Abstract
In this work, for the first time, alginate dialdehyde (AD) has been used as a cross linker to prepare casein films. The films, synthesized, have been characterized by Fourier transform infrared spectroscopy, X-ray diffraction analysis and scanning electron microscopy and thermogravimetric analysis. The films were investigated for their water absorption capacity in the physiological fluid of pH 7.4 at 37 °C. The film samples (AD-X-CAS)12, (AD-X-CAS)24 and (AD-X-CAS)36 demonstrated an equilibrium water absorption of 30.86, 26.15 and 11.93 g/g, respectively. Moreover, the swelling exponents for the films were in the range of 0.66–0.75, thus indicating non-Fickian water transport mechanism. The dynamic water uptake data were analysed by various kinetic models. The water vapour transmission rates (WVTR) for the three film samples were found to be 16587, 15900 and 14316 g/m2/day, respectively. The higher values of WVTR, obtained for all the three samples, indicated their suitability for high exudating wounds. The results of expansion study in 4% gelatin medium revealed that the three samples exhibited an almost 2.54, 2.09 and 1.90-fold increase in their diameter in 90, 75 and 60 min, respectively.
1. Introduction
Hydrogels are three-dimensional polymer networks with the ability to take in large quantities of water and solutes. Owing to the soft and rubbery nature upon hydration, they may be used as tissue substitutes where similar physical attributes permit biocompatibility. However, for biomedical applications it is desirable to use eco-friendly and biocompatible polymers.[Citation1] Some of the major applications include drug delivery,[Citation2] porous sponges for nerve re-generation,[Citation3] artificial cartilage [Citation4] and wound dressings.[Citation5] In recent past, a number of biopolymers like chitosan,[Citation6] starch,[Citation7] carrageenan,[Citation8] alginates,[Citation9] gelatin,[Citation10] collagen,[Citation11] etc. have been exploited for the above-mentioned biomedical applications. In order to fabricate a polysaccharide/protein-based matrix with well-defined network and fair structural integrity, it is essential to crosslink it with some appropriate crosslinking agent like glutaraldehyde,[Citation12] glyoxal,[Citation13] formaldehyde,[Citation14] genipin [Citation15] etc. However, owing to the toxicity of these compounds, sincere attempts are being made to have an alternative strategy to fabricate these biopolymers-based hydrogels. In recent past, one of the most widely used strategy adopted, is to chemically modify a biopolymer and use it to crosslink another suitable biopolymer. In this way, a non-toxic hydrogel matrix with desired physic-chemical properties can fabricated. There are several reports describing periodate-induced oxidation of polysaccharides to yield dialdehyde derivative. For example, Li et al. [Citation16] have investigated potassium periodate-induced controlled oxidation of carboxymethyl cellulose (CMC) under various experimental parameters such as pH, temperature, concentration of periodate etc. In another work by Salisu et al. [Citation17], starch has been oxidized to dialdehyde starch by potassium periodate and subsequently crosslinked by Cu (II) ions to produce hydrogels. In another interesting work by Pascalau and co-workers [Citation18], dialdehyde alginate (DA), obtained by periodate-oxidation, was crosslinked with K-carrageenan using adipicdihydrazide as cross-linker. The resulting hydrogel was characterized by various techniques. In a recent report,[Citation19] sodium alginate (SA) was oxidized to alginate dialdehyde (AD) via periodate-initiated oxidation and then allowed to react with gelatin to form hydrogels, both with and without nano-scaled bioactive glass (45S5) particles. In vitro experiments showed good proliferation after seeding on films and an increase of mitochondrial and LDH activities.
In the present work, we have investigated water absorption properties of a unique hydrogel system obtained by crosslinking of casein with DA via coupling reaction. The system is being reported for the first time and its two components, namely DA casein have extremely fair reputation as non-toxic biocompatible polymers. Such a system can frequently be employed for different biomedical applications such as wound dressings and as oral drug delivery system. According to a report [Citation20], total protein content in one litre of milk is around 33 g, and casein constitutes almost 80% of this total protein content. Casein, a proline-rich globular protein which is readily available in milk has both hydrophilic and hydrophobic domains.[Citation21] It is a phosphoprotein, with an open and random coil structure,[Citation22] which is also responsible for the formation of transparent films from aqueous casein solution. Casein has been reported to have a number of biomedical applications.[Citation23] Alginate, a naturally occurring biopolymer, consists of linear chains of (1,4)-β-D mannuronic acid (M) and (1,3)-α-L-guluronic acid (G) monomers, with varying composition and sequence depending upon a number of factors like biological sources, growth and seasonal conditions.[Citation24] Moreover, alginate can be regarded as a true block copolymer composed of homo-polymeric regions of M and G, termed M- and G-blocks, respectively, interspersed with regions of alternating structure. Finally, alginates have no regular repeating unit and that the distribution of the monomers along the polymer chain could not be described by Bernoullian statistics. Knowledge of the monomeric composition is hence not sufficient to determine the sequential structure of alginates. There have been several reports, describing various biomedical applications of alginates such as tissue engineering,[Citation25] oral drug delivery,[Citation26] wound dressings,[Citation27,28] etc.
2. Experimental
2.1. Materials
SA (weight average molar mass (Mw) of 150 kDa and with(1-4)-b-D-mannuronic acid (M) is to (1-4)-a-L-guluronic acid (G) ratio, i.e. M/G ratio of 1.62, Hi Media Chemicals, Mumbai, India), casein powder (Casein; alkali soluble, obtained from bovine milk, molar mass 45 kDa, with purity of 99.6%, Research Lab, Pune, India), potassium periodate (KIO4;analytical grade, Hi Media, Mumbai, India), ethylene glycol (Merck, Mumbai, India) were obtained in excellent conditions and used as such. Other chemicals such as sodium hydroxide, sodium dihydrogen phosphate, were obtained from Central Drug House, Mumbai, India. The double distilled water was used throughout the investigations.
2.2. Methods
2.2.1. Preparation of AD
The alginate was oxidized using potassium periodate by the method described elsewhere [Citation29], however with some modifications. In a typical experiment, 3 g of SA was dissolved in 100 mL of pre-warmed distilled water under mild stirring for a period of 2 h to ensure its complete dissolution. Now, 2 g of potassium periodate was added under gentle stirring (molar ratios between guluronate unit and periodate were 100:70) and the reaction mixture was placed in dark under gentle stirring for a period of 12 h. Thereafter, the mixture was taken out and the reaction was quenched by the addition of ethylene glycol (molar ratio of ethylene glycol: potassium periodate 1:1). The AD was purified by precipitation with the addition of NaCl (2.500 g) and 100 mL of ethanol. The polymer was again dissolved in water (100 mL) and re-precipitated by the addition of ethanol (200 mL) in the presence of NaCl (1.00 g). The process was repeated using NaCl (0.50 g in 100 mL ethanol) and the polymer was precipitated with acetone (100 mL) under its sodium salt form. Finally, the precipitate was washed in ethanol (100 mL) under stirring during 15 min, isolated and dried at room temperature under vacuum. In order to determine the % DO, fully oxidized alginate dialdehyde (FOAD) was prepared, by allowing alginate and periodate to react in the molar ratios of guluronate to periodate as 100:120. In order to ensure complete oxidation, reaction time was kept as 24 h.
The degree of oxidation (% DO,) of AD was defined as the number of oxidized guluronate residues per 100 guluronate units, and determined by estimating the aldehyde content in the oxidized alginate with reference to the fully oxidized alginate as the reference compound. The principal of estimation was same as described elsewhere [Citation16]. In brief, a pre-calculated quantity, i.e. 0.4 g of AD, prepared as described above, was dissolved in 20 mL of distilled water and to this solution was added 20 mL of 1.0 mol/L hydroxylamine hydrochloride (NH2OH·HCl). The reaction mixture was allowed to be kept at 50 °C for a period of 4 h. The amount of HCl released was titrated with 1 M NaOH. The titre value recorded was V1. Now, the same protocol was repeated with FOAD and the titre value V2 was recorded. The % DO was calculated as:
2.2.2. Preparation of AD-crosslinked casein films
The AD-crosslinked-CAS films were prepared by the Schiff base formation reaction between the aldehyde group of AD and –NH2 groups of casein. The method was similar to that reported by Saraswathy et al. [Citation30]. In an experiment, 0.75 g of casein powder was dissolved in 15 mL of 1.5% NaOH solution and to 90 mg of cross-linker AD was added under mild stirring for 15 min to ensure complete dissolution. The reaction solution was poured into Petri plate and allowed to keep in electric oven (Tempstar, India) for a period of 6 h at 60 °C. Here, is noteworthy that in preliminary studies, we carried out the crosslinking reaction for different time periods and found that a time period of 6 h was sufficient to produce a well-shaped stable film. The film, thus formed, was peeled off and placed in an electric oven (Tempstar, India) at 40 °C and its mass measurements were made regularly after every 24 h till the film attained constant weight. We observed that it took almost 72 h for the film to dry completely. In all, three samples were prepared by adding 90, 180 and 270 mg of cross-linker AD. These film samples were designated as (AD-X-CAS)12, (AD-X-CAS)24 and (AD-X-CAS)36, where the number subscripted outside the parenthesis denotes the weight per cent of cross-linker AD with respect to the weight of casein in the film forming solution. The thickness of these samples, as measured using screw gauze (Harshman Gauges Mumbai, India) was found to be 0.525 ± 0.0285, 0.567 ± 0.0310 and 0.512 ± 0.0261 for (AD-X-CAS)12, (AD-X-CAS)24 and (AD-X-CAS)36, respectively.
2.3. Characterization of films
The Fourier transform infrared spectroscopy (FTIR) Spectrum of synthesized polymer was recorded on FTIR-spectrophotometer (Shimadzu, 8400S) using KBr. For this, dry polymer was grinded, mixed with KBr and transformed into pellet. The scans recorded were the average of 100 scans and spectral range selected was 400–4000 cm−1. 1H NMR spectra of SA and oxidized SA were recorded in D2O using Bruker avance 400(FT NMR) spectrometer at SAIF CDRI, Lucknow (India). The thermogravimetric analysis was performed using a thermo gravimetric analyser (Mettler, Toledo TGA/SDTA 851, Switzerland). A definite quantity of powdered sample was placed in a ceramic crucible and analysed over the temperature range of 30–600 °C, at the heating rate of 10 °C min−1 under the constant flow of N2 at the rate of 30 mL min−1. The X-ray diffraction (XRD) method was used to measure the crystalline nature films. These measurements were carried out on a Rikagu Diffractometer (Cu radiation = 0.1546 nm) running at 40 kV and 40 mA. The diffractogram was recorded in the 2θ range 5–50° at the speed rate of 2° per min. In order to investigate the surface morphology of films, scanning electron microscopy (SEM) and AFM images were recorded at Indian Institute of Information Technology and Designed Materials, Jabalpur, India
2.4. Tensile properties measurement
In order to investigate the effect of plasticizer glycerol on the tensile strength, the sample (AD-X-CAS)24 was selected for study. We prepared five such samples, by adding different amounts of glycerol, i.e. 0, 10, 20 and 30 and 40 wt % of casein in the film forming solution. After pre-conditioning the films at 50% relative humidity (RH), films were cut into 5 mm × 35 mm strips with a razor blade; then, the strips were allowed to equilibrate at ambient humidity overnight before mechanical testing. The thickness of an equilibrated film strip was measured at five points with ± 0.001 mm precision using a micrometre thickness gauge (India), and averaged. The mechanical properties of the films were determined using Unique Tensile Tester (UTM, Universal Tensile Tester, and Pune, India) according to the standard testing method ASTM D882-97.[Citation31] The measurements were made at 25 °C under the constant humidity of 50%. The samples were cut into strips, each of width 5 mm and length 50 mm. The initial grip separation and mechanical crosshead speed were set at 25 and 100 mm per min, respectively. The various formulae used are shown as below:(1)
where F is the maximum load and MA is the minimum cross-sectional area of the film specimen. Results were converted to mega Pascal units (MPa).(2)
(3)
L0 refers to initial length of the film sample and L is the elongation when the film breaks.
2.5. Water absorption study
The water absorption capacity of bio-polymeric films was investigated gravimetrically as described in our previous work.[Citation32] A pre-weighed film sample was placed in physiological buffer (PB; pH7.4) at 37 °C and mass measurements were made periodically till the attainment of equilibrium. The swelling ratio (SR) was calculated by the following well-known expression:(4)
where Wt is the swollen mass of the hydrogel film at time ‘t’ and W0 is the dry mass of the hydrogel film. In order to determine the equilibrium swelling ratio (ESR), Wt was replaced by We which is the mass of hydrogel film at equilibrium. The investigations were made in triplicate and the data have been given as mean ± SD.
2.6. Moisture permeation study
A modified ASTM method (inverted cup, E96-90, Procedure D) was employed to study the moisture permeation capacity of film samples.[Citation33] For this, a poly (propylene) cup, filled with 5 g of distilled water, and fitted with the test film sample at its mouth, was placed inside a plastic jar which contained saturated solution of lithium chloride to produce RH of 20% at 32 °C. The cup was taken out periodically and weighed using an electronic balance (Denber, Germany). The moisture, permeated through the film, was calculated in the terms of water vapour transmission rate (WVTR) using the following expression:(5)
where m = weight loss in g, A = area of cross section of test sample (m2), ∆t = time-interval (day).
These experiments were also done in triplicate and the average data is reported.
2.7. Film expansion study
In order to mimic the behaviour of film on the surface of the wound during the healing process, its expansion was monitored on the aqueous solution of gelatin, as described elsewhere [Citation34]. In brief, a 4% (w/v) solution of gelatin was prepared by dissolving 4 g of gelatine in 100 mL of water at 95 °C. Now, the solution was filtered through a sieve to remove any insoluble impurity and 25 mL of this solution was transferred into a Petri plate and allowed to keep overnight. Finally, the circular piece of test film was placed on the solution and change in its diameter was monitored periodically. The expansion ratio (ER) was determined as per following expression:(6)
3. Results and discussion
3.1. Rationale for the selection of polymers
Alginate is a naturally occurring anionic hydrophilic biopolymer with a number of outstanding properties such as biocompatibility, biodegradability, non-antigenicity and chelating ability.[Citation35] This has been approved by Food and Drug Administration department of USA and has been exploited for a large number of biomedical applications. The basic idea, lying behind the oxidation of alginate to produce AD, was that increase in the number of reactive aldehyde groups could render a better adhesiveness and a faster degradation rate.[Citation36] In addition, the reason for forming the aldehyde functionality is to give a functional moiety to cross-link to the casein. The other polymer, casein is a milk protein and it has great hydrophilic nature. However, its films are readily soluble in water and this puts restrictions on its use for a number of biomedical and other applications where structural integrity and insoluble nature is required.[Citation37] Therefore, crosslinking of casein by oxidized alginate could be a most suitable strategy to produce a film matrix with all desired physic-chemical properties for biomedical applications.
3.2. Oxidation of alginate to AD
When SA is allowed to react with potassium periodate under dark conditions, the –OH groups, present at –C2 and –C3 positions of uronic units are oxidized to aldehyde, thus producing AD. In order to confirm the formation of AD from alginate, FTIR spectra of both alginate and its oxidized form were recorded.
Figure (a) and (b) shows FTIR spectrum of Alginate and DA, respectively. In the FTIR spectrum of alginate two strong bands, appearing at 1599 cm−1 and 1419 cm−1 are attributed to asymmetric and symmetric stretching vibrations of −COO− groups on the polymer backbone, respectively. In addition, there appear the polysaccharides specific bands at 1035–1084 cm−1 of the C–O–C (cyclic ether) stretching vibration, the bands at 2885 cm−1 of C–H stretching, and a broad band due to the hydrogen bound OH groups appears between 3200 and 3600 cm−1 attributed to the complex vibrational stretching, associated with free, inter and intra-molecular bound hydroxyls. The periodate-induced oxidation of alginate can be remarked in the FTIR spectrum of AD, shown in Figure (b). Firstly, there is new peak at 1629 cm−1, which is characteristic for C=O group of the dialdehyde product of the OH groups oxidation process. Moreover, the C–O–C (cyclic ether) band at 1033 cm−1 is reduced remarkably as a result of the chains cleavage. Another indication on the oxidation process via OH groups of alginate is the appreciable decrease in the intensity of the band between 3200 and 3600 cm−1 due to the OH vibrations. In this way, it may be inferred that alginate has transformed into its dialdehyde form due to periodate oxidation.
Figure 1. FTIR spectrum of (a) sodium alginate and (b) alginate dialdehyde, (c) casein, and (d) AD-crosslinked casein film.
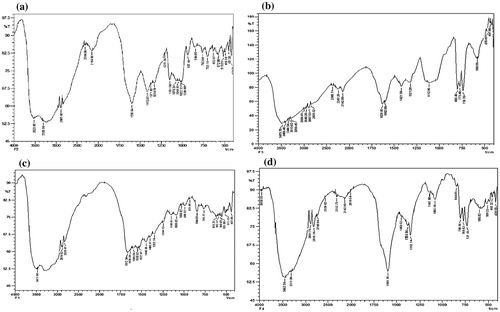
The degree of oxidation (% DO), determined by the Schiff’s reaction, was calculated to be 57.7%.
The results of 1H NMR spectroscopy of native alginate and AD are shown in Figure (a) and (b), respectively. The signals observed at 5.18, 5.35 and 4.92 ppm correspond to peaks of H1-G, H1-M and H5-G, respectively, shown in Figure (a). Signals observed at 3.60 and 4.13 ppm were assigned for H3-M and H3-G, respectively.
However in the spectrum of sample AD, shown in Figure (b), the proton signal modification of the H1 and H5 signals for M and G units at 5.39 and 4.93 ppm confirm the oxidation of Na-alginate. Moreover, the appearance of two new signals at 5.66 and 5.05 ppm, which would correspond to a hemiacetalic proton formed from aldehyde and neighbours hydroxyl groups, also confirm the proposed modification. The alkyl side chains attached to oxidized alginates were determined by 1H NMR spectrometry and the signal was recorded at 1.19 ppm.[Citation38] The signal observed at 2.1 ppm was assigned for D2O solvent in the both recorded samples.
3.3. Formation of (AD-X-CAS) films
As stated earlier, the Schiff base reaction between the aldehyde groups from the oxidized alginate and –NH2 groups from casein results in the formation of AD crosslinked casein films. Now, the AD, thus produced, is allowed to react with casein, thus resulting in the formation of (AD-X-CAS) films through the Schiff base coupling reaction between the aldehyde groups of AD and amino groups from casein molecules. The overall mechanism for the formation of film has been illustrated in Figure .
In order to further confirm the above reaction, FTIR spectra of plain casein and AD-X-CAS films were recorded. The results, as shown in Figure (c) and (d), establish the Schiff base reaction and formation of the product. The spectrum of casein film, shown in Figure (c), displays characteristic peaks of various functionalities present in the protein molecules. It is known that casein has random coil nature and has fair capacity to form intermolecular H-bonding. In the spectrum a broad absorption band appears in the region of 3000–3600 cm−1 which may probably be attributable to H-bonding interactions between caseinate and –OH from DAs.[Citation39] The absorption from unbounded –NH groups also lies in the same region. In addition, amide I and amide II peaks are also visible in the region of 1500–1700 cm−1. Furthermore, peaks at 1635 cm−1 in the amide I region and 1527 cm−1 in the amide II region could be assigned to the stretching of the carbonyl group (C=O) and to the symmetric stretching of N–C=O bonds, respectively. The spectrum of DA-X-CAS film (see Figure (d)) shows a sharp distinct peak at 1596 cm−1, which is attributed to the C=N stretching vibration of the imines group of Schiff bases, a structure, which should only occur after successful crosslinking of amine groups of casein and AD.[Citation40]
3.4. Characterization of (AD-X-CAS) films
The thermal stability of the film samples CAS, (AD-X-CAS)12 and (AD-X-CAS)36 was investigated and the respective thermograms are shown in Figure (a)–(c), respectively. The thermogram of pure casein film shows two-stage degradation. The initial weight loss of around 20% occurs from ambient to 190 °C and is simply attributable to the loss of water molecules that are associated with the polar groups of casein. The second thermal event starts at 200 °C and continues up to 600 °C with a higher weight loss of around 45%, and is attributable to degradation of casein backbone. In this way, total weight loss suffered by casein film in the temperature range of ambient to 600 °C was almost 65%. The thermogram of film sample (AD-X-CAS)12, shown in Figure (b), reveals an improved thermal stability in the same temperature range studied. The total weight loss, suffered between ambient to 600 °C was nearly 30%. Such a great improvement in the thermal stability is attributable to the presence of crosslinked network within the casein film.[Citation41]
In fact, crosslinking of the casein film by AD makes the film stiffer and compact. In a study by Mu et al. [Citation42], the dialdehyde CMC – crosslinked gelatin films also showed an enhanced thermal stability as compared to the plain gelatin film. However, the film sample (AD-X-CAS)36 shows almost the opposite trend. Although the film is more cross-linked (i.e. amount of cross-linker AD is 36% of the weight of casein in the film) as compared to the film (AD-X-CAS)12, but it shows remarkable decrease in its thermal stability. This may probably be due to the fact that cross-linker AD has low thermal stability and since its concentration in this film sample is almost 36%, it contributes towards lower the overall thermal stability of the film. Therefore, it may be concluded that AD-crosslinked casein film shows more stability, but using a higher concentration of cross-linker AD will result in decrease in the thermal stability.
The surface morphology of the films was also investigated by SEM analysis. The surface textures of plain casein film was compared with that of sample (AD-X-CAS)24 was compared. The SEM images of plain and crosslinked film are shown by the Figure (a)–(f) at the magnifications of 600, 1000 and 2000×, respectively. A simultaneous comparison of these SEM images reveals that the plain casein samples have very rough texture with micrometre sized casein particle dispersed throughout. On the other hand, the crosslinked film (AD-X-CAS)24 has relatively less rough surface, probably due to the crosslinking of casein molecules with AD. The overall conclusion is that both of the films do not have smooth surface and the presence of granules may provide appreciable porosity to these films.
Figure 5. SEM images of plain casein and (AD-X-CAS)24 film samples at (a) and (b) 600×; (c) and (d) 1000×; and (e) and (f) 2000× magnifications, respectively.
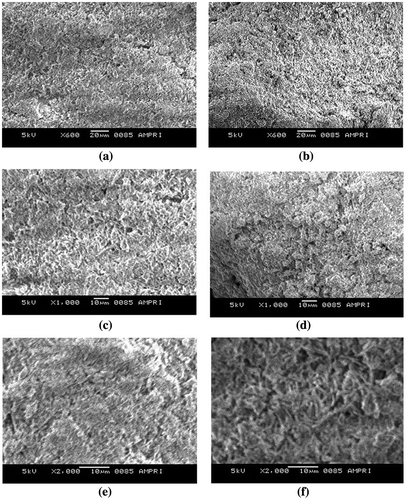
Finally, the XRD patterns of plain casein and (AD-X-CAS)24 film samples are shown in Figure (a) and (b), respectively. The scattered broad pattern, observed in Figure (a), is indicative of the amorphous nature of casein as has also been reported elsewhere [Citation43]. Furthermore, The XRD pattern of film sample (AD-X-CAS)24 also appears to be almost diffused, thus indicating amorphous phase.
3.5. Water absorption behaviour of films
When a polymeric film is applied on a wound, it absorbs the exudates coming out from the wound and simultaneously releases the entrapped bioactive agent. In this way, wound dressings films are swelling dependent drug release devices. Therefore, it becomes essential to make a thorough investigation of water absorption capacity of these films. The dynamic water uptake data of the film samples (AD-X-CAS)12, (AD-X-CAS)24 and (AD-X-CAS)36 was studied in the physiological fluid (PF) of pH 7.4 at 37 °C. The results, as shown in Figure (a), reveals that at a given time the water absorption or SR decreases with the increase in the amount of crosslinking agent AD in the film.
Figure 7. (a) Dynamic water uptake of film samples (AD-X-CAS)12, (AD-X-CAS)24 and (AD-X-CAS)36 in the PF of pH 7.4 at 37 °C; interpretation of dynamic water uptake data by (b) power function law, (c) Beren–Hopfenberg model and (d) Schott model, respectively, and (e) dynamic water vapour permeation through the various film samples.
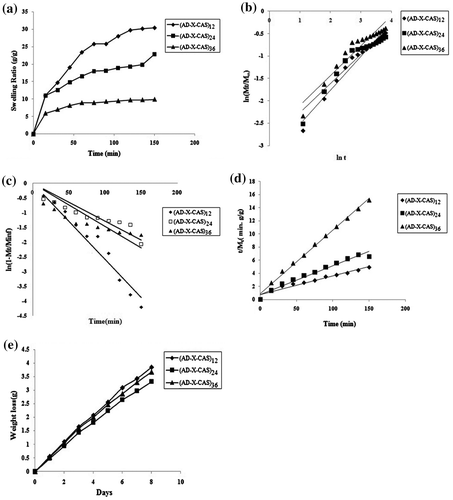
The ESR, demonstrated by the three film samples was, 30.86 ± 2.05, 26.15 ± 1.69 and 11.93 ± 1.17 g/g, respectively. It can be seen that as the amount of cross-linker AD increase, water uptake decreases. This is a commonly observed phenomenon [Citation44] and attributable to the fact that with the increase in the amount of cross-linker, the number of crosslinks between the chains increases, thus reducing the free space available for incoming water molecules. This results in decrease in the water absorption .One more reason is that with the increase in the extent of crosslinking, the number of crosslink points along the casein chains increases, thus rendering the chains stiffness. As a result, the relaxation of casein chains is suppressed, thus resulting in decrease in the water uptake.[Citation45]
The kinetic water absorption data, obtained with polymeric hydrogels, is usually interpreted by a number of kinetic models such as Power function law, Beren–Hopfenberg kinetic model, Schott model, etc.[Citation46] The various kinetic models, applied in this study, are given in Table .
Table 1. Various kinetic models used in the present work.
The linear plots, obtained for various kinetic models using the water uptake data, are shown in Figure (b)–(d) for power function law, Beren–Hopfenberg model and Schott model, respectively. Values of various parameters associated with these models are given in Table . A close look at the values displayed reveals some significant facts about the mode of water penetration into the polymeric hydrogels. The values of swelling exponent ‘n’, determined using power function law, are in the range of 0.65 ± 0.06–0.75 ± 0.08 for all the three samples studies. This suggests that for all the film samples the mechanism of water penetration in to film matrix is non-Fickian or anomalous for which 1.0 > n > 0.5.[Citation47] This indicates that the rate of diffusion of water into polymer matrix and the rate of relaxation of polymeric chains are quite comparable, thus confirming the existence of certain coupling between molecular diffusion and tension relaxation which are developed during swelling of the hydrogel films.[Citation48] As the polymer CAS and cross-linker AD have a number of polar groups i.e. hydroxyls and carboxylic groups along their macromolecular chains, the interactions of these polar groups with invading water molecules and chain relaxation of polymeric segments during the swelling process are the significant factors which contribute towards overall swelling mechanism. Therefore, it may be concluded that for the initial 60% water uptake, the hydrogel films follow anomalous or non-Fickian diffusion process. The anomalous swelling of polysaccharides/protein-based hydrogels is also reported by others. For example, Bueno et al. [Citation49] have reported that crosslinked xanthan hydrogels exhibit non-Fickian swelling behaviour. It is well known that Power function model is only applicable up to the 60% of the initial swelling data. Therefore, we also applied First-order kinetic model on the water uptake data.[Citation50] The regression values obtained for this model are very low and indicate unsuitability of this model to interpret the kinetic water absorption data. Finally, the Schott model, applicable in the case of long-term swelling kinetics, was applied.[Citation51] The respective parameters along with their regressions indicate that this model is fairly suitable. A comparison between the values of M∞(theor) and M∞(exp) indicates a close agreement between them, thus further establishing superiority of this model to the others. Based on the regression values obtained for various models applied, the order of fitness was: Schott model > Power function law > First-order model.
Table 2. Parameters of various kinetic models applied.
3.6. Moisture permeation study
An ideal wound dressing must control the water loss from a wound at an optimal rate. Lamke et al. [Citation52] reported the evaporative water loss for normal skin as 2047 g/m2/day and that for injured skin can range from 2797 g/m2/day for a first-degree burn to 51,387 g/m2/day for a granulating wound. The water vapour permeability of a wound dressing should prevent excessive dehydration as well as build-up of exudates. It has been recommended that a rate of 2000–2500 g/m2/day would provide adequate level of moisture without risking wound dehydration.[Citation53] Surface drying not only impedes delivery of nutrients and immune defences to the wound surface, but also markedly impedes the ability of cells to migrate across the wound surface. Epithelial cells need a moisture layer to migrate and spread. In this work, the moisture loss vs. time profile, shown in Figure (e), were used to calculate the WVTR for the three film samples, namely (AD-X-CAS)12, (AD-X-CAS)24 and (AD-X-CAS)36. The values were found to be 16,587, 15,900 and 14,316 g/m2/day, respectively. It is clear that as the amount of cross-linker AD increases, the WVTR shows a decreasing trend. This is attributable to the fact that with the increase in the concentration of AD, the number of crosslinks increases, thus producing a denser network. This finally reduces the rate of water vapour transmission through the films.
A close look at the values obtained reveals that these values are much higher as compared to the values obtained in the case of majority of the wound dressing films. For example, Balakrishnan et al. [Citation54] have reported WVTR of AD/gelatin-based films as 2686 g/m2/day indicating that the hydrogel can maintain a moist environment over wound bed in moderate to heavily exuding wound which would enhance epithelial cell migration during the healing process. Moreover, Devi et al. [Citation55] have reported WVTR of chitosan-fibrin composite films in the range of 2118–2383 g/m2/day. However, there are some reports which claim relatively higher vapour transmission rates. For example, Sasikala et al. [Citation56] have claimed the WVTR of various manuka honey-loaded chitosan hydrogel films in the range of 7000–11,000 g/m2/day.
However, a relatively much higher WVTR range of 14,000–16,000 g/m2/day in the present study can be explained as follows: the moisture permeation is said to occur through following steps, (1) condensation of water vapour molecules on the surface of the film, (2) solubilisation of vapour molecules and their diffusion through the film matrix across the thickness of the film and finally (3) evaporation of migrated molecules from the other side of the film. The whole scheme has been illustrated in Figure (a).
Figure 8. (a) Mechanism of moisture permeation, (b) hindrance in moisture diffusion and (c) chain relaxation favouring the moisture diffusion through the film.
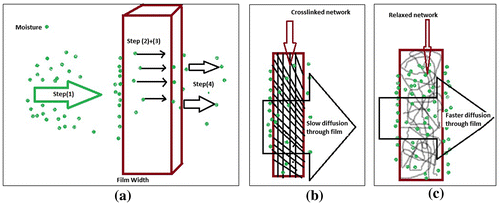
Now, in the present case as the film forming polymers casein and cross-linker oxidized alginate are highly hydrophilic due to presence of a number of polar groups, the step (1), i.e. condensation of vapour molecules takes place to a great extent. This results in accumulation of a higher concentration of water vapour molecules on the surface. Due to large concentration gradient of water vapour molecules along the thickness of the film, there is fast and appreciable diffusion of molecules towards other side of the film. It has been reported that during the diffusion of water vapour molecules through the width of the film, the crosslinked network of the film also puts hindrance towards diffusion process (see Figure (b)). However, in the present work, it is most probable that due to moisture absorption by the film, there are appreciable polymeric segmental movements within the film matrix, thus resulting in faster diffusion as shown in Figure (c). This also enhances the evaporation of vapour molecules from the other end of the film, thus enhancing WVTR. Looking to the higher water absorption and permeation rates of these polymeric films, they appear to be suitable for the wounds with higher exudates.
3.7. Expansion study
When a wound dressing film is placed over the wound, the exudate is absorbed by the film. This causes an increase in the size of the film. The essential requirement for the film is to maintain its structural integrity during the expansion process. The results of expansion study, carried out for all the three film samples are shown in Figure . It was found that the samples (AD-X-CAS)12, (AD-X-CAS)24 and (AD-X-CAS)36 exhibited an almost 2.54, 2.09 and 1.90-fold increase in their diameter in 90, 75 and 60 min, respectively. Indeed, the film sample with minimum cross-linker content shows highest increase in the diameter and vice versa. We noticed that after 36 h all the three samples maintained their integrity and had smooth and slippery surface due to absorption of gelatin (a 4% aqueous solution was used to mimic the wound surface). This experiment indicated the suitability of these films for application on high exudating wound. Here, it is worth mentioning that the thickness of the films was also increase during the expansion process. However, as the aspect ratio, i.e. ratio between diameters to thickness was too high; the marginal increase in thickness was not measured.
3.8. Mechanical strength study
The mechanical strength of a wound dressing film is a key factor to establish its suitability for application on a wound. When a wound dressing film is put on a wound, it absorbs exudates coming out from the wound. It also bears the external stress applied on it during its application and handling. The film must be able to withstand the external stress. In addition, per cent elongation (% PE) of the film is also a desirable property as the film must not be broken during its expansion owing to the absorption of fluid. The film sample (AD-X-CAS)24 was selected for the mechanical analysis. We prepared five samples, by adding different amounts of glycerol, i.e. 0, 10, 20 and 30 wt % of casein in the film forming solution. These samples were designated as F0–F4, respectively. The variation in TS and PE with amount of plasticizer glycerol (Gly) are shown in Figure (a) and (b), respectively.
It is observed in Figure (a) that as the Gly content in the film increases, there is drastic decrease in the TS of the films. Addition of 0.5 mL glycerol into control film F0, results in decrease in TS from 11.05 to 1.85 MPa. Further addition of Gly brings the TS of the resulting films to below unity. The observed decrease in TS due to addition of Gly is attributable to the fact that glycerol molecules occupy the space between the polymeric casein chains and lowers the interactions between the polar groups of casein molecules. Therefore, the strength of the resulting films continues to decrease with the addition of Gly. Similar results are also reported elsewhere [Citation57].
It appears that nature of film-forming polymer is also a major factor to impart strength to the films. For example, film made from Kefiran, a polysaccharide, is reported to have TS of 6.40 MPa,[Citation58] while ovalbumin (a protein) films, crosslinked with 1-ethyl-3-3-dimethylaminopropyl carbodiimide hydrochloride (EDC) remarkably lower TS, in the range of 0.40–0.74 MPa.[Citation59] The Gly added films, in the present work, also possess TS in the same range. The variation in PE with addition of Gly, as shown in Figure (b), demonstrates just opposite trend. The PE increases with Gly content, probably due to reduction in rigidity of the polymeric segments. In addition, addition of glycerol causes a decrease in the inter-molecular interactions between adjacent casein molecules. This interaction may be H-type between surface hydroxyls and NH2 groups of casein. From the above discussion, it is clear that AD crosslinked casein film has a fair tensile strength in the absence of glycerol, while addition of glycerol results in drastic decrease in the tensile strength of the resulting films and these glycerol added films, though with fair elongation capacity, may not be able to withstand the external stress.
4. Conclusion
The oxidized alginate has been used as a cross linker to prepare casein films. The films show fair water absorption capacity and exhibit high WVTR. There exist strong correlation between the water absorption, moisture permeation and ER of the three films studied. It was observed that these properties increased with the decrease in the amount of cross-linker used. Finally, these films can be used for wounds with high exudates. Use of glycerol lowers the tensile strength to a great extent.
Disclosure statement
All the authors hereby declare that we do not have any conflict of interest about this manuscript.
Acknowledgement
The authors are thankful to AMPRI, Bhopal India for providing facilities for SEM, TGA and XRD analysis.
References
- Ulery BD, Nair LS, Laurencin CT. Biomedical applications of biodegradable polymers. J. Polym. Sci., Part B: Polym. Phys. 2011;49:832–864.10.1002/polb.22259
- Posocco B, Dreussi E, Santa JD, et al. Polysaccharides for the delivery of antitumor drugs. Materials. 2015;8:2569–2615.10.3390/ma8052569
- Shirosaki Y, Hayakawa S, Osaka A, et al. Challenges for nerve repair using chitosan-siloxane hybrid porous scaffolds. BioMed. Res. Int. 2014;2014:1–7.
- Surguchenko VA, Ponomareva AS, Kirsanova LA, et al. The cell-engineered construct of cartilage on the basis of biopolymer hydrogel matrix and human adipose tissue-derived mesenchymal stromal cells (in vitro study). J. Biomed. Mater. Res. Part A. 2015;103:463–470.10.1002/jbm.v103.2
- Archana D, Singh BK, Dutta J, et al. Chitosan-PVP-nano silver oxide wound dressing: in vitro and in vivo evaluation. Int. J. Biol. Macromol. 2015;73:49–57.10.1016/j.ijbiomac.2014.10.055
- Jayakumar R, Prabaharan M, Sudheesh Kumar PT, et al. Biomaterials based on chitin and chitosan in wound dressing applications. Biotechnol. Adv. 2011;29:322–337.10.1016/j.biotechadv.2011.01.005
- Torres FG, Commeaux S, Troncoso OP. Starch-based biomaterials for wound-dressing applications. Starch. 2013;65:543–551.10.1002/star.v65.7/8
- Boateng JS, Pawar HV, Tetteh J. Polyox and carrageenan based composite film dressing containing anti-microbial and anti-inflammatory drugs for effective wound healing. Int. J. Pharm. 2013;441:181–191.10.1016/j.ijpharm.2012.11.045
- Straccia MC, d’Ayala GG, Romano I, et al. Alginate hydrogels coated with chitosan for wound dressing. Mar. Drugs. 2015;13:2890–2908.10.3390/md13052890
- Lee Y, Bae JW, Lee JW, et al. Enzyme-catalyzed in situ forming gelatin hydrogels as bioactive wound dressing: effect of fibroblast delivery on wound healing efficacy. J. Mater. Chem. B. 2014;2:7712–7718.10.1039/C4TB01111B
- Holmes C, Wrobel JS, Eachern MPM, et al. Collagen-based wound dressings for the treatment of diabetes-related foot ulcers: a systematic review. Diabetes Metab. Syndr. Obes. 2013;6:17–29.10.2147/DMSO
- Rose JB, Pacelli S, Haj AJ, et al. Gelatin-based materials in ocular tissue engineering. Materials. 2014;7:3106–3135.10.3390/ma7043106
- Vartiainen J, Harlin A. Crosslinking as an efficient tool for decreasing moisture sensitivity of biobased nanocomposite films. Mater. Sci. Appl. 2011;2:346–354.
- Kennedy-Darling J, Smith LM. Measuring the formaldehyde protein–DNA cross-link reversal rate. Anal. Chem. 2014;86:5678–5681.10.1021/ac501354y
- Yan LP, Wang YJ, Ren L, et al. Genipin-cross-linked collagen/chitosan biomimetic scaffolds for articular cartilage tissue engineering applications. J. Biomed. Mater. Res. Part A. 2010;95A:465–475.10.1002/jbm.a.v95a:2
- Li H, Wu B, Mu C, et al. Concomitant degradation in periodate oxidation of carboxymethyl cellulose. Carbohydr. Polym. 2011;84:881–886.10.1016/j.carbpol.2010.12.026
- Salisu AA, Musa H, Abba H, et al. Preparation and characterization of dialdehyde starch and its cross-linking with copper (II) ion. J. Chem. Pharm. Res. 2013;5:153–158.
- Pascalau V, Gcalsu P, Popescu V, et al. Obtaining and characterizing alginate/k-carrageenan hydrogel cross-linked with adipic dihydrazide. Adv. Mater. Sci. Eng. 2013:1–12, Article ID 380716. Available from http://dx.doi.org/10.1155/2013/380716
- Rottensteiner U, Sarker B, Heusinger D, et al. In vitro and in vivo biocompatibility of alginate dialdehyde/gelatin hydrogels with and without nanoscaled bioactive glass for bone tissue engineering applications. Materials. 2014;7:1957–1974.10.3390/ma7031957
- Ginger MR, Grigor MR. Comparative aspects of milk caseins. Comp. Biochem. Physiol. B: Biochem. Mol. Biol. 1999;124:133–145.10.1016/S0305-0491(99)00110-8
- Maddinedi SB, Mandal BK, Vankayala R, et al. Casein mediated green synthesis and decoration of reduced graphene oxide. Spectrochim. Acta: Part A Mol. Biomol. Spectrosc. 2014;126:227–231.10.1016/j.saa.2014.01.114
- Arrieta MP, Peltzer MA, López J, et al. Functional properties of sodium and calcium caseinate antimicrobial active films containing carvacrol. J. Food Eng. 2014;121:94–101.10.1016/j.jfoodeng.2013.08.015
- Elzoghby AO, El-Fotoh WS, Elgindy NA. Casein-based formulations as promising controlled release drug delivery systems. J. Controlled Release. 2011;153:206–216.10.1016/j.jconrel.2011.02.010
- Abdollahi M, Alboofetileh M, Behrooz R, et al. Reducing water sensitivity of alginate bio-nanocomposite film using cellulose nanoparticles. Int. J. Biol. Macromol. 2013;54:166–173.10.1016/j.ijbiomac.2012.12.016
- Venkatesan J, Bhatnagar I, Manivasagan P, et al. Alginate composites for bone tissue engineering: a review. Int. J. Biol. Macromol. 2015;72:269–281.10.1016/j.ijbiomac.2014.07.008
- Mukhopadhyay P, Chakraborty S, Bhattacharya S, et al. pH-sensitive chitosan/alginate core-shell nanoparticles for efficient and safe oral insulin delivery. Int. J. Biol. Macromol. 2015;72:640–648.10.1016/j.ijbiomac.2014.08.040
- Lee KY, Mooney DJ. Alginate: properties and biomedical applications. Prog. Polym. Sci. 2012;37:106–126.10.1016/j.progpolymsci.2011.06.003
- Ibrahim NA, El-Bisi MK, Ibrahim HM, et al. Triclosan-loaded alginate films for topical medical applications. Int. J. Adv. Res. 2015;3:547–554.
- He X, Tao R, Zhou T, et al. Structure and properties of cotton fabrics treated with functionalized dialdehyde chitosan. Carbohydr. Polym. 2014;103:558–565.10.1016/j.carbpol.2013.12.076
- Manju S, Muraleedharan CV, Rajeev A, et al. Evaluation of alginate dialdehyde cross-linked gelatin hydrogel as a biodegradable sealant for polyester vascular graft. J. Biomed. Mater. Res. Part B: Appl. Biomater. 2011;98B:139–149.10.1002/jbm.b.v98b.1
- Sothornvit R, Krochta JM. Oxygen permeability and mechanical properties of films from hydrolyzed whey protein. J. Agric. Food Chem. 2000;48:3913–3916.10.1021/jf000161m
- Bajpai SK, Chand N, Ahuja S, et al. Curcumin/cellulose micro crystals/chitosan films: water absorption behavior and in vitro cytotoxicity. Int. J. Biol. Macromol. 2015;75:239–247.10.1016/j.ijbiomac.2015.01.038
- Dias AMA, Braga MEM, Seabra IJ, et al. Development of natural-based wound dressings impregnated with bioactive compounds and using supercritical carbon dioxide. Int. J. Pharm. 2011;408:9–19.10.1016/j.ijpharm.2011.01.063
- Thu HE, Zulfakar MH, Fern S. Alginate based bilayer hydrocolloid films as potential slow-release modern wound dressing. Int. J. Pharm. 2012;434:375–383.10.1016/j.ijpharm.2012.05.044
- Sun J, Tan H. Alginate-based biomaterials for regenerative medicine applications. Materials. 2013;6:1285–1309.10.3390/ma6041285
- Jeon O, Samorezov JE, Alsberg E. Single and dual crosslinked oxidized methacrylated alginate/PEG hydrogels for bioadhesive applications. Acta Biomater. 2014;10:47–55.10.1016/j.actbio.2013.09.004
- Bonnaillie LM, Zhang H, Akkurt S, et al. Casein films: the effects of formulation, environmental conditions and the addition of citric pectin on the structure and mechanical properties. Polymers. 2014;6:2018–2036.10.3390/polym6072018
- Gomez CG, Rinaudo M, Villar MA. Oxidation of sodium alginate and characterization of the oxidized derivatives. Carbohydr. Polym. 2007;67:296–304.10.1016/j.carbpol.2006.05.025
- Arrieta MP, Peltzer MA, Garrigós MDC, et al. Structure and mechanical properties of sodium and calcium caseinate edible active films with carvacrol. J. Food Eng. 2013;114:486–494.10.1016/j.jfoodeng.2012.09.002
- Khakalo A, Filpponen I, Johansson LS, et al. Using gelatin protein to facilitate paper thermoformability. React. Funct. Polym. 2014;85:175–184.
- Hoque MS, Benjakul S, Prodpran T. Properties of film from cuttlefish (Sepia pharaonis) skin gelatin incorporated with cinnamon, clove and star anise extracts. Food Hydrocolloids. 2011;25:1085–1097.10.1016/j.foodhyd.2010.10.005
- Mu C, Guo J, Li X, et al. Preparation and properties of dialdehyde carboxymethyl cellulose crosslinked gelatin edible films. Food Hydrocolloids. 2012;27:22–29.10.1016/j.foodhyd.2011.09.005
- Singh A, Bajpai J, Bajpai AK. Investigation of magnetically controlled water intake behavior of Iron Oxide Impregnated Superparamagnetic Casein Nanoparticles (IOICNPs). J. Nanobiotechnol. 2014;12:38–44.10.1186/s12951-014-0038-4
- Singhal R, Tomar RS, Nagpal AK. Effect of cross-linker and initiator concentration on the swelling behaviour and network parameters of superabsorbent hydrogels based on acrylamide and acrylic acid. Int. J. Plast. Technol. 2009;13:22–37.10.1007/s12588-009-0004-4
- Ikeda J, Hasei Y, Yasuda Y, et al. Effect of primary polymer chain rigidity on intramolecular cyclization and intramolecular crosslinking in free-radical crosslinking monomethacrylate/dimethacrylate copolymerizations. J. Appl. Polym. Sci. 2004;94:1086–1093.10.1002/(ISSN)1097-4628
- Schott H. Swelling kinetics of polymers. J. Macromol. Sci. Part B. 1992;31:1–9.10.1080/00222349208215453
- Bueno VB, Bentini R, Catalani LH, et al. Synthesis and swelling behavior of xanthan-based hydrogels. Carbohydr. Polym. 2013;92:1091–1099.10.1016/j.carbpol.2012.10.062
- Katime I, Mendizábal E. Swelling properties of new hydrogels based on the dimethyl amino ethyl acrylate methyl chloride quaternary salt with acrylic acid and 2-methylene butane-1,4-dioic acid monomers in aqueous solutions. Mater. Sci. App. 2010;1:162–167.10.4236/msa.2010.13026
- Bueno VB, Bentini R, Catalani LH, et al. Synthesis and swelling behavior of xanthan-based hydrogels. Carbohydr. Polym. 2013;92:1091–1099.10.1016/j.carbpol.2012.10.062
- Berens AR, Hopfenberg HB. Diffusion and relaxation in glassy polymer powders. 2. Separation of diffusion and relaxation parameters. Polymer. 1978;19:489–496.10.1016/0032-3861(78)90269-0
- Aydinoglu D. Investigation of pH-dependent swelling behavior and kinetic parameters of novel poly(acrylamide-co-acrylic acid) hydrogels with spirulina. e-Polymers. 2015;15:81–93.
- Lamke LO, Nilsson GE, Reithner HL. The evaporative water loss from burns and the water-vapour permeability of grafts and artificial membranes used in the treatment of burns. Burns. 1997;3:159–165.
- Queen D, Gaylor JDS, Evans JH, et al. The preclinical evaluation of the water vapour transmission rate through burn wound dressings. Biomaterials. 1987;8:367–371.10.1016/0142-9612(87)90007-X
- Balakrishnan B, Mohanty M, Umashankar PR, et al. Evaluation of an in situ forming hydrogel wound dressing based on oxidized alginate and gelatin. Biomaterials. 2005;26:6335–6342.10.1016/j.biomaterials.2005.04.012
- Devi MP, Sastry TP, Meignanalakshmi S. Preparation and characterization of fibrin-chitosan composite and it’s in vivo studies. J. Pharm. 2012;2:21–32.
- Sasikala L, Durai B, Rathinamoorthy R, et al. Manuka honey loaded chitosan hydrogel films for wound dressing applications. Int. J. PharmTech. Res. 2013;5:1774–1785.
- Shekarabi AS, Oromiehie AR, Vaziri A, et al. Investigation of the effect of nanoclay on the properties of quince seed mucilage edible films. Indian J. Fundam. Appl. Life Sci. 2014:2:1241–1248.
- Shahabi-Ghahfarrokhi I, Khodaiyan F, Mousavi M, et al. Preparation of UV-protective kefiran/nano-ZnO nanocomposites: physical and mechanical properties. Int. J. Biol. Macromol. 2015;72:41–46.10.1016/j.ijbiomac.2014.07.047
- Shojaee M, Navaee F, Firoozinezhad J, et al. Fabrication and characterization of ovalbumin films for wound dressing applications. Mater. Sci. Eng., C. 2015;48:158–164.10.1016/j.msec.2014.11.063