (PI) were obtained by size-exclusion chromatography.
For P(MAP-co-St) copolymers, the tests were conducted with equipment consisting of a Waters 515 HPLC pump, a refractive index-detector Waters 2414, a Wyatt viscometer, an auto sampler Waters 717, and three Waters Styrogel columns (HR1, HR2, HR3). 1.2–1.8 mg mL−1 samples were analyzed at room temperature in THF at 1 mL min−1.
For P(MAAM-co-St) copolymers, samples were analyzed with a high temperature GPC (Waters Alliance GPC 2000) equipped with three SHODEX columns, HT803, HT804, and HT805 along with a refractometer coupled to a viscometer as detectors. The eluent was DMSO at 1 mL min−1 and 135 °C. The concentration of each sample was 1–1.4 mg mL−1 in DMSO and dissolution was first achieved by heating at 90 °C for 120 min.
2.4.3. Thermal analysis
Thermal transitions were determined by differential scanning calorimetry (DSC) with a TA Q10 device, by heating and cooling ramps from −50 to 200 °C at 10 °C min−1 under a nitrogen flow. The glass transition temperatures of the copolymers were measured during the second heating ramp. The glass transition temperature was calculated in all cases from a unique observed transition meaning that any phase separation was observed by this technique. The used method consisted to determine the inflexion point on the thermogram.
2.4.4. Dynamic mechanical spectroscopy in the molten state
Rheological measurements were carried out with two different pieces of equipment. Molten state rheology of P(MAP-co-St) copolymers was performed using a strain controlled Anton Paar Physica MCR 301 rotational rheometer, in the linear viscoelastic domain of the materials. Dynamic strains were applied using parallel plate geometry with 25 mm diameter. The gap between the plates was fixed at 2 mm. The temperature ramp was fixed at 3 °C min−1 from 200 °C to the minimum temperature at which samples become slipping and/or the transducer is overloaded. The frequency was fixed at 1 rad s−1 and the strain was fixed at 10%.
Molten state rheology of P(MAAM-co-St) copolymers was performed using a stress controlled SR5000 rheometer, also in the linear viscoelastic domain of the material. Dynamic stress was applied using 25 mm parallel plate geometry. The ramp temperature was 3 °C min−1 between 120 and 300 °C. The frequency was fixed at 1 rad s−1 and the stress was typically chosen as 5000 Pa around 200 °C.
3. Results and discussion
3.1. Dimerization of self-assemblies
Self-assemblies by supramolecular interactions can occur between copolymer chains obtained by radical polymerization of styrene with functionalized comonomers. The number of the DA moieties as pendant groups was adjusted by the molar comonomer ratio.
In this study, two monomers with self-assembling moieties were studied: methacrylamide (MAAM) and methacryloyl aminopyridine (MAP).
The capability of self-homodimerization via hydrogen bonds from amide was already described.[Citation22] The homodimerization occurs with a cyclic conformation between both of the involved primary amide functions as shown in Scheme .
The homodimerization between two MAP units is due to two kinds of hydrogen acceptors, nitrogen atoms from pyridine ring, or ketonic oxygen. Then, they can form two different structures in equilibrium with two hydrogen bonds per homodimer as it is shown in Scheme .
3.2. Calculation of association constants K
K value is the factor reflecting the strength of hydrogen bonds. Higher K values mean that more stable assemblies are produced. It is also in relationship with the material plateau modulus in rheology. Strong hydrogen-bonding interactions influence this plateau.[Citation32] The purpose of K calculation is not only to calculate the binding strength in connection with the strength of the non-covalent network, but also to determine quantitatively whether intermolecular or intramolecular dimers are formed. In this study, K was first calculated with simple model molecules before further complicated characterizations with supramolecular polymers since chains’ entanglements and viscosity may affect the dimerization phenomenon.
2-Acetamidopyridine (AcAP) was chosen as a model molecule because its structure is very similar to the MAP. It is able to form the same dimer associations as shown with Scheme . MAAM is used directly.
As discussed in the introduction, Chen’s equation [Citation20] is applied to the homodimer formation. Since δm, δd, and K are constant, the monomer concentration (C) and δobs are the variable. δm, δd, and most importantly, K, are obtained by fitting the experimental data with this equation.
Different monomer concentrations in CDCl3 were prepared five days before the analyses, and placed at room temperature for stabilization. N–H proton has an upfield while the AcAP concentration decreases (Figure ). This phenomena is generally known. Namely, as the solution concentration in structures such as N–H, O–H containing heteroatom increase, the intensity of the hydrogen bond increases and proton resonance has an downfield in the H NMR. NOESY 1H NMR spectra (Figure ) were exclusively recorded for the lowest 2-AcAP concentration in order to deconvolve the doublet at 8.2 ppm to obtain the accurate chemical shift of N–H proton which is overlapped by a pyridine-ring proton. Curve fitting results of AcAP and MAAM are shown in Figure and K values are listed in Table . δN–H proton has an upfield shift while the AcAP concentration decreases (Figure ). This can also be observed in other self-associated amides.[Citation22,42] The difference of δN–H between intramolecular (folded) and intermolecular (unfolded) hydrogen bonds has been reported, concluding that the resonance of a folded hydrogen bond proton is narrow (approx. 0.2 ppm), while it is large (2–3 ppm) for a non-folded one.[Citation43] The maximum of
δ for both AcAP and MAAM (Table ) exceeds 2 ppm, indicating that intermolecular hydrogen bonds are formed between dimers in chloroform.
Figure 1. 1H NMR chemical shift of amide proton at different AcAP concentrations in CDCl3 and 300 K. From upper to lower: 0.0921, 0.0417 and 0.0088 mol L−1.
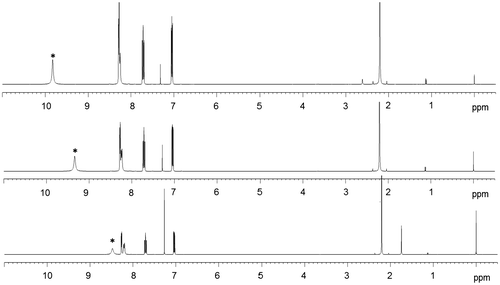
Figure 2. δN–H fitting vs. concentration in CDCl3 at 300 K of (A) AcAP; (B) MAAM. : experimental data and black line: fitted curve.
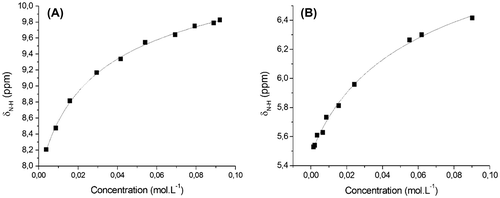
Figure 3. Glass transition temperature (°C) vs. MAAM mass fraction in P(MAAM-co-St) copolymers, ■ experimental point; – fits Tg respecting Fox equation; – fits Tg respecting Kwei equation.
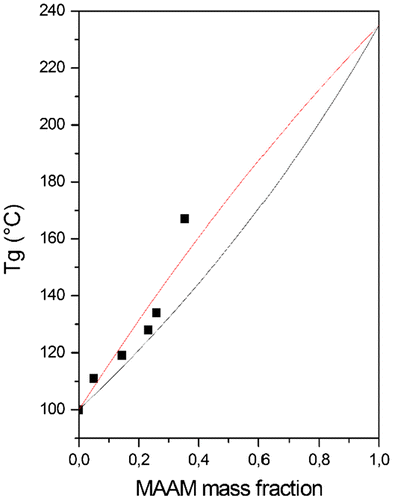
Figure 4. Glass transition (°C) vs. MAP mass fraction in P(MAP-co-St) copolymers, ■ for experimental point; – fits Tg respecting fox equation; – fits Tg respecting Kwei equation.
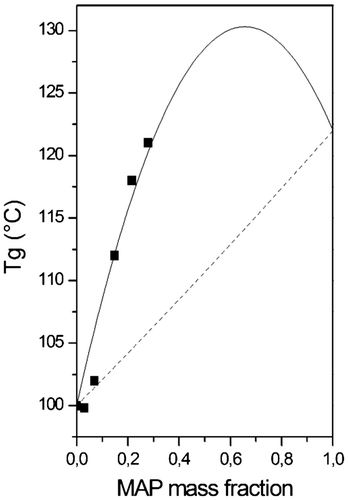
Figure 5. G′ storage modulus (Pa) and G″ loss modulus (Pa) of P(MAAM-co-St) in temperature ramp tests: ramping rate at 3 °C min−1, frequency = 1 rad s−1, strain = 10–0.1%, -●- G′ of 6 mol% MAAM; -○- G″ of 6 mol% MAAM; -▲- 17 mol% G′ of MAAM; -- G″ of 17 mol% MAAM; -■- 27 mol% G′ of MAAM; -□- G″ of 27 mol% MAAM; -◆- 30 mol% G′ of MAAM; -◇- G″ of 20 mol% MAAM; -▼- 40 mol% G′ of MAAM; -▽- G″ of 40 mol% MAAM.
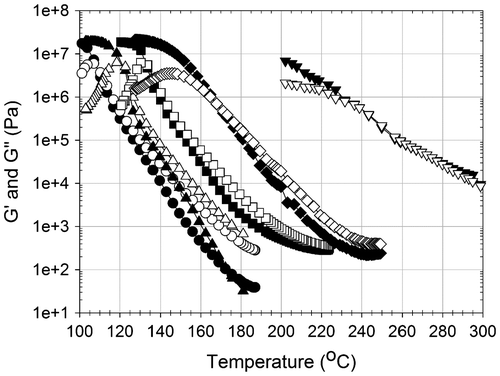
Figure 6. G′ (●) storage modulus (Pa) and G″ (○) loss modulus (Pa) of P(MAAM-co-St) in frequency sweep tests at T = 200 °C strain = 10% for 6, 17 and 27 mol% of MAAM; strain = 1% for 30 mol% of MAAM; strain = 0.1% for 40 mol% of MAAM.
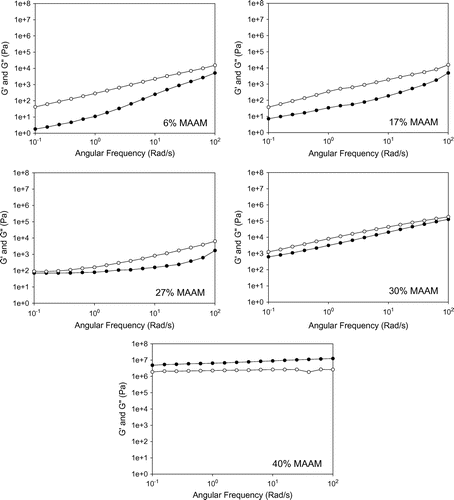
Figure 7. G′ Storage modulus (Pa) and G″ loss modulus (Pa) of P(MAP-co-St) in temperature ramp tests: ramping rate = 3 °C min−1, frequency = 1 rad s−1, strain = 10%, ●: storage modulus G′, ○: loss modulus G″. -●- G′ of 0 mol% MAP; -○- G″ of 0 mol% MAP; -▲- 2 mol% G′ of MAP; -- G″ of 2 mol% MAP; -■- G′ of 10 mol% MAP; -□- G″ of 10 mol% MAP; -◆- G′ of 15 mol% MAP; -◇- G″ of 15 mol% MAP; -▼- G′ of 20 mol% MAP; -▽- G″ of 20 mol% MAP.
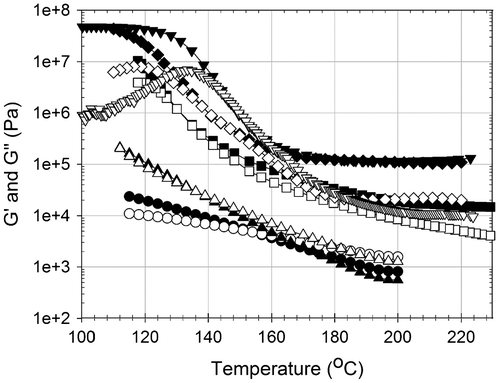
Table 1. K, δm, δd and
δ of monomers derived from curve-fits from Chen Model [Citation20].
K is influenced by the hydrogen-bonding number and array. Calculated KAcAP and KMAAM are based on the simple double hydrogen bonds (DA), so they are certainly weaker than those in typical triple hydrogen bond arrays which are mostly between 65 and 890 M−1.[Citation44] Furthermore, the DA-AD homodimer is also predicted to have lower K than DD-AA homodimer according to Beijer’s theory.[Citation28] But we can conclude that these values are in the suitable range of double hydrogen bonds from 1 to 100 M−1.[Citation22,32,42] In particular, KAcAP (16.75 M−1) is in agreement with existing references according to which the K of acylaminopyridine derivatives varies from 1.5 to 17 M−1,[Citation22] and comparatively higher than other N–HN bonds (0.7–2.1 M−1) in small molecule assemblies.[Citation45] This value is also superior to KMAAM calculated with the value of 6.75 M−1. The difference of the K between AcAP and MAAM may be attributed to the supramolecular moieties’ structures. In AcAP, double hydrogen bonds could be formed based on either N–H
Npyridine or Namide–H
O=C, while MAAM only have one formation based on the amide function. The interaction of N–H
Npyridine is reported as stronger and more stable than amide in solid state [Citation46] that may cause the KAcAP to overwhelm KMAAM with a higher association constant.
These K results from model molecules by NMR dilution experiments prove the dimerization of hydrogen-bonding DA moieties and their corresponding strength. So, when copolymers are synthesized, there exist a high probability to achieve simultaneously the networks’ formation through DA-AD homodimerization before studying them in the next characterizations.
3.3. Solubility of P(MAAM-co-St) and P(MAP-co-St) with various DA moiety concentrations
Formation of a cross-linked material can be determined by solubility tests. Uncross-linked polymers should be soluble in appropriate solvents, while cross-linked polymers can only be swollen. In this study, the copolymers using MAAM or MAP bearing associating moieties are expected to develop hydrogen bonds between the chains making the copolymer insoluble. Solvents of polystyrene were chosen for these tests (THF and CHCl3) and the amounts of MAAM or MAP are the only factors affecting the solubility of the copolymers.
As shown in Schemes and , MAP or MAAM moieties have donor and acceptor neighbors that could self-assemble to DA-AD homodimers. After the copolymerization with styrene, both these DA-AD assemblies behave like crosslinking spots in the polymer networks. The results in Tables and show that with an increasing molar ratio of the DA moiety in copolymers, the solubility changes from soluble to insoluble.
Figure 8. G′ Storage modulus (Pa) and G″ loss modulus (Pa) of P(MAP-co-St) in frequency sweep tests: T = 200 °C, strain = 10%. ●: storage modulus G′, ○: loss modulus G″.
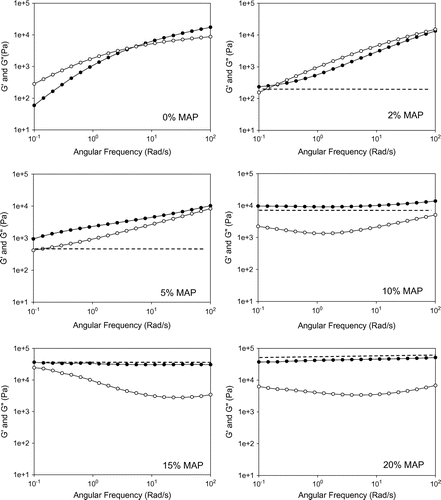
Scheme 1. Synthesis of 2-methacrylamidopyridine (MAP) from 2-aminopyridine and methacryloyl chloride.
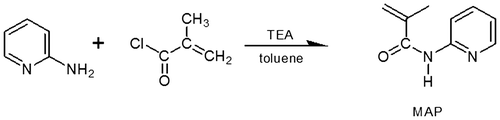
Scheme 2. (a) Self-homodimerization between P(MAAM-co-St) chains and (b) equilibrium self-homodimerization between P(MAP-co-St) chains.
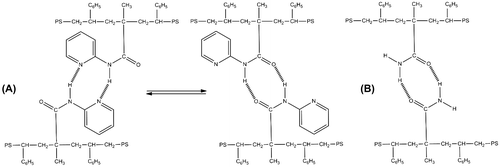
Table 2. Solubility of P(MAAM-co-St) in different solvents for different MAAM/styrene compositions.
Table 3. Solubility of P(MAP-co-St) in different solvents for different MAAM/styrene.
This behavior also depends on the solvents’ polarity. In less polar solvents such as CHCl3 and THF, the assemblies are difficult to dissociate if intermolecular hydrogen bonds are strong and copolymers are insoluble in these solvents. In solvents with high polarity as DMSO, hydrogen bonds’ interactions are weakened, and the solubility of these copolymers is enhanced. For insoluble copolymers, the increase of temperature will improve the solubility, i.e. for P(MAP-co-St). Some of the copolymers, soluble only at high temperature, precipitated again when the temperature dropped down, showing the thermo-responding properties and reversible solubility of these copolymers, which is a feature of supramolecular polymers.
Comparing MAAM and MAP, it is obvious that the latter has stronger interactions than the former in the medium-polar solvents, which is in agreement with K results. Copolymers with a MAP content superior to 5 mol% are insoluble in CHCl3 while in P(MAAM-co-St), this threshold is 17%.
Solubility tests of P(MAAM-co-St) and P(MAP-co-St) confirmed that the polymer networks were formed and controlled by adjusting the molar percentage of DA moiety in the copolymers. The networks based on MAP are stronger than those based on MAAM. They could only be broken at very high temperatures in a highly polar solvent such as DMSO. The threshold of P(MAP-co-St) which begins to show insolubility in THF is also lower as 5%, while in P(MAAM-co-St) it is 27%.
3.4. Thermal properties of P(MAAM-co-St) and P(MAP-co-St)
Both P(MAP-co-St) and P(MAAM-co-St) copolymers show only one glass transition temperature. Tg obtained from both series cannot be fitted to Fox law (Figures and ). They are generally higher than predicted values from Fox equation because secondary interactions exist and restrict the chain motions and relaxations. In this case, Kwei equation (Equation (Equation3(3) )) can represent experimental data.[Citation29,47,48]
(3)
where Tg1 and Tg2 are the glass transition temperatures of the co-monomers and W1 and W2 their weight fractions. K and q are constants. Q value represents a measure of the interaction between both components. For K = 1, q = 0, a simple additive rule is obtained. In this case, Tg evolves linearly with monomer fraction, indicating that no specific interactions are involved in this copolymer system.
Differences of Tgs obtained from Fox equation and experimental values for P(MAAM-co-St) (in Figure ) are not obvious. Curve fitting results in Figure give K = 1, q = 27.47. This high value represents the extent of secondary interaction between polymer chains and the existence of intermolecular hydrogen bonds.[Citation47]
Tgs of P(MAP-co-St) perform a more distinct change depending on MAP mole fraction in Figure . They are relatively close to the value calculated by Fox equation when MAP mole fraction is below 5%. For these samples, hydrogen bonding moieties are not sufficient for the association between the polymer chains. When MAP mole fraction is increased to 10, 15, or even 20%, the differences of Tg between that predicted by Fox equation and the experimental data become more important. These differences are directly in relation with the MAP self-dimerization that increases the strengthening. Calculated constant q from curve-fitting for P(MAP-co-St) is 70.30, which is much higher than P(MAAM-co-St). Consequently, the dimerization between the DA moieties in the P(MAP-co-St) is much stronger than that in P(MAAM-co-St). Relative ratio of qMAP-St and qMAAM-St is in accordance with KMAP-St and KMAAM-St calculated by NMR spectroscopy.
Thanks to this interesting result, it can be seen that although neither Tgs obtained from P(MAAM-co-St) nor P(MAP-co-St) could obey the traditional Fox equation, their fitting with Kwei equation proves the existence of secondary interactions. The Q value derived from Kwei equations could be used as a measure of intermolecular interactions qualitatively.
3.5. Structure analyses by dynamic rheology in the molten state
Dynamic mechanical analyses (DMA) of P(MAAM-co-St) and P(MAP-co-St) copolymers are reported in this part. Since the differences of Tg have already been discussed above, only the viscoelasticity above Tg was analyzed here. The results of P(MAAM-co-St) and P(MAP-co-St) are discussed separately since they are quite different.
3.5.1. P(MAAM-co-St) copolymers dynamic rheology
3.5.1.1. Temperature ramps
Storage (G′) and loss moduli (G″) evolutions in function of the temperature of P(MAAM-co-St) are shown in Figure . Temperature ramp tests were subsequently performed for these copolymers in order to observe the full transition zones above the glass transition temperature of the polystyrene chains.
The crossover temperature at which G″ overpasses G′, corresponds to the joint effect of the glass transition and the hydrogen bond interactions. It is obvious in the temperature-moduli graph that the addition of MAAM has a considerable effect shifting G′ and G″ curves, as well as the corresponding Tcrossover, to high temperatures in Figure .
First, it is observed that a plateau is always observed at 107 Pa for the storage modulus. This plateau is located lower than the glassy plateau of PS since an amorphous polymer in the solid state below its glass transition temperature is expected near 109 Pa. The temperature range of this plateau is clearly dependent of the MAAM content that not influence the value modulus With 30 mol% of MAAM, this plateau holds 140 °C while 40 mol% of MAAM shifts this plateau to 200 °C. It cannot be simply attributed to the hydrogen bonds by the MAAM content since the temperature to break these interactions is not expected as variable with the MAAM content. It is certainly a composition effect due to the MAAM comonomer content in the copolymer chain, since it cannot be neglected when it reaches 40 mol%. This composition effect affects the glass transition temperature finally observed here, always above 100 °C, by DMA. It is in accordance with the Tg determined by DSC (Table ) with a typical shift observed between both the techniques. It is therefore noticed that the chosen range of MAAM content in these synthesis, higher than 17 mol%, shifts the glassy plateau of a pure PS to around two decades lower that finally gives a rubbery-like behavior below Tg by introducing the MAAM comonomer in the PS chains. The MAAM content doesn’t influence the glassy plateau modulus which remains constant at 107 Pa. After a modulus decrease is observed around the glass transition temperature to reach a second plateau attributed to hydrogen bond interactions which appear in copolymers containing over 17 mol% of MAAM. It shows that a part of DA associations still exists at high temperatures. Although the plateau moduli are low (103 Pa) in 27 and 30 mol% of MAAM, copolymers are in a viscous state and thermoplastics under high shear. With a further increment of MAAM content to 40%, the plateau modulus is increased to 105 Pa and the copolymer is in a rubbery state suggesting a high possibility of network formation. The MAAM content is expected to shift this last rubbery plateau at higher values of modulus since the comonomer content directly affects the density of physical crosslinking. This new plateau is very large in temperature to affirm that the thermal reversibility is obtained at reasonable temperature but the modulus, less 103 Pa and less than 30 mol% of MAAM, are low enough to expect the processing in the molten state of these materials by extrusion where the high shear is helpful.
3.5.1.2. Frequency sweep
Storage (G′) and loss (G″) moduli evolutions with angular frequency of P(MAAM-co-St) are shown in Figure . They were measured at least 30 °C above Tg. Since Tg in P(MAAM-co-St) varies from 100 to 167 °C, the testing temperature was set at 200 °C to ensure that all the thermoplastic samples suited the condition of molten state rheological analyses. To interpret these frequency sweep tests, it is interesting to correlate them with the corresponding sample presented on the temperature sweep test at 200 °C (Figure ). Before interpreting a material frequency sweep test for a given content of MAAM on Figure , it is necessary to first remind its physical state at 200 °C by the temperature sweep test on Figure . Then, it is not surprising that copolymers with MAAM contents inferior to 30 mol% have parallel descending G′ and G″ curves from high to low frequency. Storage moduli are low (100–104 Pa) and are depending on the frequency indicating that these materials are in the viscous states without a network formation as observed on Figure . The interaction of DA moieties in MAAM is weak, and the mobility of polymer chains is barely restricted at the tested temperature. When the MAAM content in the copolymer increases (40 mol%), a considerable increase of moduli is obtained when comparing 30% MAAM to 27 mol% MAAM. Furthermore, a plateau on the storage modulus appears and G′ becomes higher than G″ and almost independent from the frequency. Intermolecular interactions in this sample are denser, and highly concentrated, which limits polymer chain movements in a small range, indicating the formation of networks with rubbery-like performances. These complementary analyses complete and confirm the interpretations presented by temperature sweep test.
3.5.2. P(MAP -co-St) copolymers dynamic rheology
3.5.2.1. Temperature ramps
Temperature dependent G′ and G″ of P(MAP-co-St) are shown in Figure . Two important points need to be noticed with temperatures ranging from 110 to 200 °C. First, the increase of MAP content has a direct effect on the storage moduli increase although it is less obvious for copolymers with low MAP content (2–5 mol%). Comparatively to pure PS, an extend of the glassy plateau is observed above 100 °C as it was observed by thermal analysis (Figure ). Similar to MAAM comonomer, MAP content acts directly to the temperature range of this plateau by a composition effect. This plateau is stable at 3.107 Pa, a little higher than the one observed with MAAM but the range MAP content is greatly lower here. The further increase of MAP content, or more specifically the increase of DA hydrogen bond moieties from 5 to 20%, will lead to the same second important observation: higher concentrations of DA-AD hydrogen bonding dimer block the chain movements and create a G′ plateau at high temperatures corresponding to a rubbery plateau due to the formation of the physical network. This plateau cannot be observed in the pure PS at the tested temperature range, whereas it appears in the copolymers with high MAP content (10–20 mol%) between 170 and 200 °C. This is unprecedented because although a few works [Citation14,32,34] present the introduction of supramolecular interaction into polymer materials which transform materials from a viscous liquid to a rubbery-like state with high modulus around 105 Pa, they are normally limited at comparatively low temperature (30–120 °C).
The selective appearance of a rubbery plateau in P(MAP-co-St) with MAP content higher than 5% MAP confirmed that the polymer network could be controlled by adjusting the molar ratio of DA moiety in the copolymers. The interaction, between MAP moieties by homodimerization, is strong and present in the copolymers even at high temperature. This rubbery plateau appears with lower contents than MAAM comonomer that is not suprising since the association constant is stronger with MAP, requiring lower contents of comonomer to reach the same equilibrium, consequently the same crosslinking density.
3.5.2.2. Frequency sweep
Kn results showed that the association in P(MAP-co-St) was stronger than that in P(MAAM-co-St); thus, a more distinctive influence of MAP on both the copolymers’ structure and viscoelasticity is expected. Figure shows the frequency-dependent G′ and G″ curves of various samples having different MAP contents at 200 °C. By the temperature ramp on Figure , 2 and 5 mol% of MAP show that the rubbery state is just reached at 200 °C with a low storage modulus. From 10 mol% of MAP, a clear rubbery plateau is already obtained with a high relative storage modulus above 104 Pa. These observations have to be retrieved by frequency dynamic tests.
Obviously, the evolutions of G′ moduli with MAP content are more noticeable than MAAM. Even a small amount of MAP influences both storage and loss moduli; addition of an auxiliary line (dash line in Figure ) helps to illustrate the MAP’s effect. For the copolymer with 2 mol% MAP, the appearance of a plateau around 5 × 102 Pa, only at low frequency, is due to the joint influence of MAP impact and neat PS performance. This concentration of MAP is not so high that the slope coefficient of G′ curve approaches to neat PS at high frequency. No network is formed in these two samples. With the increase of MAP content, the slope of G′ curve is softened with a lower slope coefficient due to the elevation of MAP effect as shown from 0% MAP to 5% MAP. The plateau at low frequency range is more clearly observed, and is eventually independent of the frequency with further increment of MAP from 10 to 20%, showing the formation of networks. Ascending G′ moduli due to MAP content prove that DA homodimerizations reduce the mobility of the polymer chains and reinforce the copolymer viscoelastic properties.
4. Conclusion
Two supramolecular copolymers, P(MAAM-co-St) and P(MAP-co-St), were prepared by radical copolymerization between styrene and monomers bearing double hydrogen bonding moieties. Properties of the copolymer-assembled networks such as glass transition temperature and viscoelasticity behavior can be controlled by altering the comonomer composition. Both G′ and G″ moduli increased with increasing MAAM or MAP contents, and a plateau with high modulus appears in P(MAP-co-St) with MAP content above 10 mol%. These controllable properties were attributed to the strength of the dimers formed by hydrogen bonds. The hydrogen bonding strength has been quantified by 1H NMR spectroscopy at different concentrations to obtain K calculated at 6.75 M−1 for MAAM and 16.75 M−1 for MAP. Furthermore, the stronger hydrogen bond interaction of P(St-co-MAP), compared to P(St-co-MAAM), was proved by applying Kwei equation to Tg dependence against copolymer composition and by solubility tests. This study takes great advantage of simple synthesis procedures of the supramolecular polymers compared to common complicated synthetic methods that refrain potential developments. The resulting double association by homodimerization endows cross-linked PS-based copolymers with good properties above Tg from monomers industrially available or easy to prepare.
Acknowledgments
The authors thank the China Scholarship Council who attributed a Scholarship to Yiping NI. This study was realized at imp@ujm within the framework of an agreement between Université Jean-Monnet Saint-Etienne (France) and East China University of Science and Technology (China).
Disclosure statement
No potential conflict of interest was reported by the authors.
References
- Aakeröy C, Epa K. Controlling supramolecular assembly using electronic effects. Top Curr. Chem. 2012;1–23.
- Du JZ, Tang YP, Lewis AL, Armes SP, pH-sensitive vesicles based on a biocompatible zwitterionic diblock copolymer. J. Am. Chem. Soc. 2005;17982–17983.
- Lee OS, Stupp SI, Schatz GC. Atomistic molecular dynamics simulations of peptide amphiphile self-assembly into cylindrical nanofibers. J. Am. Chem. Soc. 2011;133:3677–3683.10.1021/ja110966y
- Ghosh A, Lee WB. Numerical studies on the thermal tuning of domain size in supramolecular diblock copolymer melts. Macromol. Res. 2011;19:483–486.10.1007/s13233-011-0510-z
- Lehn JM. Supramolecular chemistry – scope and perspectives molecules, supermolecules, and molecular devices (nobel lecture). Angew. Chem. Int. Ed. Engl. 1988;27:89–112.10.1002/(ISSN)1521-3773
- Binder WH, Zirbs R. Supramolecular polymers and networks with hydrogen bonds in the main- and side-chain. Hydrogen Bond. Polym. 2007;207:1–78.
- Patra D, Ramesh M, Sahu D, Padhy H, Chu CW, Wei KH, Lin HC. Enhancement of photovoltaic properties in supramolecular polymer networks featuring a solar cell main-chain polymer H-bonded with conjugated cross-linkers. Polymer. 2012;53:1219–1228.10.1016/j.polymer.2012.01.026
- Wan DC, Chen F, Kakuchi T, Satoh T. Guest release and solution behavior of a hydrogen-bonding physical micelle during chemoresponsive shell disruption. Polymer. 2011;52:3405–3412.10.1016/j.polymer.2011.05.025
- Chen XM, Lu XM, Cui K, Cui W, Wu J, Lu QH. Precipitation supramolecular complex for photoinduced anisotropic material with dual mesogenic units. Polymer. 2011;52:3243–3250.10.1016/j.polymer.2011.02.023
- Chiang WH, Lan YJ, Huang YC, Chen YW, Huang YF, Lin SC, Chern CS, Chiu HC. Multi-scaled polymersomes from self-assembly of octadecanol-modified dextrans. Polymer. 2012;53:2233–2244.10.1016/j.polymer.2012.03.030
- Chen YY, Lin HC. Metallo-homopolymer and metallo-copolymers containing light-emitting poly(fluorene/ethynylene/(terpyridyl)zinc(II)) backbones and 1,3,4-oxadiazole (OXD) pendants. Polymer. 2007;48:5268–5278.10.1016/j.polymer.2007.06.040
- Zou G, Wang YL, Zhang QJ, Kohn H, Manaka T, Iwamoto M. Molecular structure modulated properties of azobenzene-substituted polydiacetylene LB films: chirality formation and thermal stability. Polymer. 2010;51:2229–2235.10.1016/j.polymer.2010.03.029
- Folmer BJB, Sijbesma RP, Versteegen RM, van der Rijt JAJ, Meijer EW. Supramolecular polymer materials: chain extension of telechelic polymers using a reactive hydrogen-bonding synthon. Adv. Mater. 2000;12:874–878.10.1002/(ISSN)1521-4095
- Rieth LR, Eaton RF, Coates GW. Polymerization of ureidopyrimidinone-functionalized olefins by using late-transition metal ziegler-natta catalysts: synthesis of thermoplastic elastomeric polyolefins. Angew. Chem.-Int. Ed. 2001;40:2153–2156.10.1002/(ISSN)1521-3773
- Bureiko SF, Denisov GS. Dynamics of molecular hydrogen exchange in hydrogen-bonded systems. Pol. J. Chem. 2002;76:1177–1190.
- Bureiko SF, Denisov GS. Spectroscopic study of hydrogen exchange processes and structure of intermediate complexes with intermolecular hydrogen bonds. J. Mol. Struct. 2004;700:49–53.10.1016/j.molstruc.2003.11.054
- Adamson AW. A textbook of physical chemistry. New York (NY): Academic Press; 1986.
- Schalley C. Analytical methods in supramolecular chemistry. Weinheim: Wiley-vch Verlag GmbH & Co. KGaA; 2007.
- Ilhan F, Gray M, Rotello VM. Reversible side chain modification through noncovalent interactions. “Plug and Play” polymers. Macromolecules. 2001;34:2597–2601.10.1021/ma001700r
- Chen JS, Rosenberger F. Accurate NMR data evaluation for monomer shift, dimer shift and dimerization constant in a self associating system. Tetrahedron Lett. 1990;31:3975–3978.
- Horman I, Dreux B. Estimation of dimerisation constants from complexatin-induced displacements of 1H NMR chemical shifts: dimerisation of caffeine. Helv. Chim. Acta. 1984;67:754–764.10.1002/(ISSN)1522-2675
- Ośmiałowski B, Kolehmainen E, Dobosz R, Gawinecki R, Kauppinen R, Valkonen A, Koivukorpi J, Rissanen K. Self-organization of 2-acylaminopyridines in the solid state and in solution. J. Phys. Chem. A. 2010;114:10421–10426.10.1021/jp1063116
- Ośmiałowski B, Kolehmainen E, Kalenius E, Behera B, Kauppinen R, Sievänen E. Intermolecular steric hindrance in 7-acylamino-[1H]-2-oxo-1,8-naphthyridines: NMR, ESI-MS, IR, and DFT calculation studies. Struct. Chem. 2011;22:1143–1151.10.1007/s11224-011-9808-x
- Beijer FH, Sijbesma RP, Kooijman H, Spek AL, Meijer EW. Strong dimerization of ureidopyrimidones via quadruple hydrogen bonding. J. Am. Chem. Soc. 1998;120:6761–6769.10.1021/ja974112a
- Brunsveld L, Folmer BJB, Meijer EW, Sijbesma RP. Supramolecular polymers. Chem. Rev. 2001;101:4071–4098.10.1021/cr990125q
- Hirschberg J, Beijer FH, van Aert HA, Magusin P, Sijbesma RP, Meijer EW. Supramolecular polymers from linear telechelic siloxanes with quadruple-hydrogen-bonded units. Macromolecules. 1999;32:2696–2705.10.1021/ma981950w
- De Greef TFA, Smulders MMJ, Wolffs M, Schenning A, Sijbesma RP, Meijer EW. Supramolecular polymerization. Chem. Rev. 2009;109:5687–5754.10.1021/cr900181u
- Oishi T, Lee YK, Nakagawa A, Onimura K, Tsutsumi H. Syntheses and polymerizations of novel chiral poly(acrylamide) macromonomers and their chiral recognition abilities. J. Polym. Sci. Part A: Polym. Chem. 2002;40:1726–1741.10.1002/(ISSN)1099-0518
- Kao HC, Kuo SW, Chang FC. Effects of inert diluent segment and hydrogen bonding in poly(styrene-co-methacrylamide) copolymers. J. Polym. Res. 2003;10:111–117.10.1023/A:1024978102847
- Coskun M, Tem¨¹z MM, Demirelli K, Characterization and thermal degradation of poly (2-methacrylamidopyridine). Pol. Degrad. Stab. 77 (2002) 371–376.
- Coşkun M, Barim G, Temüz MM, Demirelli K. A study on thermal stabilities of poly(2-methacrylamidopyridine)-poly(methyl methacrylate) blends. Polym.-Plas. Technol. Eng. 2005;44:677–686.10.1081/PTE-200057816
- Woodward PJ, Hermida Merino DH, Greenland BW, Hamley IW, Light Z, Slark AT, Hayes W. Hydrogen bonded supramolecular elastomers: correlating hydrogen bonding strength with morphology and rheology. Macromolecules. 2010;43:2512–2517.10.1021/ma9027646
- Corbin PS, Zimmerman SC. Self-association without regard to prototropy. A heterocycle that forms extremely stable quadruply hydrogen-bonded dimers. J. Am. Chem. Soc. 1998;120:9710–9711.10.1021/ja981884d
- Lange RFM, Van Gurp M, Meijer EW. Hydrogen-bonded supramolecular polymer networks. J. Polym. Sci. Part A: Polym. Chem. 1999;37:3657–3670.10.1002/(ISSN)1099-0518
- Hofmeier H, Hoogenboom R, Wouters MEL, Schubert US. High molecular weight supramolecular polymers containing both terpyridine metal complexes and ureidopyrimidinone quadruple hydrogen-bonding units in the main chain. J. Am. Chem. Soc. 2005;127:2913–2921.10.1021/ja042919e
- Söntjens SHM, Sijbesma RP, van Genderen MHP, Meijer EW. Stability and lifetime of quadruply hydrogen bonded 2-ureido-4[1H]-pyrimidinone dimers. J. Am. Chem. Soc. 2000;122:7487–7493.10.1021/ja000435m
- Feldman KE, Kade MJ, Meijer E, Hawker CJ, Kramer EJ. Model transient networks from strongly Hydrogen-bonded polymers. Macromolecules. 2009;42:9072–9081.10.1021/ma901668w
- Subramani C, Dickert S, Yeh YC, Tuominen MT, Rotello VM. Supramolecular functionalization of electron-beam generated nanostructures. Langmuir. 2011;27:1543–1545.10.1021/la1039514
- McKee MG, Elkins CL, Park T, Long TE. Influence of random branching on multiple hydrogen bonding in poly(alkyl methacrylate)s. Macromolecules. 2005;38:6015–6023.10.1021/ma050667b
- Park T, Zimmerman SC. Formation of a miscible supramolecular polymer blend through self-assembly mediated by a quadruply hydrogen-bonded heterocomplex. J. Am. Chem. Soc. 2006;128:11582–11590.10.1021/ja0631854
- Park T, Zimmerman SC. Interplay of fidelity, binding strength, and structure in supramolecular polymers. J. Am. Chem. Soc. 2006;128:14236–14237.10.1021/ja065469u
- Sartorius J, Schneider HJ. A general scheme based on empirical increments for the prediction of hydrogen-bond associations of nucleobases and of synthetic host–guest complexes. Chem. – A Eur. J. 1996;2:1446–1452.10.1002/(ISSN)1521-3765
- Hisamatsu Y, Fukumi Y, Shirai N, Ikeda S-I, Odashima K. Five-membered heterocyclic ureas suitable for the donor–donor–acceptor hydrogen-bonding modules. Tetrahedron Lett. 2008;49:2005–2009.10.1016/j.tetlet.2008.01.068
- Zimmerman SC, Corbin FS. Heteroaromatic modules for self-assembly using multiple hydrogen bonds. Mol. Self-Assembly. 2000;96:63–94.
- Plante A, Mauran D, Carvalho SP, Pagé J, Pellerin C, Lebel O. Tg and rheological properties of triazine-based molecular glasses: incriminating evidence against hydrogen bonds. J. Phys. Chem. B. 2009;113:14884–14891.10.1021/jp905268a
- Chapkanov AG, Zareva SY, Nikolova R, Trendafilova E. Synthesis and spectroscopic investigation of (acetylamino)pyridines. Collect. Czech. Chem. Commun. 2009;74:1295–1308.10.1135/cccc2009056
- Hsu WP. Phase behavior and a modified Kwei equation for ternary polymer blends containing stereoregular PMMA. Thermochim. Acta. 2006;441:137–143.10.1016/j.tca.2005.12.013
- Slark A. Application of the Kwei equation to the glass transition of dye solute–polymer blends. Polymer. 1999;40:1935–1941.10.1016/S0032-3861(98)00435-2