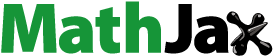
ABSTRACT
Although hyperbranched polysiloxanes have been extensively studied, they have limited practical applications because of their low glass transition temperatures. In this study, we synthesized benzocyclobutene-functionalized hyperbranched polysiloxane (HB-BCB) via the Piers-Rubinsztajn reaction. The synthesized material was cured and crosslinking occurred at temperatures greater than 200 °C, forming a low-k thermoset resin with high thermostability. The structure of the resin was characterized using nuclear magnetic resonance (NMR) spectroscopy, viz. 1H NMR and 13C NMR spectroscopy. 29Si NMR spectroscopy was used to calculate the degree of branching. Differential scanning calorimetry, dynamic mechanical analysis, and thermogravimetric analysis revealed that the cured resin possesses good high-temperature mechanical properties and exhibits a high thermal decomposition temperature (Td5 = 512 °C). In addition, the cured resin has a low dielectric constant (k = 2.70 at 1 MHz) and low dissipation factor (2.13 × 10−3 at 1 MHz). Thus, the prepared resin can function as a low-k material with excellent high-temperature performance. These findings indicate that the performance of crosslinked siloxane is significantly attributed to the introduction of BCB groups and the formation of the highly crosslinked structure.
1. Introduction
Signal delay of interconnects is the main obstacle in the development of integrated microelectronic devices with high density. To overcome this limitation, it is necessary to develop high-performance materials with low dielectric constants, i.e., low-k materials [Citation1]. An ideal low-k material needs to exhibit a low dielectric dissipation factor, good thermal stability, and low coefficient of thermal expansion. Various low-k organic materials have been developed, such as polyimides [Citation2–5], organic siloxanes [Citation6], polybenzoxazoles [Citation7,Citation8], epoxy resins [Citation9,Citation10], etc. Among them, benzocyclobutene (BCB) resins are considered to be high-performance materials with a wide-range of potential applications [Citation3,Citation11–15]. The ring-opening reaction of cyclobutene in BCB, followed by crosslinking and polymerization forms a highly crosslinked network structure [Citation16]. The low polarity of this results in reduced dielectric constant and water absorption. In addition, the high extent of crosslinking enhances the thermal stability and increases the glass transition temperature of BCB-based resins [Citation17]. Moreover, BCB can be thermally-cured at relatively low temperatures without the formation of byproducts.
Polysiloxanes are another important class of insulating materials that exhibit good thermal and chemical stabilities. However, their low glass transition temperature (Tg) limits their application as high-performance dielectric materials. To overcome this limitation, crosslinkable groups such as BCB can be introduced into the polysiloxane network to increase Tg by thermal curing of BCB. There have been reported many methods to incorporate BCB into siloxane polymers, such as Heck reaction [Citation17,Citation18], hydrosilation reaction [Citation19], and hydrolysis and condensation reaction of BCB functionalized oxosilanes [Citation12,Citation20]. The Piers-Rubinsztajn (P-R) reaction has attracted considerable attention as an effective and facile approach for the synthesis of polysiloxanes [Citation21–24]. Unlike other reactions which require precious metal catalysts, such as platinum in the hydrosilylation reaction and palladium in the Heck reaction, the P-R reaction is catalyzed by tris(pentafluorophenyl)borane [B(C6F5)3], a weak Lewis acid [Citation25]. In addition, this reaction is rapid even under mild conditions, and leads to the release of an alkane gas, which aids in the monitoring the reaction progress. The generation of Si-OH groups is also prevented in the P-R reaction; thus, the product formed has a low water absorption rate [Citation6]. Recently, we have prepared a linear oligomer BCB functionalized polysiloxane by P-R reaction of BCB functionalized dimethoxysilane monomer (A2) with silphenylene/silbiphenylene hydrosilanes (B2) [Citation26]. After cured, a linear oligomer showed low dielectric constants at 2.76 and 2.78 at 1 MHz. the synthesis of the monomers A2 and B2 were complicated and the dielectric constant can be further decreased.
Hyperbranched polymers usually exhibit different properties as compared to linear polymers because of their unique structure, in which numerous reactive functional groups are concentrated at the chain terminals [Citation27]. Compared with a linear polymer, a hyperbranched polymer presumably possesses a larger free volume, owing to the formation of porous voids between two different chains in the structure. Thus, formation of a hyperbranched structure could be an effective approach to reduce the dielectric constant of polymers [Citation7,Citation28,Citation29]. Based on this presumption, we have synthesized a BCB-functionalized hyperbranched polysiloxane (HB-BCB) by performing the P-R reaction with commercial diethoxymethylsilane (AB2 type monomer) to construct a hyperbranched polysiloxane, and then substituting the remaining Si-OEt groups by the Si-H-functionalized BCB groups in a cascade reaction way. It’s a facile and convenient method to prepare the BCB functionalized siloxane polymer. After curing, the material exhibited a low dielectric constant of 2.70 at 1 MHz, good high-temperature performance, and high thermostability.
2. Experimental sections
2.1. Materials and reagents
4-Br-BCB (97%) was purchased from Chem-target Technologies Co., Ltd, China. Dry toluene was obtained after refluxing and distilling toluene with sodium and benzophenone. Benzocyclobutene-4-yl-dimethylsilane (BCBSiH) was synthesized according to a previously reported procedure [Citation19]. Diethoxymethylsilane (DEMS 98%) was purchased from Sun Chemical Technology Co., Ltd. (Shanghai). Neutral alumina was used as received. Tris(pentafluorophenyl)borane, B(C6F5)3, was purchased from Tokyo Chemical Industry Co., Ltd.
2.2. Characterization method
Nuclear magnetic resonance (NMR) spectroscopy, viz. 1H NMR, 13C NMR, and 29Si NMR was conducted using a Bruker DRX-500 spectrometer with tetramethylsilane (TMS) as the reference and CDCl3 as the solvent. Fourier transform infrared (FT-IR) spectra were recorded on a Bruker VERTEX 70 FT-IR spectrophotometer. Differential scanning calorimetry (DSC; TA Q200 calorimeter) and thermogravimetric analysis (TGA; TA Instruments Q500) were performed at a heating rate of 10 °Cmin−1 under a N2 atmosphere. Dynamic mechanical analysis (DMA; TA Instruments Q800) was conducted using the three-point bending mode at a heating rate of 3 °Cmin−1 and a test frequency of 1 Hz. The dielectric properties were measured by the parallel-plate capacitor method using a Keysight E4980A precision LCR meter and Keysight 16451B as a test fixture at room temperature. The molecular weight was determined using gel permeation chromatography (GPC; Waters 1515 system) equipped with a refractive index (RI) detector using tetrahydrofuran (THF) as solvent at a flow rate of 1.0 mL min−1. Calibration was accomplished with polystyrene standards.
2.3. Preparation of HB-BCB
In a 25 mL three-necked round-bottomed flask equipped with a magnetic stirring bar, DEMS (1.34 g, 0.01 mol), and toluene (5 mL, 4.28 g) were added under nitrogen atmosphere using a three-way stopcock, and the reactor was placed in an ice-water bath. While stirring, B(C6F5)3 (4 mg, 0.0078 mmol) in toluene was injected into the reaction mixture using a syringe. The ice-water bath was removed to increase the reaction rate. The generation of bubbles indicated the initiation of the P-R reaction. After the generation of bubbles stopped, BCBSiH (1.62 g, 0.01 mol) was slowly added to the reaction mixture using a syringe. The resulting solution was stirred for 1 h. The reaction was terminated by adding Al2O3 to deactivate the catalyst. The mixture was stirred for 10 min and filtered using a filter paper and an ultrafiltration membrane (0.45 μm). The solvent, toluene, was removed by rotary evaporation, and HB-BCB was obtained as a colorless viscous liquid. 1H-NMR (CDCl3, 500 MHz, ppm): 0.02–0.10 (3 H), 0.32–0.35 (6 H), 3.12 (4 H), 7.00–7.07 (1H), 7.24–7.25 (1H), 7.39–7.42 (1H). 13C-NMR (CDCl3, ppm): −0.25–0.57, 3.26–3.58, 32.22–32.31-32.37, 124.17–124.24, 129.27, 133.82, 139.70–140.10-140.53, 147.76–147.89, 149.70–149.80. 29Si-NMR (ppm): 0.18–1.57, 56.04–59.42, 63.94–66.78. GPC: MW = 1555 g/mol, MW/MN = 1.25.
2.4. Curing of the resin
To prepare the bulk polymer, the glass mold with HB-BCB was degassed in a vacuum oven at 130 °C for 0.5 h, and then heated sequentially at 180 °C /1 h, 210 °C /1 h, 230 °C /1 h, 250 °C /1 h, 260 °C/1 h, and 290 °C /0.5 h under a nitrogen atmosphere. After cooling to 25 °C, the thermoset was removed from the glass mold and polished to an appropriate size for DMA and TGA analyses ().
3. Results and discussion
3.1. Preparation and characterization of HB-BCB
shows the synthetic approach used to prepare HB-BCB. DEMS was used as a precursor for the P-R reaction, and a hyperbranched polysiloxane (HB-DEMS) was produced (step 1). Then, BCBSiH was added to substitute the ethoxy groups (-O-Et) with the BCB functional groups, which enabled the crosslinking of the oligomer (step 2). As measured by GPC, HB-BCB showed a low average molecular weight of 1555 g/mol; thus, it is considered to be an oligomer.
The 1H and 13C NMR spectra are shown in . The peaks corresponding to Si-H proton (4.5 ppm) and Si-OCH2CH3 protons (3.81 and 1.24 ppm) are almost disappeared in the 1H-NMR spectrum of HB-BCB, indicating that the P-R reaction reached completion. In addition, the integration traces of peak 1 and peak 3 have a ratio of 3:1, which is in accordance with the stoichiometric ratio of DEMS and BCBSiH in the reaction. However, the formation of a hyperbranched structure cannot be verified solely on the basis of the 1H and 13C NMR spectra because the branched chains are formed by the Si–O linkages.
Thus, the 29Si-NMR spectrum of the intermediate, HB-DEMS, is used to characterize the hyperbranched structure (). The signals corresponding to the dendritic units (D), linear units (L), and terminal units (T) of the hyperbranched polymer can be clearly observed in the 29Si-NMR spectrum of HB-DEMS. It has been reported that for silicon atoms connected to the same number of oxygen atoms, the introduction of -OEt groups increases the chemical shift value by approximately 7–10 ppm, indicating that larger chemical shift values correspond to Si atoms with more -OEt groups [Citation30]. On the basis of this discussion, the peaks in the 29Si-NMR spectrum of HB-DEMS were easily assigned. The degree of branching (DB) was calculated by the following equation:
where D, L, and T are the relative amounts of D, L, and T units, respectively. These units can be evaluated from the ratio of the integral intensities of the peaks in the 29Si-NMR spectrum [Citation31]. Using this Equationequation (1)(1)
(1) , the DB of HB-DEMS was found to be approximately 0.45. In the 29Si-NMR spectra of HB-BCB, there was no peak corresponding to the T unit. In addition, the intensity of the peak attributed to the L unit reduced significantly, possibly due to the substitution of the O-Et groups by the – O-SiMe2BCB groups. These findings suggest that most of the core silicon atoms of the T and L units in HB-DEMS are converted to the D units in HB-BCB (). A new peak is observed at approximately −2 ppm, and it can be ascribed to – O-SiBCB group. Since the hyperbranched framework of HB-DEMS remains unchanged after the substitution reaction, it is reasonable to assume that the DB of HB-BCB is the same as that of HB-DEMS (0.45).
3.2. Curing behavior
The curing behavior of the synthesized HB-BCB is studied using DSC (). The crosslinking process is exothermic and begins at a temperature of 200 °C. The peak exotherm is observed at 251 °C and the crosslinking reaction reaches completion at 340 °C. These findings are in accordance with those reported for other BCB-based polymers. This curing behavior can be ascribed to the ring-opening of the four-membered ring in BCB, leading to the formation of o-quinodimethane intermediates, along with the crosslinking of the ring-opened BCB units. No significant glass transition was observed in the DSC curve of cured HB-BCB because it is highly crosslinked, and the movement of its segments is limited.
The formation of the highly crosslinked HB-BCB was also verified using FT-IR spectroscopy (). The following discussion is based on the theoretical FT-IR data obtained by Ocola et al. [Citation32]. A comparison of the FT-IR spectra reveals that the peaks at 1432 cm−1 and 2830 cm−1, which are assigned to the CH2 deformation and its overtone band, are not present in the spectrum of cured HB-BCB. In addition, the intensity of the peak at 2928 cm−1, which is ascribed to the CH2 symmetrical stretching vibration of BCB, significantly decreases after curing. The peak corresponding to the aromatic C-C stretching in BCB shifts from 1465 cm−1 to 1495 cm−1 after curing because the ring strain caused by the four-member ring is released after the ring-opening and crosslinking reactions. Moreover, in the FT-IR spectrum of the cured resin, there is no peak at 880 cm−1, which may correspond to a combination band related to the four-member ring. These findings verify the ring-opening of cyclobutene in BCB, which leads to the formation of a highly crosslinked network structure.
3.3. Properties of the cured resin
After curing, the oligomer HB-BCB as viscous liquid converted into yellow transparent rigid plastic. Thermal stability of the cured resin is measered by TGA and the TGA curve is shown in . It reveals a high thermal decomposition temperature at 5% weight loss (Td5 = 512 °C) with a high char yield of 61% at 800 °C. The excellent thermal stability of the cured HB-BCB may be attributed to the siloxane framework and the highly crosslinked network structure constructed by the BCB group.
The DSC curve of the cured HB-BCB () show is smooth with no peak. Therefore, it is difficult to detect the glass transition temperature (Tg) for crosslinked resins using only DSC. Thus, DMA was used to measure the thermomechanical properties of the cured HB-BCB (). The cured HB-BCB exhibits a high initial storage modulus of 2.4 GPa, attributing to the curing of HB-BCB and the formation of the highly crosslinked structure. The storage modulus decreases with increase in temperature: 0.78 GPa at 200 °C and 0.58 GPa at 350 °C. The tan δ curve of the cured HB-BCB exhibits a maximum at 178 °C with a damping coefficient of 0.09, and the corresponding storage modulus is 0.94 GPa. The properties of cured HB-BCB differ from those of ordinary polymers that have a low storage modulus (<50 MPa) in the rubber state and a large damping coefficient at the Tg [Citation26] . Since the hyperbranched siloxane framework is surrounded by the rigid BCB crosslinked network, it is reasonable to assume that the tan δ peak may be regarded as a synergistic secondary relaxation of the siloxane structure confined by the highly crosslinked structure.
The cured HB-BCB exhibits a low relative dielectric constant of 2.70 and low dissipation factor of 2.75 × 10−3 at 1 MHz (), which suggests that this material shows relatively good dielectric property, which may have potential applications in the field of microelectronics and packaging.
4. Conclusions
The HB-BCB resin, consisting of a hyperbranched polysiloxane framework functionalized with BCB, was successfully synthesized via the P-R reaction in a cascade reaction way. It’s a facile and convenient method to prepare the BCB functionalized siloxane polymer. The obtained oligomer was cured and it was found that crosslinking occurred at temperatures greater than 200 °C. The hyperbranched structure was verified using NMR and FT-IR spectroscopy. The cured resin possessed a low dielectric constant (k = 2.70), low dissipation factor (2.13 × 10−3 at 1 MHz), good high-temperature mechanical properties, and an extremely high thermal decomposition temperature (Td5 = 512 °C). The high performance of the cured resin can be ascribed to the introduction of BCB groups to form the highly crosslinked and low-polarity structure. In addition, the findings of this study demonstrate that the prepared resin may function as an ideal low-k material with excellent high-temperature performance. Thus, the HB-BCB resin can be potentially applied in the microelectronics industry.
Disclosure statement
No potential conflict of interest was reported by the author(s).
Additional information
Funding
References
- Volksen W, Miller RD, Dubois G. Low dielectric constant materials. Chem Rev. 2010;110(1):56–110.
- Liu YW, Qian C, Qu LJ, et al. A bulk dielectric polymer film with intrinsic ultralow dielectric constant and outstanding comprehensive properties. Chem Mater. 2015;27(19):6543–6549.
- Yang J, Cheng Y, Jin Y, et al. Synthesis and characterization of organo-soluble and photosensitive benzocyclobutene-terminated imides. Eur Polym J. 2013;49(6):1642–1653.
- Zuo H, Gan F, Dong J, et al. Highly transparent and colorless polyimide film with low dielectric constant by introducing meta-substituted structure and Trifluoromethyl groups. Chinese J Polym Sci. 2021;39(4):455–464.
- Zhang M, Liu W, Gao X, et al. Preparation and characterization of Semi-Alicyclic polyimides containing Trifluoromethyl groups for optoelectronic application. Polymers-Basel. 2020;12(7):1532.
- Wang J, Zhou J, Jin K, et al. A new fluorinated polysiloxane with good optical properties and low dielectric constant at high frequency based on easily available Tetraethoxysilane (TEOS). Macromolecules. 2017;50(23):9394–9402.
- Wang Y, Yu JR, Zhu J, et al. Hyperbranched Polybenzoxazoles incorporated polybenzoxazoles for High-Performance and Low-K materials. J Polym Sci Pol Chem. 2016;54(11):1623–1632.
- Zhang K, Han L, Froimowicz P, et al. A smart latent catalyst containing o-Trifluoroacetamide Functional benzoxazine: precursor for low temperature formation of very high performance polybenzoxazole with low dielectric constant and high thermal stability. Macromolecules. 2017;50(17):6552–6560.
- Gu JW, Dong WC, Tang YS, et al. Ultralow dielectric, fluoride-containing cyanate ester resins with improved mechanical properties and high thermal and dimensional stabilities. J Mater Chem C. 2017;5(28):6929–6936.
- Wang B, Shang Y, Ma Z, et al. Non-porous ultra low dielectric constant materials based on novel silicon-containing cycloolefin copolymers with tunable performance. Polymer. 2017;116:105–112.
- Li Q, Chen L, Gadinski MR, et al. Flexible high-temperature dielectric materials from polymer nanocomposites. Nature. 2015;523(7562):576–579.
- Cheng Y, Chen W, Li Z, et al. Hydrolysis and condensation of a benzocyclobutene-functionalized precursor for the synthesis of high performance low-K polymers. RSC Adv. 2017;7(24):14406–14412. https://doi.org/10.1039/C7RA00141J.
- Cheng Y, Tian S, Shi Y, et al. Benzocyclobutene organosiloxane resins prepared by alcoholysis of BCB functionalized chlorosilane for highly crosslinked low-k thermosets. Eur Polym J. 2017;95:440–447.
- Tong J, Diao S, Jin K, et al. Benzocyclobutene-functionalized poly(m -phenylene): a novel polymer with low dielectric constant and high thermostability. Polymer. 2014;55(16):3628–3633.
- Cao K, Yang L, Huang Y, et al. High temperature thermosets derived from benzocyclobutene-containing main-chain oligomeric carbosilanes. Polymer. 2014;55(22):5680–5688.
- Ramabhadran RO, Mhb AR, Collier SJ, et al. An unusual [4+4] cycloadduct from an o-quinodimethane: characterisation and computational studies. Tetrahedron. 2018;74(1):1–5.
- Yang J, Cheng Y, Xiao F. Synthesis, thermal and mechanical properties of benzocyclobutene-functionalized siloxane thermosets with different geometric structures. Eur Polym J. 2012;48(4):751–760.
- Zuo X, Chen J, Zhao X, et al. Synthesis and characterization of siloxane resins derived from silphenylene-siloxane copolymers bearing benzocyclobutene pendant groups. J Polym Sci Pol Chem. 2008;46(23):7868–7881.
- Yang L, Cao K, Huang Y, et al. Synthesis and properties of cross-linkable polysiloxane via incorporating benzocyclobutene. High Perform Polym. 2014;26(4):463–469. https://doi.org/10.1177/0954008313518567.
- Wang J, Luo Y, Jin K, et al. A novel one-pot synthesized organosiloxane: synthesis and conversion to directly thermo-crosslinked polysiloxanes with low dielectric constants and excellent thermostability. Polym Chem UK. 2015;6(33):5984–5988.
- Rubinsztajn S, Cella JA. A new polycondensation process for the preparation of polysiloxane copolymers. Macromolecules. 2005;38(4):1061–1063.
- Chojnowski J, Rubinsztajn S, Cella JA, et al. Mechanism of the B(C6F5)3-Catalyzed reaction of Silyl hydrides with Alkoxysilanes. Kinetic and spectroscopic studies. Organometallics. 2005;24(25):6077–6084.
- Chojnowski J, Rubinsztajn S, Fortuniak W, et al. Synthesis of highly branched Alkoxysiloxane−Dimethylsiloxane copolymers by nonhydrolytic dehydrocarbon polycondensation catalyzed by tris(pentafluorophenyl)borane. Macromolecules. 2008;41(20):7352–7358.
- Chojnowski J, Kurjata J, Fortuniak W, et al. Hydride transfer Ring-Opening Polymerization of a cyclic oligomethylhydrosiloxane. route to a polymer of closed multicyclic structure. Macromolecules. 2012;45(6):2654–2661.
- Oestreich M, Hermeke J, Mohr J. A unified survey of Si-H and H-H bond activation catalysed by electron-deficient boranes. Chem Soc Rev. 2015;44(8):2202–2220.
- Tian S, Li J, Cai Z, et al. Piers-Rubinsztajn reaction for BCB functionalized silphenylene/silbiphenylene siloxane oligomers to highly crosslinked low-k thermosets. Eur Polym J. 2018;108:373–379.
- Jikei M, Kakimoto M. Hyperbranched polymers: a promising new class of materials. Prog Polym Sci. 2001;26(8):1233–1285.
- Devaraju S, Vengatesan MR, Selvi M, et al. Hyperbranched polysiloxane-based diglycidyl ether of bisphenol a epoxy composite for low k dielectric application. Polym Compos. 2013;34(6):904–911.
- Zhuo D, Gu A, Liang G, et al. Flame retardancy and flame retarding mechanism of high performance hyperbranched polysiloxane modified bismaleimide/cyanate ester resin. Polym Degrad Stabil. 2011;96(4):505–514
- Kurf Rst M, Blechta V, Schraml J. Geminal J(29Si-O-29Si) couplings in oligosiloxanes and their relation to direct 1J(29Si-13C) couplings. Magn Reson Chem. 2011;49(8):492–501.
- Migulin D, Tatarinova E, Meshkov I, et al. Synthesis of the first hyperbranched polyorganoethoxysilsesquioxanes and their chemical transformations to functional core-shell nanogel systems. Polym Int. 2016;65(1):72–83.
- Ocola EJ, Shin HW, Laane J. Infrared and Raman spectra and theoretical calculations for benzocyclobutane in its electronic ground state. Spectrochim Acta A Mol Biomol Spectrosc. 2015;136:58–63.