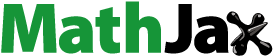
ABSTRACT
Novel monomer, N, N’-bis(acryloyl) cystinamide (NBACA), was designed and synthesized with L-cystine as row material. By using this NBACA both as the monomer and crosslinker, reduction-sensitive nanohydrogel was prepared in ethanol via distillation–precipitation polymerization. The obtained nanohydrogel can provide a relatively hydrophobic environment and hydrogen-bonding sites inside the gel; therefore, it is suitable for loading hydrophobic drug. When paclitaxel that possess poor water-solubility was used as a model drug, the nanohydrogel represented a high drug-loading capacity, and dispersed well in aqueous solutions. Furthermore, the disulfide-group-containing nanohydrogel exhibited good reduction-sensitive drug-release behavior. The nanohydrogel biodegraded rapidly in a reducing environment, and released approximately 80% of the PTX within 24 h. Cytotoxicity assays showed that the PTX-loaded nanohydrogel exhibited high cytotoxicity against MCF-7 breast cancer cells, while blank nanohydrogels displayed a negligible cytotoxicity.
1. Introduction
Hydrogels have been supposed outstanding candidates as one of the promising carriers in biomedical area due to their unique structure and properties. As cross-linked polymers, the hydrogels can absorb, swell and retain considerable amounts of aqueous fluid through its three–dimensional network [Citation1–3]. In particular, nanohydrogels with a hydrodynamic diameter in the sub-micron range exhibit high guest–molecular loading capacity and excellent biocompatibility with low side effects, rendering them adaptive for various applications, such as bioimaging and drug delivery systems [Citation4–7]. Moreover, the nanohydrogels can be designed rationally to respond various external stimuli including pH, temperature, light, enzymatic action, and redox gradients, thus enabling environmentally sensitive drug release [Citation8–12].
Among these stimuli-responsive nanohydrogels, reduction-sensitive nanohydrogels have received increasing attention, and widely used in targeted cancer therapy due to the difference in redox potential between normal cells and cancer cells [Citation13,Citation14]. In general, disulfide bonds are employed to achieve a reduction-triggered drug release, since it can be biodegraded responding to reduction signal molecule such as glutathione [Citation15,Citation16]. So far, several reduction-sensitive nanohydrogels have been reported, in which the monomer or backbone structure was derived from hydrophilic component including methacrylic acid [Citation17], dextrin [Citation18], chitosan [Citation19], alginate [Citation20], hyaluronic acid [Citation21], sorbitan [Citation22], etc. We previously reported N, N’-dimethacryloylcystine crosslinked nanohydrogels [Citation23]. Therefore, these nanohydrogels are preferred to carrying hydrophilic drug such as cationic doxorubicin (DOX). However, most of the drugs are hydrophobic and poorly soluble in water. Thus, the introduction of hydrophobic segments at the nanohydrogels is a useful approach, although reduction-sensitive nanohydrogels carrying hydrophobic drugs reported previously are limited [Citation24–27].
In this study, a novel N, N’-bis(acryloyl) cystinamide (NBACA), was designed and synthesized with L-cystine as row material. By using this NBACA both as the monomer and crosslinker, reduction-sensitive nanohydrogel was prepared for hydrophobic drug, paclitaxel (PTX). Although PTX is one of the most successful anti-cancer drugs [Citation28], the water solubility is very poor (0.3 μg/mL) due to many hydrophobic group in the molecule [Citation29,Citation30]. The reduction-sensitive nanohydrogels developed in this study not only can create a relatively hydrophobic environment, but also can provide hydrogen-bonding sites for the PTX owing to its electroneutral amide group and polyethylene backbone inside the gel. Besides, a facile one-step synthesis of nanohydrogels in ethanol was achieved by using distillation–precipitation polymerization (D-P polymerization). Comparing with other methods, the D-P polymerization does not need additional surfactant, excipients or stabilizers and the process is easy to scale up [Citation31]. Furthermore, the disulfide group inside the nanohydrogels can degrade in reducing environments; hence, a redox-triggered delivery of PTX is also expected.
2. Experimental
2.1. Materials
L-cystine was purchased from Dipper Biotechnology Co., Ltd. (Shanghai, China). Acrylic chloride, aqueous ammonia (28 wt%), paclitaxel (PTX), glutathione (GSH), 2,2-Azobisisobutyronitrile (AIBN), and dithiothreitol (DTT) were purchased from Sun Chemical Technology (Shanghai, China) Co., Ltd. The AIBN was used after recrystallization in ethanol. Methanol, tetrahydrofuran (THF) and diethyl ether were purchased from Hengxing Chemical Preparation Co., Ltd (Tianjin, China). Triethylamine and thionyl chloride were purchased from Damao Chemical Reagent Factory (Tianjin, China). Methanol and THF were dried with CaH2 and distillated before use.
2.1.1. Measurements
The Fourier transforms infrared (FTIR) spectra of NBACA monomer, NBACA nanohydrogel and drug-loaded NBACA nanohydrogel (PTX@nanohydrogels) were performed on the Nicolet IS10 spectrometer. 1H NMR (600 MHz) and 13C NMR (150 MHz) spectra were obtained with a Bruker Advance spectrometer. The morphology of the nanohydrogels was characterized by using scanning electron microscopy (SEM; Hitachi S-3400 N). The hydrodynamic diameter and size distribution of the nanohydrogels were measured by dynamic laser light scattering (DLS; Malvern, Zetasizer Nano ZS90) at a concentration of 1 mg/mL in ultrapure water. Transmittance of the nanohydrogels suspension and the amount of the drug in ethanol were measured by an UV-visible spectrophotometer (SHIMADZU, UV-2700).
2.2. Synthesis of N, N’-bis(acryloyl) cystinamide monomer
The N, N’- bis(acryloyl) cystinamide (NBACA) was synthesized as follows: Firstly, L-cystine (10.0 g, 41.6 mmol) and thionyl chloride (8 mL, 110.3 mmol) were slowly added into anhydrous methanol (150 mL, 3.7 mol) at 0°C, followed by returning to room temperature. The solution was kept refluxing for 6 h at 60°C, and then evaporated to remove excess methanol. The resulting crude product was washed and reprecipitated from methanol/diethyl ether (1/8, v/v) to give L-cystine dimethyl ester dihydrochloride as white solid (yield: 92.8%). Next, dry L-cystine dimethyl ester dihydrochloride (6.0 g, 17.6 mmol) was dissolved in anhydrous THF (50 mL) followed by the addition of triethylamine (8 mL, 57.6 mmol). Subsequently, acryloyl chloride (5 mL, 61.8 mmol) was added dropwise to the solution at −10°C. The reaction was continued with stirring for 6 h at room temperature. After the evaporation, the resulting precipitate was dissolved in CH2Cl2 (100 mL) and thoroughly washed with distilled water (30 mL), 5% NaHCO3 (30 mL) and saturated NaCl (30 mL) for three times, respectively. The organic phase was dried with anhydrous Na2SO4, and then filtered followed by the evaporation. The resulting crude product was recrystallized from acetone/petroleum ether to afford N, N’- bis(acryloyl) cystine dimethyl ester as a white solid (yield: 38.0%). Finally, N, N’-bis(acryloyl) cystine dimethyl ester (374 mg, 0.99 mmol) and 28% aqueous ammonia (3 mL) were mixed and reacted with stirring for 6 h at 0°C. The reaction solution was evaporated and then dried in vacuum at 40°C. The crude product was dissolved in methanol followed by the filtration. After evaporation of the filtrate, the residue was dried to afford the NBACA monomer as a white solid (yield: 67.9%). 1H NMR (600 MHz, DMSO-d6) δ 8.42 (d, J = 8.3 Hz, 1H, -NH-C-), 7.55 (s, 1H, -C-NH2), 7.19 (s, 1H, -C-NH2), 6.31 (dd, J = 17.1, 10.2 Hz, 1H, -CH = C-), 6.10 (dd, J = 17.1, 2.1 Hz, 1H, -C = CH2), 5.61 (dd, J = 10.2, 2.1 Hz, 1H, -C = CH2), 4.59 (td, J = 8.7, 5.1 Hz, 1H, -CHCO-), 3.14 (dd, J = 13.4, 5.0 Hz, 1H, -SSCH2-), 2.90 (dd, J = 13.4, 9.0 Hz, 1H, -SSCH2-). 13C NMR (150 MHz, DMSO-d6) δ 172.0, 164.9, 131.8, 126.1, 52.2, 41.2.
2.3. Synthesis of NBACA nanohydrogels
NBACA nanohydrogels were synthesized via distillation–precipitation polymerization method. The typical synthesis procedure was as follows: N, N’-bis(acryloyl) cystinamide (75 mg, 0.22 mmol) and AIBN (11 mg, 0.067 mmol) were dissolved in anhydrous ethanol (40 mL) with ultrasonic agitation for 10 min. The reaction mixture was heated to 83°C (the temperature of water bath) and kept for 30 min. The reaction was stopped until about 18 mL of ethanol was distilled from the reaction mixture. After repeating centrifugation (8000 rpm, 10 min) and redispersion (ethanol, 10 mL) cycle for three times, the precipitates were lyophilized to afford nanohydrogels as white powders.
2.4. Reductive degradation of nanohydrogels
The reductive degradation of NBACA nanohydrogels was monitored by measuring the transmittance change of the nanohydrogel suspension. Lyophilized nanohydrogels (4 mg) were dispersed in 10 mL of phosphate buffer solution (PBS, pH = 7.4) in the presence or absence of reductant (GSH or DTT, 10 mM) respectively. Subsequently, the mixture was placed in a shaking bed (37°C, 150 rpm). At defined time points, 80 µL of the solution was collected and the transmittance was measured by UV-visible spectrophotometer at 630 nm.
2.5. Drug loading assay
PTX was used to prepare drug-loaded nanohydrogels. Typically, 6 mg of PTX and 10 mg of lyophilized empty nanohydrogels were dispersed and ultrasonicated in 10 mL of ethanol for 10 min, and then continuously stirred for 24 h at room temperature. After centrifugating (8000 rpm, 10 min) and removing the supernatant, the resultant precipitate was then lyophilized to afford PTX-loaded NBACA nanohydrogels. To determine the drug-loading content in the nanohydrogels, 1.5 mg of lyophilized PTX-loaded NBACA nanohydrogels were dispersed in 14 mL of ethanol and then stirred for 24 h. Subsequently, the suspension was centrifuged (8000 rpm, 10 min), and the amount of PTX in the supernatant was determined by UV-visible spectrophotometer at 227 nm using a standard curve. The drug loading capacity (DLC) and drug encapsulation efficiency (EE) were calculated by following equations:
2.6. In vitro drug release
The PTX-release behavior was evaluated under different reducing conditions by dialysis method using a dialysis bag (MWCO = 3500) [Citation32]. Typically, 10 mg PTX-loaded nanohydrogels were dispersed in 10 mL PBS (pH = 7.4) buffer containing 1.2 mM of sodium dodecyl sulfate. The sample was then transferred into a dialysis bag followed by dialyzing against 150 mL of the PBS buffer with or without the presence of GSH or DTT (10 mM) and gently shaken (160 rpm) at 37°C. At a predetermined time, 2 mL of the solution was removed and the volume of reservoir was held constant by adding 2 mL fresh buffer medium. The sample solution was extracted with 10 mL CH2Cl2 for three times. The CH2Cl2 phase was collected, and the solvent was evaporated under reduced pressure. Subsequently, the residue was redissolved with 1 mL ethanol, and the amount of the released PTX was measured three times by UV-visible spectrophotometer at 227 nm.
2.7. Cell culture
The MCF-7 cells were routinely cultured in Dulbecco’s Modified Eagle’s Medium (high glucose) supplemented with 10% fetal bovine serum (FBS) and maintained at 37°C in a humidified incubator with 5% CO2.
2.8. Cytotoxicity assay
MCF-7 cancer cells were seeded into 96-well plates at a density of 3000 cells/well. After overnight incubation, the cells were incubated in the presence of different samples for 72 h at 37°C. Blank NBACA nanohydrogels and PTX-loaded nanohydrogels and free PTX were diluted with culture medium, respectively, to obtain various concentrations of samples. After incubation, cell viability was investigated by a standard MTT (3-(4,5-Dimethylthiazol-2-yl)-2,5-diphenyltetrazoliumbromide) assay.
3. Results and discussion
3.1. Synthesis and characterization of NBACA monomer
The N, N’-bis(acryloyl)cystinamide (NBACA) monomer was synthesized in three steps (). Firstly, L-cystine dimethyl ester dihydrochloride was prepared by the esterification of L-cystine and methanol in the presence of thionyl chloride. In the second step, N, N’-bis(acryloyl) cystine dimethyl ester was synthesized from the amidation reaction of acryloyl chloride with the L-cystine dimethyl ester dihydrochloride in the presence of triethylamine. Finally, NBACA was synthesized by ammonolysis of N, N’-bis(acryloyl) cystine dimethyl ester in 28% aqueous ammonia.
The chemical structure of NBACA monomer was confirmed by FTIR, 1H NMR and 13C NMR. As shown in , the typical amide band (1656.4 cm−1) was observed clearly. Furthermore, the FTIR spectra also showed peaks at 1625.8 cm−1, 1311.5 cm−1, 961.4 cm−1 and 803.9 cm−1 due to C = C stretching, bending vibration of C-H on the C = C, respectively. 1H NMR spectrum of N, N’-bis(acryloyl) cystinamide is shown in . The signals of N-H protons in amide groups were observed at 8.4, 7.5 and 7.2 ppm, and the characteristic signals of C-H protons in the C = C bonds were observed at 6.3, 6.1 and 5.6 ppm. The signals of the C-H protons in methylene at 4.6 ppm and the methylene C-H protons at 3.1 and 2.9 ppm were also observed separately. The 13C NMR spectrum of NBACA monomer showed six different signals corresponding to six nonequivalent carbons (). The signals at 41.2 and 52.2 ppm are assigned to the carbon of methylene and methine, respectively. In addition, signals at 126.1 and 131.8 ppm are due to the two carbons of C = C bonds, and the carbons from amide groups showed the signals at 164.9 and 172.0 ppm.
3.2. Synthesis and characterization of NBACA nanohydrogels
NBACA nanohydrogel was synthesized via D-P polymerization by mixing the NBACA and AIBN in ethanol. The resulting nanohydrogels could be dispersed well in aqueous solution ()). The nanohydrogel was further characterized by using SEM and DLS. As shown in ), the average diameter measured by DLS (361 nm) was larger than that determined by SEM (180 nm), indicating that the NBACA nanohydrogels may swell in aqueous solution. Besides, the chemical structure of nanohydrogels was analyzed by FTIR. As shown in , comparing with the spectrum of NBACA monomer, the amide band (1656.4 cm−1) was retained in that of the nanohydrogels, whereas the absorption peaks from double bonds (δC-H 1311.5 cm−1, νC=C 1625.8 cm−1, δC=C-H 961.4 cm−1, 803.9 cm−1) disappeared. This indicated that NBACA nanohydrogels were successfully prepared by the polymerization of NBACA monomers.
3.3. Reduction-sensitive degradation of NBACA nanohydrogels
The reduction-sensitive behavior of NBACA nanohydrogels was investigated by monitoring transmittance change of nanohydrogel suspension with UV-visible spectroscopy, in which DTT and GSH were used as reductant. As shown in , almost no transmittance change in nanohydrogels suspension was observed under no reductant condition; however, after the addition of DTT, the transmittance of suspension increased about 50% within 15 min, indicated that the nanohydrogels rapidly degraded. Upon the addition of GSH, the transmittance of the nanohydrogels also increased significantly after 2 h, whereas the degradation rate was slower than that in the presence of DTT. This may be due to the difference in steric hindrance between the two compounds, the DTT is more readily accessible to the disulfide bond than GSH.
3.4. Drug-loading studies
In order to evaluate the hydrophobic drug-loading property of the nanohydrogel, PTX was used as a model drug, and the drug loading capacity and entrapment efficiency were determined by UV-vis spectrometer. As the PTX dosage increased from 1 mg to 4 mg, the DLC of the nanohydrogels increased from 1.28% to 24.81%, and the EE increased from 6.49% to 41.06% (). The drug-loading capacity (24.81%) obtained for the NBACA nanohydrogels, is higher than that of some other nanogel carrier [Citation25], indicating the potential of these nanohydrogels as a hydrophobic drug carrier. Probably, the relative hydrophobic environment due to the polyethylene backbone as well as the role of hydrogen bonding donor/acceptor of electroneutral amide group inside the nanohydrogels enabled better drug loading content. In addition, FTIR spectrum of the drug-loaded nanohydrogel was also investigated. As shown in , all characteristic bands of blank nanohydrogel could be observed in the PTX-loaded nanohydrogel. This result indicated that there was no chemical interaction between PTX and nanohydrogel. In contrast, in the spectrum of PTX-loaded nanohydrogel, additional weak characteristic peaks due to ester group (1243.8 cm-1 and 1072.7 cm-1) and C-O stretching peak due to hydroxyl group (1025.5 cm−1, 984.0 cm−1) were found. These peaks are from the PTX, but not from the nanohydrogel, indicating that PTX is successfully encapsulated in nanohydrogel.
Table 1. Drug loading capacity (DLC) and encapsulation efficiency (EE) of PTX for NBACA nanohydrogels
3.5. Release of drug from nanohydrogels
Reduction-triggered release of PTX from NBACA nanohydrogels was also investigated. As shown in , approximately 80% of the PTX was released from the nanohydrogels within 24 h under a reducing environment (GSH or DTT). This result suggested that the release of PTX from the nanohydrogel was not only ascribed to cleavage of disulfide bond, but also dependent on its disadsorption from the backbone of nanohydrogels. In contrast, only 25% of the PTX was released under non-reducing conditions, which may be caused by the PTX absorbed on the surface of the nanohydrogel. These results demonstrated that PTX-loaded NBACA nanohydrogels can release the PTX in a reduction-sensitive manner.
3.6. Cytotoxicity assays
MTT assay was performed against MCF-7 breast cancer cells by using blank nanohydrogel, PTX-loaded nanohydrogel. As shown in ), blank nanohydrogels exhibited a negligible cytotoxicity against MCF-7 cells, even at a high dosage, suggesting that the nanohydrogels may be suitable as nontoxic nanocarriers. After treatment with PTX-loaded nanohydrogels, the viability of MCF-7 cells decreased significantly with increasing the concentration of PTX-loaded nanohydrogels. The calculated IC50 value of the PTX-loaded nanohydrogels was 3.78 µg/mL, which similar to that of PTX-loaded heparin-alpha-tocopherol succinate (Hep-cys-TOS) nanoparticles (IC50 = 0.79 µg/mL) [Citation33]. Besides, although higher cytotoxicity of free PTX than PTX-loaded nanohydrogels was observed in low concentration region; however, the cytotoxicity of PTX-loaded nanohydrogels was higher than that of free PTX in high concentration region, suggesting that aggregation of the free PTX may occurred in high concentration region ()), which may due to poor solubility of PTX in aqueous media [Citation29]. The cytotoxicity of the PTX-loaded nanohydrogels may be attributed to the fact that the cancer cells uptake the PTX-loaded NBACA nanohydrogels and then release the drugs in a reduction-sensitive manner. Therefore, NBACA nanohydrogels represent a promising drug nanocarrier due to their great biocompatibility and reduction sensitivity.
4. Conclusion
A novel monomer, N, N’-bis(acryloyl) cystinamide was designed and successfully synthesized via esterification, amidation and aminolysis reactions. By using the N, N’-bis(acryloyl) cystinamide as both a monomer and crosslinking agent, new NBACA nanohydrogels were prepared in ethanol via distillation–precipitation polymerization. The nanohydrogels can provide electroneutral hydrophobic environment and hydrogen bonding sites for hydrophobic drug, thus exhibited a good PTX-loading capacity. Furthermore, the disulfide-bonds-containing nanohydrogel also exhibited promising reduction-responsive drug-release behavior. Cell cytotoxicity results demonstrated that the nanohydrogels showed almost no cytotoxicity, while the PTX-coated nanohydrogels showed strong cytotoxicity in MCF-7 breast cancer cells. Therefore, as a new biodegradable, reduction-sensitive nanocarrier, this type of nanohydrogel could be used for delivering more kinds of hydrophobic drugs as well as PTX.
Acknowledgments
We also thank the program for innovative research team of the Ministry of Education and the program for Liaoning Innovative Research Team at Shenyang Pharmaceutical University.
Disclosure statement
No potential conflict of interest was reported by the author(s).
Additional information
Funding
References
- Hoffman AS. Hydrogels for biomedical applications. Adv Drug Del Rev. 2012;64:18–23.
- Buwalda SJ, Boere KW, Dijkstra PJ, et al. Hydrogels in a historical perspective: from simple networks to smart materials. J Control Release. 2014;190:254–273.
- Duan JJ, Zhang LN. Robust and smart hydrogels based on natural polymers. Chin J Polym Sci. 2017;35:1165–1180.
- Tahara Y, Akiyoshi K. Current advances in self-assembled nanogel delivery systems for immunotherapy. Adv Drug Del Rev. 2015;95:65–76.
- Peppas NA, Hilt JZ, Khademhosseini A, et al. Hydrogels in biology and medicine: from molecular principles to bionanotechnology. Adv Mater. 2006;18:1345–1360.
- Li YL, Maciel D, Rodrigues J, et al. Biodegradable polymer nanogels for drug/nucleic acid delivery. Chem Rev. 2015;115:8564–8608.
- Li D, Van Nostrum CF, Mastrobattista E, et al. Nanogels for intracellular delivery of biotherapeutics. J Control Release. 2017;259:16–28.
- Chacko RT, Ventura J, Zhuang JM, et al. Polymer nanogels: a versatile nanoscopic drug delivery platform. Adv Drug Del Rev. 2012;64:836–851.
- Kabanov AV, Vinogradov SV. Nanogels as pharmaceutical carriers: finite networks of infinite capabilities. Angew Chem Int Ed. 2009;48:5418–5429.
- Motornov M, Roiter Y, Tokarev I, et al. Stimuli-responsive nanoparticles, nanogels and capsules for integrated multifunctional intelligent systems. Prog Polym Sci. 2010;35:174–211.
- Álvarez-Bautista A, Duarte CMM, Mendizábal E, et al. Controlled delivery of drugs through smart pH-sensitive nanohydrogels for anti-cancer therapies: synthesis, drug release and cellular studies. Des Monomers Polym. 2016;19:319–329.
- Hajebi S, Rabiee N, Bagherzadeh M, et al. Stimulus-responsive polymeric nanogels as smart drug delivery systems. Acta Biomater. 2019;92:1–18.
- Ghorbani M, Hamishehkar H. Redox-responsive smart nanogels for intracellular targeting of therapeutic agents: applications and recent advances. J Drug Targeting. 2019;27:408–422.
- Kumar P, Liu B, Behl G, et al. Outlook of synthetic strategies and applications of redox-responsive nanogels in drug delivery. Macromol Biosci. 2019;19. DOI:https://doi.org/10.1002/mabi.201900071
- Deng B, Ma P, Xie Y. Reduction-sensitive polymeric nanocarriers in cancer therapy: a comprehensive review. Nanoscale. 2015;7:12773–12795.
- Lee MH, Sessler JL, Kim JS. Disulfide-based multifunctional conjugates for targeted theranostic drug delivery. Acc Chem Res. 2015;48:2935–2946.
- Pan YJ, Chen YY, Wang DR, et al. Redox/pH dual stimuli-responsive biodegradable nanohydrogels with varying responses to dithiothreitol and glutathione for controlled drug release. Biomaterials. 2012;33:6570–6579.
- Zhang F, Gong S, Wu J, et al. CXCR4-targeted and redox responsive dextrin nanogel for metastatic breast cancer therapy. Biomacromolecules. 2017;18:1793–1802.
- Zuo YJ, Kong M, Mu YZ, et al. Chitosan based nanogels stepwise response to intracellular delivery kinetics for enhanced delivery of doxorubicin. Int J Biol Macromol. 2017;104:157–164.
- Maciel D, Figueira P, Xiao S, et al. Redox-responsive alginate nanogels with enhanced anticancer cytotoxicity. Biomacromolecules. 2013;14:3140–3146.
- Yang CC, Li C, Zhang P, et al. Redox responsive hyaluronic acid nanogels for treating RHAMM (CD168) over-expressive cancer, both primary and metastatic tumors. Theranostics. 2017;7:1719–1734.
- Khoee S, Yousefalizadeh G, Kavand A. Preparation of dual-targeted redox-responsive nanogels based on pegylated sorbitan for targeted and antitumor drug delivery. Eur Polym J. 2017;95:448–461.
- Jiang GF, Weng JP, Kong LP, et al. Reduction-sensitive N,N′-dimethacryloylcystine nanohydrogel for triggered drug release. Mater Lett. 2017;189:122–125.
- Abdullah AN, Lee H, Lee YS, et al. Development of disulfide core-crosslinked pluronic nanoparticles as an effective anticancer-drug-delivery system. Macromol Biosci. 2011;11:1264–1271.
- Perez E, Fernandez A, Olmo R, et al. pH and glutathion-responsive hydrogel for localized delivery of paclitaxel. Colloids Surf B Biointerfaces. 2014;116:247–256.
- Qiao ZY, Zhang R, Du FS, et al. Multi-responsive nanogels containing motifs of ortho ester, oligo(ethylene glycol) and disulfide linkage as carriers of hydrophobic anti-cancer drugs. J Control Release. 2011;152:57–66.
- Ryu JH, Jiwpanich S, Chacko R, et al. Surface-functionalizable polymer nanogels with facile hydrophobic guest encapsulation capabilities. J Am Chem Soc. 2010;132:8246–8247.
- Sofias AM, Dunne M, Storm G, et al. The battle of “nano” paclitaxel. Adv Drug Deliv Rev. 2017;122:20–30.
- Ezrahi S, Aserin A, Garti N. Basic principles of drug delivery systems - the case of paclitaxel. Adv Colloid Interface Sci. 2019;263:95–130.
- Wang FH, Porter M, Konstantopoulos A, et al. Preclinical development of drug delivery systems for paclitaxel-based cancer chemotherapy. J Control Release. 2017;267:100–118.
- Feng B, Yang XL, Huang WQ. Synthesis of narrow or monodisperse poly(divinylbenzene) microspheres by distillation-precipitation polymerization. Macromolecules. 2004;37:9746–9752.
- Jin S, Wan JX, Meng LZ, et al. Biodegradation and toxicity of protease/redox/ph stimuli-responsive PEGlated PMAA nanohydrogels for targeting drug delivery. ACS Appl Mater Interfaces. 2015;7:19843–19852.
- Yang XY, Cai XQ, Yu AH, et al. Redox-sensitive self -assembled nanoparticles based on alpha-tocopherol succinate-modified heparin for intracellular delivery of paclitaxel. J Colloid Interface Sci. 2017;496:311–326.