Abstract
To study the impacts of emission controls on aerosol physical and chemical properties, real-time measurements of size-resolved aerosol number concentration and chemical composition were conducted in urban Beijing during the 2014 Asia-Pacific Economic Cooperation (APEC) summit, in a period that a series of measures, for example shutting down or halting production from factories and power plants, and restricting the number of vehicles on the roads were implemented in Beijing and surrounding regions. Significantly, reductions in particle mass concentration (55% for PM2.5 and 48% for PM10) were observed during the APEC summit. A clear decrease in secondary inorganic aerosols (SIA), such as sulphate, nitrate and ammonium, was found during APEC, with the reduction ranged from 65.7 to 72.2% for PM1, in which sulphate showed the largest decrease compared with periods before APEC. As a comparison, organics showed a much smaller decrease of 44.3% for PM1 during APEC. These changes were mainly caused by large reductions in accumulation mode particles, which decreased by 36% compared with 19% for Aitken mode particles. The results from the positive matrix factorization (PMF) of particle number concentration indicate that regionally transported aerosols showed significant decreases (70%), similar to those of SIA during APEC, whereas primary factors from traffic and local combustion sources presented much smaller decreases, with the reduction ranged from 4 to 40%. The elevated contributions of these sources indicated the presence of strong local source emissions. The changes in particle chemical composition, size distribution and sources during the evolution of pollution episodes with and without emission controls are further illustrated. Our results highlight the importance of regional atmospheric transport in the formation of severe pollution episodes in Beijing, indicating that reducing the precursors of secondary aerosol over regional scales represent the key steps to reduce the urban particulate pollution. However, stricter emission controls on local source emissions are needed to further mitigate air pollution in Beijing.
1. Introduction
Atmospheric aerosols, especially fine particles of particulate matter (PM) with aerodynamic diameters of less than 2.5 μm, have significant impacts on air quality and visibility (Watson, Citation2002; Molina and Molina, Citation2004) and are detrimental to human health (Liu et al., Citation2013). Aerosol particles also exert highly uncertain radiative forcing impacts on climate change (Forster et al., Citation2007). In addition to aerosol mass, the size distribution of atmospheric aerosols, together with chemical composition, provides important information on the sources and processes of aerosols and for assessing their health and climatic effects. For example, absorption and scattering of incoming radiation depends on particle size and composition (Seinfeld and Pandis, Citation2006; Nishita et al., Citation2007). The degradation of visibility on hazy days was mainly attributed to the number concentration of accumulation mode particles (See et al., Citation2006). Furthermore, close relationship between particle number concentration and cardiovascular mortality has been reported in recent studies (Breitner et al., Citation2011; Leitte et al., Citation2011). All of these observations show that particle number concentration warrants equal or greater attention than mass concentration.
Over the past decade, numerous studies have been done on urban aerosol number size distributions, including long-term measurements such as in Helsinki, Finland (Hussein et al., Citation2004) in Leipzig, Germany (Wehner and Wiedensohler, Citation2003), and in London UK (von Bismarck-Osten et al., Citation2013), and short-term monitoring like in Atlanta, USA (Woo et al., Citation2001), in Birmingham, UK (Shi et al., Citation2001), and in Braunschweig, Germany (Ruths et al., Citation2014). However, most of the research was done in the developed countries, only a few studies were carried out in the heavy polluted megacities of the newly industrialized countries, such as Mexico (Dunn et al., Citation2004), New Delhi (Mönkkönen et al., Citation2005), and Beijing (Yu et al., Citation2005; Wu et al., Citation2007, Citation2008; Wehner et al., Citation2008). The characteristics of particle number size distributions (PNSD) in these megacities with much higher number concentrations are usually different from the cities in the developed countries (Yue et al., Citation2009), because of the high population density, fast industrialization, and rapid urbanization in these megacities. As the capital of China and a major megacity, Beijing has been experiencing serious air pollution due to the large quantity of air pollutants emitted by anthropogenic activities, e.g. traffic, industry, and power plants (Chan and Yao, Citation2008). Although recent real-time measurements of aerosol composition have improved our understanding of the evolutionary processes of haze pollution, which shows relatively constant variations in secondary inorganic aerosols (SIA) and secondary organic aerosols during persistent evolution periods, while further evolution associated with fog processing and significantly enhanced sulphate levels (Sun et al., Citation2016), most of them focus on aerosol chemical composition; few of them concern aerosol number concentration and size distribution (Yue et al., Citation2009; Guo et al., Citation2014; Liu, Hu, et al., Citation2016). Simultaneous observations of aerosols’ physical and chemical properties, as well as information on their respective sources during severe pollution events, are rather limited.
To ensure good air quality during APEC, strict emission controls were implemented in Beijing and in the surrounding regions. These included restrictions on the number of vehicles in operation, factory operations, construction activities, and open barbeques, which provides a unique opportunity to study the impacts of source emissions on the physical and chemical properties of aerosols in a megacity such as Beijing. In this study, we conduct real-time measurements of size-resolved aerosol number concentration and chemical composition from 9 October to 12 November 2014. The aerosol particle number concentration (PNC), chemical composition, size distributions and sources of PNC are investigated in detail. In particular, the impacts of emission controls on aerosol PNC, size distributions and chemical compositions are elucidated.
2. Experimental Methods
2.1. Site description
This study took place from 9 October to 12 November 2014 at the Institute of Atmospheric Physics, Chinese Academy of Sciences (IAP, 39°58′N, 116°22′E), between north third and fourth Ring roads in Beijing. This site is located within educational, commercial and residential areas, representative for an area of typical urban particulate pollution in Beijing. No major pollution sources are detected nearby, except for the Jingzang Expressway, which is 300 m to the east. During the sampling period, the sampling instruments were set up on the rooftop of a two-storey building (approximately 10 m above ground level).
2.2. Instrumentation
A scanning mobility particle sizer (SMPS), which comprises a model TSI 3080 electrostatic classifier and a model TSI 3775 condensation particle counter (CPC, TSI Inc., St Paul, USA), was used to measure particle size distributions in the range of 14.5–710.5 nm. The flow rate was 0.3 L/min for the sample air and 3.0 L/min for the sheath air. Ambient air was sampled by the SMPS from a 0.5 inch stainless steel tube with a low flow PM10 inlet. The relative humidity (RH) within the systems was maintained below 30% by adding a silica-gel dryer in the inlet line and in the sheath air cycle to avoid water condensation in the inlet systems, and size-dependent diffusional and gravitational losses for the inlet line have been corrected (Willeke and Baron, Citation1993). The size distribution of individual submicron aerosol species was measured on an HR-AMS. Calibration of the HR-AMS and analysis of the spectra during the study period are discussed in detail by Zhang et al. (Citation2016). The black carbon (BC) in PM1 was measured with a multi-angle absorption photometer (5012 MAAP, Thermo Scientific, USA). In addition, PM mass concentrations (PM10 and PM2.5) were measured using a tapered element oscillating microbalance (TEOM 1405-DF, Thermo Scientific, USA) with a Filter Dynamic Measurement System. Gaseous species (such as NO, NO2, O3 and SO2) were simultaneously measured using a series of gas analysers from Thermo Scientific. Visibility was measured using a visibility sensor (Model 6000, Belfort Instruments, USA) with wavelength of 880 nm. Meteorological parameters (relative humidity, air temperature, wind speed and direction) were obtained from an automatic meteorological observation instrument (Milos520, Vaisala, Finland). The time basis for all data in the study was Beijing time (UTC+8).
2.3. Positive matrix factorization
PMF is one of the most common receptor models used in the identification of PM sources in the atmosphere and their contributions (Paatero and Tapper, Citation1994; Paatero, Citation1997). In brief, the non-negative factor analysis model is applied to a measured data matrix X (n × m), where n is the number of samples and m is the number of chemical species, to solve for two matrices G (n × p) and F (p × m) as well as a residual matrix E, where p is the number of sources and E is the unexplained portion of X. A least-squares algorithm is used to iteratively solve for the matrices by minimizing the object function (Q) defined as follows:(1)
where eij is an element in the n × m matrix, E, of residual concentrations and sij is the analytical uncertainty of the jth species in the ith sample.
Several studies have been made to use PNSD data to elicit source information (Norris et al., Citation2008), and only PNSD data was considered in most of previous studies (Vu et al., Citation2015). However, more complete source apportionments were impossible unless the PNSDs could be combined with highly time resolved particle composition measurements (Viana et al., Citation2008). In this study, PMF was applied to combined PNSD and high-temporal-resolution of chemical composition data and gaseous pollutants to find additional source types and provide insight into the characteristics and processes of these sources as we conducted in our previous studies (Liu et al., Citation2014; CitationLiu, Wang, et al., 2016). Furthermore, organic aerosol (OA) components including a hydrocarbon-like aerosol (HOA), biomass-burning OA (BBOA), cooking organic aerosol (COA), and two oxygenated OA (semi-volatile oxygenated OA (SV-OOA) and low-volatility oxygenated OA (LV-OOA)) which reported by Zhang et al. (Citation2016), were also combined with PNSD data to resolve a more complete understanding of the sources of PNC in Beijing. In addition, to smooth the size distribution data and minimize the error as suggested by Zhou et al. (Citation2005), the values for each set of three consecutive size bins of SMPS were averaged to produce 42 new size intervals. Thus, the whole data-set includes 56 variables representing different PNSD, OA components (HOA, BBOA, COA, SV-OOA and LV-OOA), particle chemical components (PCC) in PM1 (nitrate, sulphate, ammonium, chlorine and BC) and gaseous pollutants (NO, NO2, O3, SO2).
The PMF solutions were investigated in detail by evaluating the spectral profiles and time series of PNC factors as a function of the rotational parameter (fPeak). The fpeak matrix is a p × p matrix with all off-diagonals set to a non-zero fpeak value. By trying a range of fpeak values, resulting solutions can be evaluated for change in the factor profiles, contributions, and change in the Q-value (Norris et al., Citation2008). The robustness of the factor analysis was evaluated by exploring an fpeak rotational analysis which increase or decrease elements of the matrix G or F. In this study, the solutions produced by rotations across fpeak values from −5 to 5 using the EPA PMF application were examined. The number of possible sources (p) was initially determined by investigating the ratio of Q to ‘Qexp’ which is theoretical Q defined by the equation, n × m − p × (n + m). A smaller change in Q/Qexp with increasing factor numbers may indicate that the interesting number of factors is not stable (Ulbrich et al., Citation2009). In addition, the range of possible solutions was examined to see if one solution was more physically realistic than the others. Finally, the source types were identified as described in detail in our previous studies (Liu et al., Citation2014; CitationLiu, Wang, et al., 2016). Briefly, the following information was analysed: (1) the profiles of particle number and volume size distribution; (2) association between each source factor and the measured chemical and gaseous species; (3) the diurnal behaviour of the source; (4) source directionality as provided by the conditional probability function (CPF); and (5) source contribution to the total apportioned particle concentrations. A summary of these PMF results is shown in Figs. S3–S5 in the Supplement. Description about the CPF could be found in the Supplement.
3. Results and discussion
3.1. Particle mass and number concentration
The time series of aerosol mass and number concentrations during the entire study period are showed in Fig. . As indicated in the figure, four pollution episodes were observed before APEC (9 October–2 November), while two were observed during APEC (3–12 November). Stagnant meteorology, characterized by low wind speed and high relative humidity, was observed to be closely related to the evolution of the pollution episodes. The average mass concentrations of PM10 and PM2.5 during APEC were 102.3 ± 70.5 μg m−3 and 53.3 ± 49.2 μg m−3, respectively, which were 47.8% and 54.6% lower than those measured before APEC. The mass ratios of PM2.5/PM10 changed from 0.60 before APEC to 0.52 during APEC, which suggests that fine particles were reduced. In addition, mean visibility during APEC also improved compared with periods before APEC (Fig. e). The days with the worst visibility (<1 km) during the observation period were all found to be occurred before APEC. Chemical components in fine particles also showed significant reductions during APEC, as shown in Fig. , mass concentrations of SIA and organics were decreased in all size bins during APEC, especially in the size range larger than 300 nm in diameter. In addition, the peak diameter of these species was larger before APEC, and the distribution of which appears narrower than those during APEC. For example, the median diameter (MD) and geometric standard deviations (GSD) for the mass size distribution of organics was 537 nm and 0.7 before APEC, while the MD and GSD of which were 426 nm and 0.9 during APEC. Statistics of the mass concentrations of SIA and organics before and during APEC are shown in Table . Average mass concentration of organics, nitrate, sulphate and ammonium before APEC were 32.7, 20.7, 10.9 and 10.0 μg m−3, respectively. A clear decrease in SIA, such as nitrate, sulphate and ammonium, was observed during APEC, with the reduction ranged from 65.7 to 72.2%, in which sulphate showed the largest decrease compared with periods before APEC. In comparison, a much smaller decrease of 44.3% for organics was observed during APEC. In addition, black carbon (BC) and chlorine also showed much smaller decrease of 37.3 and 41.0% during APEC. The chemical composition of PM1 before APEC was mainly composed of organics, accounting for 39.4%, followed by nitrate at 25.0%, sulphate at 13.1%, ammonium at 12.1% and BC at 7.2%. The average aerosol composition during APEC showed significant changes. The contribution of organics showed a large increase, accounting for nearly half of PM1, whereas that of SIA was decreased from 50.2 to 36.4%. These results suggest that SIA and organics showed different behaviours before and during APEC.
Fig. 1. The time series of (a) temperature (T) and relative humidity (RH), (b) wind speed (WS) and wind direction (WD), (c)evolution of PNSD and mean diameter (circles) of the dominant mode, (d) particle number concentrations in different modes and (e) the mass concentration of PM2.5 and PM10 and visibility during the measurement period. The APEC summit period and six pollution episodes (Ep1–Ep6) are marked for further discussion.
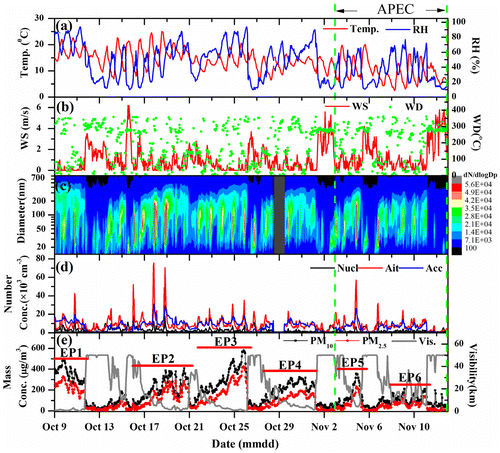
Fig. 2. Average particle (a) number, (b) surface, (c) volume size distribution and (d–h) average mass size distributions of chemical components in PM1 measured before and during the APEC summit. The median diameters (MD) and GSD for the mass size distributions of chemical components in PM1 are also shown.
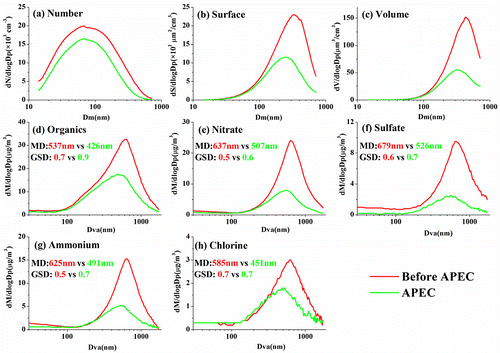
Table 1. Summary of average meteorological parameters, particle matter and gaseous species, PNC in different modes, PSC, particle volume concentration (PVC) and mass concentrations of PM1 species for the entire study, before and during APEC, and also the change percentages during APEC.
The variations in PNC were similar to those of particle mass before and during APEC. In this study, the measured PNSDs were fitted and parameterized by a multiple log-normal distribution function (Seinfeld and Pandis, Citation2006); three modes were identified corresponding to the nucleation mode (Nucl, 14–30 nm), Aitken mode (Ait, 30–100 nm) and accumulation mode (Acc, 100–710 nm). Table gives the particle number statistics in different modes. The average number concentrations during the observation periods were 3.1 × 103 cm−3 for nucleation mode, 9.0 × 103 cm−3 for Aitken mode and 7.5 × 103 cm−3 for accumulation mode. A clear decrease in accumulation mode (35.9%) and nucleation mode (29.5%) particles was found during APEC. As a comparison, the variations in Aitken mode particles were more dramatic during APEC, and the changes were not as significant as those for accumulation mode and nucleation mode particles. Therefore, the size distribution of PNC was changed significantly, large reduction of particle surface concentration (PSC) was observed during APEC, especially for the accumulation mode particles (Fig. ). The Aitken mode particles contributed most to PNC before APEC, accounting for 45.0%, followed by the accumulation mode at 39.3% and nucleation mode at 15.7%. During APEC, the proportion of Aitken mode particle in PNC increased to 49.7%, whereas the contribution of accumulation mode particles decreased to 34.6%. In addition, the ratio of Acc/Ait was 0.87 before APEC, which was similar to ratios reported in Yue et al. (Citation2009) of 0.9 and Liu, Hu, et al. (Citation2016) of 0.8 during stagnant periods in Beijing. It is interesting to note that the ratio of Acc/Ait was 0.69 in pollution episodes during APEC, much lower than the values during stagnant periods reported in previous studies (Yue et al., Citation2009; CitationLiu, Hu, et al., 2016). These results indicate that both aerosol composition and sources would be significantly different before and during APEC due to regional emission controls.
3.2. Diurnal variation of PNC and chemical composition
The diurnal pattern of aerosol number concentration of each mode was investigated separately. Figure shows that the diurnal behaviours of particle number concentration (PNC) and chemical species in PM1 before and during APEC. The diurnal variation in the particle number concentration of the nucleation mode showed a three-peak pattern before APEC (Fig. b). These three peaks are presented as morning peak, midday peak, and evening peak, respectively. The morning peak occurred between 06:00 and 10:00 and was the result of a higher traffic density during rush hour combined with a lower mixing layer height and lower ambient temperature (Wu et al., Citation2008). The midday peak was mainly the result of new particle formation during daytime (Gao et al., Citation2012), which is frequently observed throughout the year in Beijing. The evening peak, which occurred between 18:00 and 20:00, was associated with evening rush hour traffic. Simultaneously, the low wind speed and decreasing mixing layer height also resulted in a less intensive vertical mixing and dilution for particles during the night (Wu et al., Citation2008). The diurnal pattern of Aitken mode particles was similar to that of the nucleation mode, the morning and evening peaks of which were also associated with traffic emissions in morning and evening rush hours. In addition, the diurnal variation of Aitken mode and the total particle number concentration showed the same trend (Fig. a and c), which indicated the variation of the number concentration of Aitken mode particle took a dominant role in the variation of total particle number concentration at urban Beijing. Accumulation mode particles showed a substantially different diurnal cycle from that of ultrafine particles, characterized by a pronounced night-time peak and a nearly non visible midday peak. The peak in the evening (18:00–20:00) may be due to the increase in the vehicle circulation during evening rush hours and to emissions from combustion activities. Additionally, radiational cooling after sunset results in the development of a stable boundary layer near the surface (Tang et al., Citation2015), inhibiting vertical mixing of the fresh emissions from the surface which, under low dilution conditions, may contribute to the observed peak.
Fig. 3. Diurnal profiles of the total PNC, PNC in different modes and mass concentrations of PM1 species measured before and during the APEC summit.
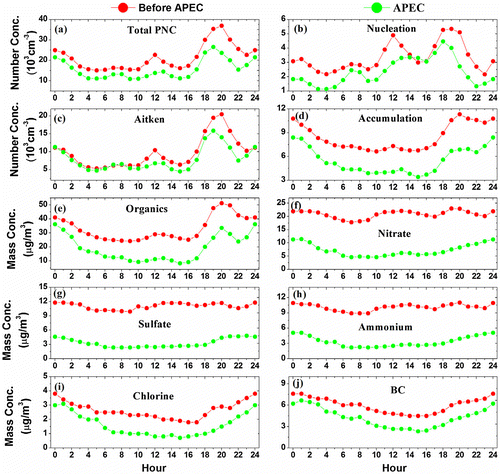
It is interesting to note that a weaker morning peak was found for Aitken mode particles compared with that of nucleation mode particles. This weak morning peak of Aitken mode particles in this study was different from those found in European cities, such as Barcelona and London (Reche et al., Citation2011), where a more distinct and also more pronounced peak of PNC was observed during morning rush hours. Interestingly, distinct morning peak of Aitken mode particles was observed in fall of 2004–2005, at an urban site located in the north-western urban area of Beijing (Wu et al., Citation2008). The inconspicuous morning peak of Aitken mode particles in this study would be attributed to the weaker wind speed during the observation period. As shown in Fig. S6a, average wind speed during morning rush hour was 1.3 m/s in fall of 2004, which reduced to 0.9 m/s in fall of 2014. As a result, transportation of traffic emissions to the receptor site (IAP), especially the ‘aged’ traffic emissions, were reduced during the morning rush hours. Further support comes from the distinct evening peak of Aitken mode particles observed in this study, as compared to that in Wu et al. (Citation2008), since the average wind speed during evening rush hours changed little between 2004 and 2014 (Fig. S6a). Actually, similar diurnal patterns of Aitken mode particles were also found during the same period at an urban site located between west third and fourth Ring roads in Beijing (Fig. S6c).
Note that the evening peak of Aitken mode particles occurs at approximately 20:00, followed by the peak number concentration of the nucleation mode. This is probably caused by the ‘fresh’ traffic emission particles growing into the Aitken mode range during the ageing processes. In addition, cooking emissions and local combustion activities, as discussed later in Section 3.3, would also be responsible for the evening peak of Aitken mode particles. Different from the midday peak of nucleation mode particles, which associated with new particle formation, the midday peak of Aitken mode particles was also related to the extension of the morning traffic peak. The diurnal cycles of nucleation mode and Aitken mode particles were consistent with that of NOx, whereas the diurnal pattern of BC does not reflect the morning and evening traffic trends, whereas ultrafine particles and BC often share the same traffic peaks during morning and evening rush hours in European urban environments (Reche et al., Citation2011; Dall’Osto et al., Citation2012; Brines et al., Citation2014, Citation2015). A likely explanation is that over 70% of BC emissions in urban Beijing were attributed to diesel trucks and heavy-duty vehicles (Wang et al., Citation2011), and the diesel trucks and heavy-duty vehicles are only allowed to operate inside the city between 22:00 and 06:00, not in the morning and evening rush hours. Thus, the unique traffic control measures in Beijing lead to the phenomenon that diurnal variation of BC does not reflect the morning and evening traffic trends.
The diurnal cycles of PNC during APEC differed significantly. The magnitude of the three peaks for nucleation mode particles were all reduced compared to those before APEC, suggesting a reduction of traffic density and also a weakening of regional nucleation events during APEC. In contrast, the reduction of Aitken mode particles during daytime was much smaller, the concentration of which in the early morning (00:00–08:00) during APEC was even approximately the same compared to that before APEC. The smaller reduction of Aitken mode particles compared with that of nucleation mode particles would be attributed to the smaller reduction of aged traffic emissions and local combustion activities during APEC, as discussed later in section 3.3. In addition, accumulation mode particles showed a decrease of approximately 42% during daytime, and the differences before and during APEC were much smaller at night-time. These results indicate that strong local source emissions continued during APEC despite the strict emission controls.
The diurnal variations of SIA before APEC were all characterized by gradual increases during daytime, which were primarily driven by photochemical processing and regional transportation, considering the rising planetary boundary layer height during daytime (Tang et al., Citation2015). Organics showed a substantially different diurnal cycle from that of SIA, characterized by a pronounced night-time peak and a minor noon peak. The night-time peak of organics would be attributed to the favourable partitioning of semi-volatile organic compounds to the particulate phase driven by temperature decreases and relative humidity increases at night, and would also be attributed to vertical transport of regional pollutants from above to ground level (Lim and Turpin, Citation2002). The diurnal cycles of SIA and organics during APEC were similar with those before APEC, although a large reduction of which was found compared to those before APEC. In addition, the larger reductions of SIA and organics were observed during the daytime, reductions of these species at night were much smaller and the concentrations of which were nearly twice what they were during the day. In addition to the regional emission control measures, the routine circulation of mountain valley breezes played a dominant role in driving these diurnal variations (Xu et al., Citation2015). Apart from SIA and organics, chlorine and BC showed similar diurnal cycles before and during APEC, with the greatest reduction occurring during daytime. As these two species were mainly from combustion sources, e.g. biomass burning and coal combustion, the small decrease in chlorine and BC at night likely indicates that a considerable amount of combustion emissions occurred during APEC. It is interesting to note that a significantly higher concentration of black carbon was found at night compared to that during the day. Similar diurnal cycle of BC was reported by Han et al. (Citation2009), indicating higher BC source emissions at night in Beijing. This result is consistent with the diurnal variations of diesel trucks and heavy-duty vehicles on road, which are only allowed to operate inside the city between 22:00 and 06:00.
3.3. Sources of particle number concentration
Eight factors of PNC were identified by PMF analysis of a combined data-set of PNSD and PCC. These included six primary factors (three traffic factors, one cooking factor and two combustion factors) and two secondary aerosol factors (secondary aerosol and regional aerosol) (Table ). The particle number spectra and time series of the eight PNC factors are shown in Fig. .
Table 2. The PMF revolved factors for particle number concentration (PNC) for the entire study, before and during APEC, and also the change percentages during APEC.
Fig. 4. Normalized feature profiles for particle number and volume size distribution (left panel) and time series (right panel) of eight PNC factors resolved by the PMF. Also shown in the right panel are the time series of external tracers including black carbon (BC), Chlorine (Cl−), O3, SIA (sulphate + nitrate + ammonium) and two organic aerosol components (cooking (COA) and (BBOA)). The two pie charts show the average source composition of PNC measured before and during the APEC summit, respectively. The correlation coefficients between PNC factors and external tracers measured before and during APEC are also shown in the figure.
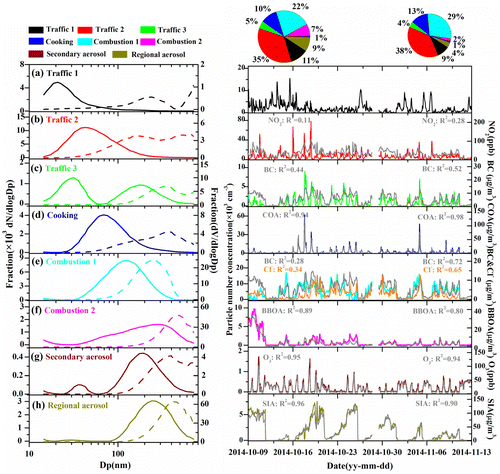
The size distribution of traffic factors is illustrated in Fig. a–c. The ‘fresh’ traffic emissions (Traffic 1) are characterized by a major number mode at approximately 20–30 nm, which is consistent with observations at various urban sites (Zhu et al., Citation2002; Wehner et al., Citation2002; Li et al., Citation2007; Ban-Weiss et al., Citation2010; Al-Dabbousa and Kumar, Citation2015). It should be noted that secondary aerosol from new particle formation would also be present in this factor, as a midday peak was observed for this factor (Fig. S3a). In contrast to the ‘fresh’ traffic emission factor, the aged traffic emission (Traffic 2) presented a major mode at approximately 40–50 nm in the number distribution and 150 nm in the volume distribution (Fig. b); evidence from other researchers has shown that gasoline engine vehicles emit particles in this range (Harris and Maricq, Citation2001; Kittelson et al., Citation2006; Rönkkö et al., Citation2013). The diurnal cycles of aged traffic emissions follow the diurnal pattern of NO2, reaching a minor peak during the morning rush hours (07:00–09:00) and a major peak in the evening rush hours (17:00–20:00) (Fig. b). In addition, a noontime peak of this factor was also related to the extension of the morning traffic peak, which is similar to the local traffic factor found in Beijing (Wang et al., Citation2013) and in European cities (Brines et al., Citation2015). The morning peak of ‘fresh’ traffic emissions correlated well with NO, although weak correlation was observed during the evening rush hours, probably due to the presence of higher O3 during the evening rush hours compared that during the morning rush hours (Fig. S1d), which attributed to the absence of evening traffic peak for NO. Although a weak correlation was observed between aged traffic factor and NO2 during the whole observation period, the relationship between the two was much stronger during the traffic rush hours, especially for that during APEC period (Table ). In addition, close relationship between ‘fresh’ traffic factor and NO during the traffic rush hours was also observed during APEC period (Table ). These results suggest a substantial change in the sources of either traffic emissions or NOx during APEC. A likely explanation is that more complex sources of NOx from traffic, industry and biomass burning before APEC were regulated during APEC, which enhanced the role of traffic as the source of NOx. Note that the diurnal cycle of the ‘fresh’ traffic factor showed clear morning rush hour peak, which were almost invisible for the aged traffic factor (Fig. b). The weak morning peak of aged traffic factor would be attributed to the lower wind speed during the morning rush hours compared with that during the evening rush hours (Fig. S2a), which reduced the transportation of aged traffic emissions from more distant areas to the receptor site (IAP). Traffic 3 presented the modes at around 30 nm associated with nucleation mode and at around 150 nm corresponding to solid carbonaceous particles from diesel exhaust (Wehner et al., Citation2009). A previous study has shown that particles with similar modes were generated during dilution of diesel exhaust emissions (Ntziachristos et al., Citation2007). Traffic 3 correlated moderately with BC before and during APEC (R2 = 0.44 and 0.52, respectively), and the diurnal cycle of which showed a dominate source during night-time (Fig. c), different from Traffic 1 and 2, but consistent with the diurnal variations of diesel trucks and heavy-duty vehicles on road, which are only allowed to operate inside the city between 22:00 and 06:00. During the daytime, the vehicles in the urban area of Beijing are dominated by gasoline-powered passenger cars, which contribute more than 90% to the total number of vehicles (source: Beijing Transportation Research Center). Thus, Traffic 1 and 2 would most probably be represented as traffic emissions from gasoline-powered vehicles, whereas Traffic 3 represented traffic emissions from diesel trucks and heavy-duty vehicles.
Fig. 5. Diurnal profiles of the number concentrations of PNC factors measured before and during the APEC summit.
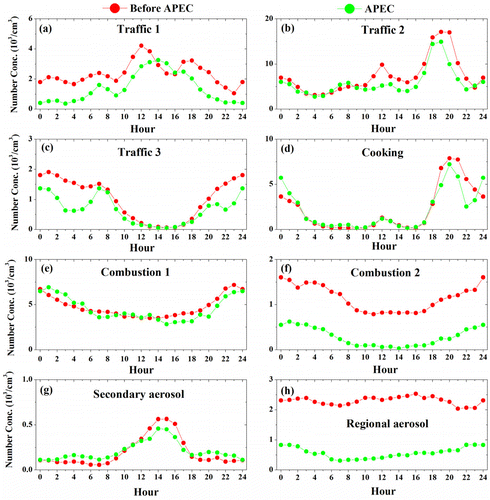
Table 3. Correlation coefficients between PNC factors source profiles and external tracers.
The cooking related factor showing that a major number mode at approximately 60–70 nm (Fig. d), consistent with previous studies that particles emitted from cooking activities have unimodal behaviour with a peak generally between 20 and 70 nm (Vu et al., Citation2015). This factor contains substantial amounts of COA and SV-OOA, but it has little correlation with secondary inorganic ions (R2 < 0.10), consistent with previous measurements that aerosols emitted from Chinese cooking are almost composed of organic compounds (He et al., Citation2004). A clear and unique diurnal pattern of this factor provides another piece of strong evidence for it being cooking related: it presented high values at noon (11:00–13:00) and in the evening (18:00–20:00) (Fig. d), which is consistent with dining hours of the local residents (Huang et al., Citation2010). Further support comes from the diurnal pattern of this factor is consistent with that of COA (Fig. S3d). The result of the CPF analysis suggests this source appears to be located in the east and north-east direction of the site (Fig. S4d), which corresponds to the location of Chinese restaurants in that direction. Due to the unique Chinese cooking habits and culture, cooking emissions have been regarded as one of the major aerosol sources in urban environments of North China (Liu, Mauzerall, et al., Citation2016). Therefore, it was a consequential result to identify a significant contribution (nearly 11%) of cooking-related aerosol to particle number in this study.
The size distribution of combustion factors is illustrated in Fig. e and f. The first kind of combustion factor (Combustion 1) was characterized by number and volume mode at approximately 100 and 300 nm, respectively. Particles with such modes originated from combustion activities, such as aged coal-fired power plant emissions (Brock et al., Citation2002), waste incineration emissions (Buonanno et al., Citation2009) and freshly generated smoke particles from biomass burning (Janhäll et al., Citation2010). Combustion 1 correlated moderately with BC and chlorine before APEC (R2 = 0.28 and 0.34, respectively), whereas a much stronger correlation between them was found during APEC (R2 = 0.72 and 0.65, respectively). The stronger correlation between Combustion 1 and BC could be attributed to the reduction of traffic emissions during APEC. Especially for the large reduction of Traffic 3 (36.7%), as over 70% of BC emissions in urban Beijing were attributed to this factor, which associated with diesel trucks and heavy-duty vehicle emissions during night-time (Wang et al., Citation2011). In addition, the close relationship between Combustion 1 and chlorine during APEC indicated that coal combustion became the dominant source of Combustion 1 during APEC (Yao et al., Citation2002; Zhang et al., Citation2013). The second kind of combustion factor (Combustion 2) was associated with biomass burning, as strong correlations between this factor and BBOA were found before and during APEC (R2 = 0.89 and 0.80, respectively). In addition, Combustion 2 shows a major number mode at approximately 200–300 nm. During the ageing process, particles emitted by biomass burning can increase their median diameter up to 240 nm by coagulation/condensation (Janhäll et al., Citation2010). Thus, Combustion 2 could be represented as aged smoke particles emitted by biomass burning from distant areas to the receptor site. The combustion factors showed similarly pronounced diurnal cycles before and during APEC, with night-time concentrations approximately 2–3 times the level of daytime concentrations (Fig. e and f). The diurnal cycle of these two combustion factors (Combustion 1 and 2) resembled that of BC and BBOA, respectively. In addition, a much larger reduction of Combustion 2 (82.7%) compared to Combustion 1 (4.3%) was found during APEC. The significantly smaller reduction of Combustion 1 indicates that a considerable amount of local combustion emissions occurred during APEC, which is consistent with the small decrease of BC and chlorine during APEC.
A bimodal PNSD, with a minor peak at 30–40 nm and a major peak at approximately 200 nm, characterizes the secondary aerosol factor; the volume distribution was largely unimodal, with a mode close to 300–400 nm. The profiles of particle number and volume size distribution for this factor were consistent with the spectral characteristics of secondary aerosols resolved in summer (Liu et al., Citation2014) and winter (CitationLiu, Wang, et al., 2016) of Beijing. In addition, the diurnal pattern of the secondary aerosol factor is consistent with that of O3 (Fig. S3), both of which predominantly appear in the afternoon (14:00–15:00), suggesting this factor was associated with local secondary aerosol formation processing. Similar pronounced diurnal cycles were observed for the secondary aerosol before and during APEC (Fig. g). Note that a considerable reduction in this factor during the afternoon was observed during APEC, probably due to weakened photochemistry as a result of the reduction of NOx and SO2.
The regional aerosol factor was characterized by number and volume mode at approximately 300 and 500 nm, respectively (Fig. h). This factor was associated with long-range transported aerosols, which comprise mostly of accumulation mode particles. Particles in the accumulation mode can be transported over long distances because they are too small to be deposited by inertial and gravitational processes and too large to be influenced appreciably by growth processes (Hussein et al., Citation2006). In addition, the regional aerosol factor was strongly correlated with SIA before and during APEC (R2 = 0.96 and 0.90, respectively), as SIA was formed mainly over a regional scale and was less influenced by local sources (Xu et al., Citation2015). This tight correlation further suggests that this factor originated from regional transport. The diurnal cycle of the regional aerosol showed a gradual increase during daytime before APEC, with the maximum of which appears in the afternoon (15:00), consistent with the development of planetary boundary layer height during the daytime (Fig. S6a)), suggesting the regional transportation of accumulation mode particles dominating the diurnal variation of the regional aerosol factor at the receptor site. Comparatively, the regional aerosol factor showed a similar diurnal cycle as that of SIA during APEC, characterized by a higher concentration at night. This result suggests a weaker influence of regional transportation during daytime, and the less intensive vertical mixing and dilution for particles contributed to the night peak of regional aerosol factor during APEC.
Overall, traffic emission particles dominated PNC composition before APEC (51%), with ‘fresh’ traffic, aged traffic and traffic emissions from diesel trucks and heavy-duty vehicles accounting for 11, 35, and 5%, respectively. The combustion factors (29%) emerged as the second largest contributor to PNC, followed by the cooking factor (10%), regional aerosol factor (9%) and secondary aerosol factor (1%). As shown in Table , the average number concentrations of these factors showed large decreases during APEC, except for the secondary aerosol factor. In addition, PNC factors mainly from regional transportation showed much larger decreases (by 70%) during APEC, which indicates that regional emission controls exerted the strongest impact on the regional aerosol factor. However, those of local primary PNC factors showed significantly lower decreases ranging from 4 to 40%. It is interesting to note that the reductions of Traffic 1 and Traffic 3 (by 37–40%) were larger than that of Traffic 2 (by 21%). A likely explanation is that the restrictions on the number of vehicles on road were stricter in the urban area of Beijing (within the fourth ring road) during APEC. Thus, ‘fresh’ traffic emissions could be largely reduced at the receptor site (urban area), whereas a smaller reduction of traffic emissions outside of the urban area could be expected to result in less reduction of aged traffic emissions. The higher wind speed during APEC further favoured the transportation of aged traffic emissions to the receptor site. The bulk PNC composition showed a substantial change during APEC. For example, the contribution of regional aerosol decreased from 9% before APEC to 4% during APEC. Correspondingly, primary PNC factors such as aged traffic, cooking, and Combustion 1 showed elevated contributions to PNC. Our results have significant implications: they show that controlling secondary precursors over regional scales can reduce secondary aerosols substantially and hence mitigate air pollution in megacities. As previously discussed, the reduction of local primary emissions was significantly less than that of secondary aerosol during APEC; therefore, stricter control of local precursor source emissions is crucial for improving air quality in the future.
3.4. Case study of the evolution of pollution episodes
Two pollution episodes that showed relatively similar synoptic systems over a regional scale were selected to characterize the evolution of particle chemical composition, sources and size distribution before and during APEC. In addition, we applied an automatic algorithm to fit every measured PNSD using MATLAB (Hussein et al., Citation2005). The five-day evolution of a severe pollution episode before APEC was observed between 21 and 25 October, during which the average PM2.5 concentration increased from below 30 μg/m3 to above 300 μg/m3 (EP3 in Fig. e). As shown in Fig. c, the evolution of this episode can be classified into clean stage (EP3_C), growth stage (EP3_G) and peak stage (EP3_P). Continuous growth of the Aitken mode particles is clearly depicted by the evolution of the mean particle size (Fig. a), which increases from approximately 65 nm to approximately 120 nm, accompanied with the PM2.5 level increasing from 30 μg/m3 to above 300 μg/m3. The largest increase (of 180 μg/m3) in the particle mass concentration occurs from 06:00 to 22:00 on 24 October, when the mean size increases by 31 nm. The three-day evolution of a pollution episode during APEC was observed between 2 and 4 November, during which the average PM2.5 concentration showed a 40-fold increase from <5 to> 200 μg/m3 (EP 5 in Fig. e). Similar to the pollution episode before APEC, a continuous growth of the Aitken mode particles was observed during the growth stage (EP5_G); they increased from approximately 30 nm to approximately 120 nm as shown in Fig. d. During a typical pollution episode in fall in Beijing, Guo et al. (Citation2014) also found that the accumulation of particle mass concentration was accompanied by a continuous size growth from ultrafine particles. However, one contradiction was evident during the peak stages of pollution episodes in this study. For example, the mean size decreased from 114 nm at 16:00 to 78 nm at 21:00 in 25 October (EP3_P), and the mean size in EP5_P also decreased from 126 nm at 15:00 to 74 nm at 20:00 in 4 November. The different behaviour of particle mean size during peak stage of pollution episodes would be attributed to the enhanced local primary emissions as both of them occurred in the evening rush hours. It is interesting to note that PM2.5 is relatively stable in peak stage of pollution episode before APEC (EP3_P), whereas PM2.5 increased from 140 to 230 μg/m3 in peak stage of pollution episode during APEC (EP5_P). This kind of discrepancy would suggest that the formation mechanism and sources of pollution episode before and during APEC was changed due to the emission controls.
Fig. 6. Temporal evolutions of (a, d) PNSD and mean diameter (white dashed curve), (b, e) particle number concentration (PNC) factors and (c, f) PM1 species during the pollution episodes on 21–25 October and 2–4 November. The pie charts show (b, e) the average source contribution of PNC and (c, f) the average chemical composition of PM1 for each stage.
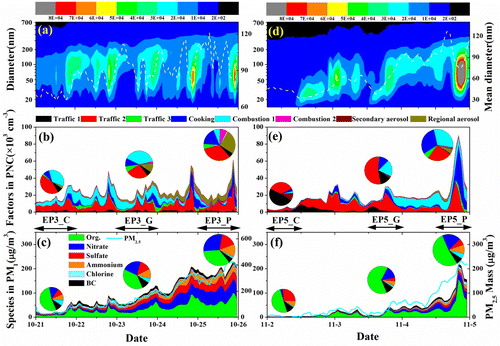
The evolution of pollution episodes can be characterized by variations in aerosol composition and sources at different stages (Table ). The aerosol composition during the clean stage in the pollution episode before APEC (EP3_C) was dominated by organics (53%) with a relatively smaller contribution from SIA (30%). The PNC showed dominant contributions from traffic (53%) and combustion (35%) sources, indicating that local sources played the most significant roles during this stage. The aerosol composition had a substantial change during the growth stage (EP3_G). The contribution of organics decreased to 38%, while those of SIA increased to 47%. Sources of PNC showed an increasing contribution from regional aerosols, which increased from 2% (EP3_C) to 10% (EP3_G), however, the dominant sources of PNC are still local sources, with the largest contributions coming from Combustion 1 (39%), followed by aged traffic emissions (26%). As this episode progressed (EP3_P), SIA exceeded organics and became the dominant component in PM1 (58%); in particular, nitrate accounted for nearly one-third of the total PM1 mass. These results highlight the enhanced roles of SIA in severe pollution episodes, which are consistent with the conclusions drawn in previous studies in China (Huang et al., Citation2014; Sun et al., Citation2014; CitationLiu, Hu, et al., 2016). In addition, regional aerosols showed an even larger contribution during this stage (25%), with decreasing contribution from combustion factors (15%). Our results suggest that regional transport plays a significant role in causing PM pollution during pollution episodes in Beijing. This is consistent with previous studies that show a large contribution of PM2.5 from regional transport during pollution episodes in Beijing (Streets, et al., Citation2007; Wang et al., Citation2014). The evolution of aerosol composition in the pollution episode during APEC was totally different from that of the pollution episode before APEC. As shown in Fig. f, organics dominated PM1 in the clean (50%, EP5_C), growth (64%, EP5_G) and peak (52%, EP5_P) stages, while SIA showed a minor and constant contribution in different stages. In addition, local sources (traffic emissions and Combustion 1) dominated PNC in the clean (86%, EP5_C), growth (88%, EP5_G) and peak (62%, EP5_P) stages, while regional aerosols contributed little to PNC during the evolution of pollution episode. These results suggest a significant reduction of regional pollution owing to regional emission controls during APEC, which largely decreased both the particle numbers and mass concentrations.
Table 4. The average number concentration of PNC factors and the average mass concentrations of PM1 species for each stage during pollution episodes before and during APEC (stages EP3_C, G, P, and EP5_C, G, P as marked in Fig. ).
Figure shows the evolution of size distributions of organics, sulphate and nitrate in pollution episodes before and during APEC. During the episode before APEC, sulphate and nitrate showed evident particle growth as the episode progressed, with peak diameters of which gradually evolved from 350 nm during EP3_C to larger than 700 nm during EP3_P. In addition, these size distributions of sulphate and nitrate during EP3_P were characterized by single large accumulation modes, indicating the ageing process of aerosol during the evolution of this episode (Liu, Hu, et al., Citation2016). Organics showed size evolution behaviour similar to that of sulphate and nitrate during the episode before APEC, although a significant contribution from particles smaller than 200 nm was also present for organics. In contrast, no obvious particle growth of organics, sulphate and nitrate was observed during the pollution episode during APEC. In addition, during the clean and growth stages, mass concentration of organics, sulphate and nitrate decreased in all particle diameters, from ultrafine mode to accumulation mode. During the peak stage, nitrate and sulphate also decreased considerably, whereas a significant increase of organics was observed (the mass concentration of which was even larger than during the episode before APEC). In addition, a smaller diameter mode of organics (approximately 420 nm) was observed in EP5_P compared to that of organics (approximately 620 nm) in EP3_P (Fig. ), suggesting the contribution of organics from ultrafine particles was increased in EP5_P. These results indicated that local sources probably contributed more to organics in the peak stage of pollution episode during APEC, as ultrafine particles primarily originating from local emission sources in urban locations (Canagaratna et al., Citation2004; Zhang et al., Citation2005). In fact, increasing contributions from combustion (32%) and traffic emissions (31%) were also resolved in this stage (Fig. e), suggesting that local sources played the most significant roles during this stage. Overall, the aerosol composition, sources and size distributions exhibited substantial changes during the evolution of the pollution episodes. The formation of the pollution episode before APEC was characterized by the significant enhancement of SIA and regional atmospheric transport, while the formation of the pollution episode during APEC was characterized by the significant enhancement of organics and local sources.
4. Conclusion
China imposed strict emission controls in Beijing and its surrounding regions during the 2014 APEC summit. In this study, we present a detailed investigation of the impacts of emission controls on changes in mass and number concentration, chemical composition and sources of fine particles. The average particle mass concentration showed a substantial decrease from 117.6 and 196.1 μg/m3 before APEC to 53.3 and 102.3 μg/m3 during APEC for PM2.5 and PM10, respectively. The aerosol chemical composition and number size distribution also showed significant changes. While submicron aerosols were composed mainly of organics (39.4%), followed by nitrate (25.0%) and sulphate (13.1%) before APEC, the contribution of organics increased to 49.5%, associated with a significant reduction of SIA during APEC. Correspondingly, the peak diameters of these species were larger before APEC, and the distribution of which appeared narrower than those during APEC. In addition, a clear decrease in accumulation mode (35.9%) and nucleation mode (29.5%) particles was found during APEC, whereas Aitken mode particles showed a much smaller reduction (19.1%), the concentration of which in the early morning (00:00–08:00) during APEC was approximately the same with that before APEC. These results suggest different responses of Aitken mode and accumulation mode particles to emission controls.
PMF analysis of PNC identified eight sources, including six primary factors (three traffic factors, one cooking factor and two combustion factors) and two secondary aerosol factors (secondary aerosol and regional aerosol). In combination the organic aerosol (OA) components with the PNSD data in PMF provide a more complete understanding of the sources of PNC in Beijing. Cooking factor and traffic factor that related to diesel trucks and heavy-duty vehicles emissions during night-time were firstly identified for PNC in Beijing. Traffic emission particles dominated PNC composition before APEC (51%), with ‘fresh’ traffic, aged traffic and traffic emissions from diesel trucks and heavy-duty vehicles accounting for 11, 35, and 5%, respectively. The combustion factors (29%) emerged as the second largest contributor to PNC, followed by the cooking factor (10%), regional aerosol factor (9%) and secondary aerosol factor (1%). The regionally transported aerosols decreased from 1.8 × 103 cm−3 before APEC to 0.6 × 103 cm−3 during APEC, the reduction of which was similar to those of SIA during APEC. In contrast, primary factors from traffic and local combustion sources presented much smaller decreases, with the reduction ranging from 4 to 40%. The elevated contributions of primary factors indicated the presence of strong local source emissions during APEC.
Finally, a comprehensive analysis is performed to illustrate the chemical and source evolution of aerosol in pollution events before and during APEC. During the evolution of pollution episodes, accumulation of the particle mass concentrations was accompanied by a continuous growth in size of the Aitken mode particles over several days, yielding numerous larger particles. At the same time, the peak diameters of SIA and organics gradually grew from approximately 350 nm to larger than 700 nm during the pollution episode before APEC, while a much smaller mass mode (below 500 nm) was observed during the polluted period during APEC. Our results suggest that the formation of the pollution episode before APEC was characterized by the significant enhancement of SIA and regional atmospheric transport, while the formation of the pollution episode during APEC was characterized by the significant enhancement of organics and local sources. These results highlight the importance of regional atmospheric transport in the formation of severe pollution episodes in Beijing. At the same time, to further mitigate air pollution, stricter emission controls on local source emissions are needed.
Disclosure statement
No potential conflict of interest was reported by the authors.
Funding
This study supported by the ‘Strategic Priority Research Program’ of the Chinese Academy of Sciences [grant number XDB05020000], [grant number XDA05100100] and the National Natural Science Foundation of China [grant number 41230642].
Supplementary Figure 6
Download EPS Image (339 KB)Supplementary Figure 5
Download EPS Image (312.2 KB)Supplementary Figure 4
Download EPS Image (2.2 MB)Supplementary Figure 3
Download Zip (120.1 KB)Supplementary Figure 2
Download EPS Image (165 KB)Supplementary Figure 1
Download EPS Image (186.8 KB)Acknowledgements
We thank Dr Pusheng Zhao of the Institute of Urban Meteorology, China Meteorological Administration for providing the observation data of particle number size distribution. We acknowledge the referees for the constructive suggestions.
References
- Al-Dabbousa, A. N. and Kumar, P. 2015. Source apportionment of airborne nanoparticles in a Middle Eastern city using positive matrix factorization. Environ. Sci.: Processes Impacts 17, 802–812.
- Ban-Weiss, G. A., Lunden, M. M., Kirchstetter, T. W. and Harley, R. A. 2010. Size-resolved particle number and volume emission factors for on-road gasoline and diesel motor vehicles. J. Aerosol Sci. 41, 5–12. DOI: 10.1016/j.jaerosci.2009.08.001.
- Breitner, S., Liu, L. Q., Cyrys, J., Brüske, I., Franck, U. co-authors. 2011. Sub-micrometer particulate air pollution and cardiovascular mortality in Beijing, China. Sci. Total Environ. 409, 5196–5204. DOI: 10.1016/j.scitotenv.2011.08.023.
- Brines, M., Dall’Osto, M., Beddows, D.C.S., Harrison, R. M., Gómez-Moreno, F. and co-authors. 2015. Traffic and nucleation events as main sources of ultrafine particles in high-insolation developed world cities. Atmos. Chem. Phys. 15, 5929–5945. DOI: 10.5194/acp-15-5929-2015.
- Brines, M., Dall’Osto, M., Beddows, D.C.S., Harrison, R. M. and Querol, X. 2014. Simplifying aerosol size distributions modes simultaneously detected at four monitoring sites during SAPUSS. Atmos. Chem. Phys. 14, 2973–2986. DOI: 10.5194/acp-14-2973-2014.
- Brock, C. A., Washenfelder, R. A., Trainer, M., Ryerson, T. B., Wilson, J. C. and co-authors. 2002. Particle growth in the plumes of coal-fired power plants. J Geophys. Res. 107(D12), AAC 9-1–AAC 9-14. DOI: 10.1029/2001JD001062.
- Buonanno, G., Ficco, G. and Stabile, L. 2009. Size distribution and number concentration of particles at the stack of a municipal waste incinerator. Waste Manag. 29, 749–755. DOI: 10.1016/j.wasman.2008.06.029.
- Canagaratna, M. R., Jayne, J. T., Ghertner, D. A., Herndon, S., Shi, Q. and co-authors. 2004. Chase studies of particulate emissions from in-use New York City vehicles. Aerosol Sci. Tech. 38, 555–573. DOI: 10.1080/02786820490465504.
- Chan, C. K. and Yao, X. H. 2008. Air pollution in mega cities in China. Atmos. Environ. 42, 1–42. DOI: 10.1016/j.atmosenv.2007.09.003.
- Dall’Osto, M., Beddows, D.C.S., Pey, J., Rodriguez, S., Alastuey, A. and co-authors. 2012. Urban aerosol size distributions over the Mediterranean city of Barcelona, NE Spain. Atmos. Chem. Phys. 12, 10693–10707. DOI: 10.5194/acp-12-10693-2012.
- Dunn, M. J., Jiménez, J. L., Baumgardner, D., Castro, T., McMurry, P. H. and co-authors. 2004. Measurements of Mexico City nanoparticle size distributions: Observations of new particle formation and growth. Geophys. Res. Lett. 31, L10102. DOI: 10.1029/2004GL019483.
- Forster, P., Ramaswamy, V., Artaxo, P., Berntsen, T., Betts, R. and co-authors. 2007. Changes in atmospheric constituents and in radiative forcing. In: Climate Change 2007: The Physical Science Basis. Contribution of Working Group I to the Fourth Assessment Report of the Intergovernmental Panel on Climate Change (eds. S. Solomon, D. Qin, M. Manning, Z. Chen, M. Marquis, K. B. Averyt, M. Tignor and H. L. Miller). Cambridge University Press, Cambridge, United Kingdom and New York, NY, USA.
- Gao, J., Chai, F. H., Wang, T., Wang, S. L. and Wang, W. X. 2012. Particle number size distribution and new particle formation: New characteristics during the special pollution control period in Beijing. J. Environ. Sci. 24(1), 14–21. DOI: 10.1016/S1001-0742(11)60725-0.
- Guo, S., Hu, M., Zamora, M. L., Peng, J. F., Shang, D. J. and co-authors. 2014. Elucidating severe urban haze formation in China. Proc. Nat. Acad. Sci. U.S.A. 111(49), 17373–17378. DOI: 10.1073/pnas.1419604111.
- Han, S., Kondo, Y., Oshima, N., Takegawa, N., Miyazaki, Y. and co-authors. 2009. Temporal variations of elemental carbon in Beijing. J. Geophys. Res. 114, D23202. DOI: 10.1029/2009jd012027.
- Harris, S. J. and Maricq, M. M. 2001. Signature size distributions for diesel and gasoline engine exhaust particulate matter. J. Aerosol Sci. 32, 749–764. DOI: 10.1016/S0021-8502(00)00111-7.
- He, L. Y., Hu, M., Huang, X. F., Yu, B. D., Zhang, Y. H. and co-authors. 2004. Measurement of emissions of fine particulate organic matter from Chinese cooking. Atmos. Environ. 38, 6557–6564. DOI: 10.1016/j.atmosenv.2004.08.034.
- Huang, R. J., Zhang, Y., Bozzetti, C., Ho, K. F., Cao, J. J. and co-authors. 2014. High secondary aerosol contribution to particulate pollution during haze events in China. Nature 514, 218–222. DOI: 10.1038/nature13774.
- Huang, X. F., He, L. Y., Hu, M., Canagaratna, M. R., Sun, Y. and co-authors. 2010. Highly time-resolved chemical characterization of atmospheric submicron particles during 2008 Beijing Olympic Games using an Aerodyne High-Resolution Aerosol Mass Spectrometer. Atmos. Chem. Phys. 10, 8933–8945. DOI: 10.5194/acp-10-8933-2010.
- Hussein, T., DalMaso, M., Petaja, T., Koponen, I. K., Paatero, P. and co-authors. 2005. Evaluation of an automatic algorithm for fitting the particle number size distributions. Boreal Environ. Res. 10, 337–355.
- Hussein, T., Karppinen, A., Kukkonen, J., Härkönen, J., Aalto, P. P. and co-authors. 2006. Meteorological dependence of size fractionated number concentrations of urban aerosol particles. Atmos. Environ. 40, 1427–1440. DOI: 10.1016/j.atmosenv.2005.10.061.
- Hussein, T., Puustinen, A., Aalto, P. P., Mäkelä, J. M., Hämeri, K. and co-authors. 2004. Urban aerosol number size distributions. Atmos. Chem. Phys. 4, 391–411. DOI: 10.5194/acp-4-391-2004.
- Janhäll, S., Andreae, M. O. and Pöschl, U. 2010. Biomass burning aerosol emissions from vegetation fires: Particle number and mass emission factors and size distributions. Atmos. Chem. Phys. 10, 1427–1439. DOI: 10.5194/acp-10-1427-2010.
- Kittelson, D. B., Watts, W. F. and Johnson, J. P. 2006. On-road and laboratory evaluation of combustion aerosols – Part 1: Summary of diesel engine results. J Aerosol Sci. 37, 913–930. DOI: 10.1016/j.jaerosci.2005.08.005.
- Leitte, A. M., Schlink, U., Herbarth, O., Wiedensohler, A., Pan, X. C. and co-authors. 2011. Size-segregated particle number concentrations and respiratory emergency room visits in Beijing, China. Environ. Health Perspect. 119, 508–513. DOI: 10.1289/ehp.1002203.
- Li, X., Wang, J., Tu, X., Liu, W. and Huang, Z. 2007. Vertical variations of particle number concentration and size distribution in a street canyon in Shanghai, China. Sci. Total Environ. 378, 306–316. DOI: 10.1016/j.scitotenv.2007.02.040.
- Lim, H. J. and Turpin, B. J. 2002. Origins of primary and secondary organic aerosol in Atlanta: Results of time-resolved measurements during the Atlanta supersite experiment. Environ. Sci. Technol. 36(21), 4489–4496. DOI: 10.1021/es0206487.
- Liu, J., Mauzerall, D. L., Chen, Q., Zhang, Q., Song, Y. and co-authors. 2016. Air pollutant emissions from Chinese households: A major and underappreciated ambient pollution source. Proc Natl. Acad. Sci. U.S.A. 113, 7756–7761. DOI: 10.1073/pnas.1604537113.
- Liu, L. Q., Breitner, S., Schneider, A., Cyrys, J., Brüske, I. and co-authors. 2013. Size-fractioned particulate air pollution and cardiovascular emergency room visits in Beijing, China. Environ. Res. 121, 52–63. DOI: 10.1016/j.envres.2012.10.009.
- Liu, Z. R., Hu, B., Liu, Q., Sun, Y. and Wang, Y. S. 2014. Source apportionment of urban fine particle number concentration during summertime in Beijing. Atmos. Environ. 96, 359–369. DOI: 10.1016/j.atmosenv.2014.06.055.
- Liu, Z. R., Hu, B., Zhang, J. K., Yu, Y. C. and Wang, Y. S. 2016. Characteristics of aerosol size distributions and chemical compositions during wintertime pollution episodes in Beijing. Atmos. Res. 168, 1–12. DOI: 10.1016/j.atmosres.2015.08.013.
- Liu, Z. R., Wang, Y. S., Hu, B., Ji, D. S., Zhang, J. K. and co-authors. 2016. Source appointment of fine particle number and volume concentration during severe haze pollution in Beijing in January 2013. Environmental Science and Pollution Research 23, 6845–6860. DOI: 10.1007/s11356-015-5868-6.
- Molina, M. J. and Molina, L. T. 2004. Megacities and atmospheric pollution. J. Air Waste Manage. Assoc. 54, 644–680. DOI: 10.1080/10473289.2004.10470936.
- Mönkkönen, P., Koponen, I. K., Lehtinen, K. E. J., Hämeri, K., Uma, R. and co-authors. 2005. Measurements in a highly polluted Asian mega city: Observations of aerosol number size distribution, modal parameters and nucleation events. Atmos. Chem. Phys. 5, 57–66. DOI: 10.5194/acp-5-57-2005.
- Nishita, C., Osada, K., Matsunaga, K. and Iwasaka, Y. 2007. Number-size distributions of free tropospheric aerosol particles at Mt. Norikura, Japan: Effects of precipitation and air mass transportation pathways. J. Geophys. Res. 112, D10213. DOI: 10.1029/2006JD007969.
- Norris, G., Vedantham, R., Wade, K., Brown, S., Prouty, J. and co-authors. 2008. EPA Positive Matrix Factorization (PMF)3.0 Fundamentals User Guide. Washington, DC: US Environmental Protection Agency, Office of Research and Development.
- Ntziachristos, L., Ning, Z., Geller, M. D. and Sioutas, C. 2007. Particle concentration and characteristics near a major freeway with heavy-duty diesel traffic. Environ. Sci. Technol. 41, 2223–2230. DOI: 10.1021/es062590s.
- Paatero, P. 1997. Least squares formulation of robust nonnegative factor analysis. Chemom. Intell. Lab. 37, 23–35. DOI: 10.1016/S0169-7439(96)00044-5.
- Paatero, P. and Tapper, U. 1994. Positive matrix factorization: A non-negative factor model with optimal utilization of error estimates of data values. Environmetrics 5, 111–126. DOI: 10.1002/(ISSN)1099-095X.
- Reche, C., Querol, X., Alastuey, A., Viana, M., Pey, J., and co-authors. 2011. New considerations for PM, Black Carbon and particle number concentration for air quality monitoring across different European cities. Atmos. Chem. Phys. 11, 6207–6227. DOI: 10.5194/acp-11-6207-2011.
- Rönkkö, T., Lähde, T., Heikkilä, J., Pirjola, L., Bauschke, U. and co-authors. 2013. Effects of gaseous sulphuric acid on diesel exhaust nanoparticle formation and characteristics. Environ. Sci. Technol. 47, 11882–11889. DOI: 10.1021/es402354y.
- Ruths, M., von Bismarck-Osten, C. and Weber, S. 2014. Measuring and modelling the local-scale spatio-temporal variation of urban particle number size distributions and black carbon. Atmos. Environ. 96, 37–49. DOI: 10.1016/j.atmosenv.2014.07.020.
- See, S. W., Balasubramanian, R. and Wang, W. 2006. A study of the physical, chemical, and optical properties of ambient aerosol particles in Southeast Asia during hazy and nonhazy days. J. Geophys. Res. 111, D10S08. DOI: 10.1029/2005JD006180.
- Seinfeld, J. H. and Pandis, S. N. 2006. Atmospheric Chemistry and Physics: From Air Pollution to Climate Change Wiley, New York, NY.
- Shi, J. P., Evans, D. E., Khan, A. A. and Harrison, R. M. 2001. Sources and concentration of nano-particles (<10 nm diameter) in the urban atmosphere. Atmos. Environ. 35(7), 1193–1202. DOI: 10.1016/S1352-2310(00)00418-0.
- Streets, D. G., Fu, J. S., Jang, C. J., Hao, J. M., He, K. B. and co-authors. 2007. Air quality during the 2008 Beijing Olympic Games. Atmos. Environ. 41, 480–492. DOI: 10.1016/j.atmosenv.2006.08.046.
- Sun, Y. L., Chen, C., Zhang, Y. J., Xu, W. Q., Zhou, L. B. and co-authors. 2016. Rapid formation and evolution of an extreme haze episode in Northern China during winter 2015. Sci. Rep. 6, 27151. DOI: 10.1038/srep27151.
- Sun, Y. L., Jiang, Q., Wang, Z., Fu, P., Li, J. and co-authors. 2014. Investigation of the sources and evolution processes of severe haze pollution in Beijing in January 2013. J. Geophys. Res. Atmos. 119, 4380–4398. DOI: 10.1002/2014jd021641.
- Tang, G., Zhu, X., Hu, B., Xin, J., Wang, L. and co-authors. 2015. Impact of emission controls on air quality in Beijing during APEC 2014: Lidar ceilometer observations. Atmos. Chem. Phys. 15, 12667–12680. DOI: 10.5194/acp-15-12667-2015.
- Ulbrich, I. M., Canagaratna, M. R., Zhang, Q., Worsnop, D. R. and Jimenez, J. L. 2009. Interpretation of organic components from positive matrix factorization of aerosol mass spectrometric data. Atmospheric Chem. Phys. 9, 2891–2918. DOI: 10.5194/acp-9-2891-2009.
- Viana, M., Kuhlbusch, T. A. J., Querol, X., Alastuey, A., Harrison, R. M. and co-authors. 2008. Source apportionment of particulate matter in Europe: A review of methods and results. J. Aerosol Sci. 39, 827–849. DOI: 10.1016/j.jaerosci.2008.05.007.
- von Bismarck-Osten, C., Birmili, W., Ketzel, M., Massling, A., Petäjä, T. and co-authors. 2013. Characterization of parameters influencing the spatio-temporal variability of urban particle number size distributions in four European cities. Atmos. Environ. 77, 415–429. DOI: 10.1016/j.atmosenv.2013.05.029.
- Vu, T. V., Delgado-Saborit, J. M. and Harrison, R. M. 2015. Review: Particle number size distributions from seven major sources and implications for source apportionment studies. Atmos. Environ. 122, 114–132. DOI: 10.1016/j.atmosenv.2015.09.027.
- Wang, X., Westerdahl, D., Wu, Y., Pan, X. C. and Zhang, K. M. 2011. On-road emission factor distributions of individual diesel vehicles in and around Beijing, China. Atmos. Environ. 45, 503–513. DOI: 10.1016/j.atmosenv.2010.09.014.
- Wang, Z. B., Hu, M., Sun, J. Y., Wu, Z. J., Yue, D. L. and co-authors. 2013. Characteristics of regional new particle formation in urban and regional background environments in the North China Plain. Atmos. Chem. Phys. 13, 12495–12506. DOI: 10.5194/acp-13-12495-2013.
- Wang, Z. F., Li, J., Wang, Z., Yang, W. Y., Tang, X. and co-authors. 2014. Modeling study of regional severe hazes over mid-eastern China in January 2013 and its implications on pollution prevention and control. Sci. China Earth Sci. 57(1), 3–13. DOI: 10.1007/s11430-013-4793-0.
- Watson, J. G. 2002. Visibility: Science and regulation. J. Air Waste Manage. Assoc. 52, 628–713. DOI: 10.1080/10473289.2002.10470813.
- Wehner, B., Birmili, W., Ditas, F., Wu, Z., Hu, M. and co-authors. 2008. Relationships between submicrometer particulate air pollution and air mass history in Beijing, China. Atmos. Chem. Phys. 8, 6155–6168. DOI: 10.5194/acp-8-6155-2008.
- Wehner, B., Birmili, W., Gnauk, T. and Wiedensohler, A. 2002. Particle number size distributions in a street canyon and their transformation into the urban-air background: Measurements and a simple model study. Atmos. Environ. 36, 2215–2223. DOI: 10.1016/S1352-2310(02)00174-7.
- Wehner, B., Uhrner, U., Von Löwis, S., Zallinger, M. and Wiedensohler, A. 2009. Aerosol number size distributions within the exhaust plume of a diesel and a gasoline passenger car under on-road conditions and determination of emission factors. Atmos. Environ. 43, 1235–1245. DOI: 10.1016/j.atmosenv.2008.11.023.
- Wehner, B. and Wiedensohler, A. 2003. Long term measurements of submicrometer urban aerosols: Statistical analysis for correlations with meteorological conditions and trace gases. Atmos. Chem. Phys. 3, 867–879. DOI: 10.5194/acp-3-867-2003.
- Willeke, K. and Baron, P. A. 1993. Aerosol measurement principles, techniques, and applications Van Nostrand Reinhold, Hoboken, NJ.
- Woo, K. S., Chen, D. R., Pui, D. Y. H. and McMurry, P. H. 2001. Measurement of Atlanta aerosol size distributions: Observations of ultrafine particle events. Aerosol Sci. Technol. 34(1), 75–87. DOI: 10.1080/02786820120056.
- Wu, Z., Hu, M., Liu, S., Wehner, B., Bauer, S. and co-authors. 2007. New particle formation in Beijing, China: Statistical analysis of a 1-year data set. J. Geophys. Res. 112, D09209. DOI: 10.1029/2006JD007406.
- Wu, Z., Hu, M., Pen, L., Liu, S., Wehner, B. and co-authors. 2008. Particle number size distribution in the urban atmosphere in Beijing. China. Atmos. Environ. 42(34), 7967–7980. DOI: 10.1016/j.atmosenv.2008.06.022.
- Xu, W. Q., Sun, Y. L., Chen, C., Du, W., Han, T. T. and co-authors 2015. Aerosol composition, oxidation properties, and sources in Beijing: Results from the 2014 Asia-Pacific Economic Cooperation summit study. Atmos. Chem. Phys. 15, 13681–13698. DOI: 10.5194/acp-15-13681-2015.
- Yao, X. H., Chan, C. K., Fang, M., Cadle, S., Chan, T. and co-authors. 2002. The water-soluble ionic composition of PM2.5 in Shanghai and Beijing, China. Atmos. Environ. 36, 4223–4234. DOI: 10.1016/S1352-2310(02)00342-4.
- Yu, J. H., Guinot, B., Yu, T., Wang, X. and Liu, W. Q. 2005. Seasonal variations of number size distributions and mass concentrations of atmospheric particles in Beijing. Adv. Atmos. Sci. 22(3), 401–407.
- Yue, D. L., Hu, M., Wu, Z. J., Wang, Z. B., Guo, S. and co-authors. 2009. Characteristics of aerosol size distributions and new particle formation in the summer in Beijing. J. Geophys. Res. 114, D00G12. DOI: 10.1029/2008JD010894.
- Zhang, J. K., Wang, L. L., Wang, Y. H. and Wang, Y. S. 2016. Submicron aerosols during the Beijing Asia-Pacific Economic Cooperation conference in 2014. Atmos. Environ. 124, 224–231. DOI: 10.1016/j.atmosenv.2015.06.049.
- Zhang, Q., Canagaratna, M. R., Jayne, J. T., Worsnop, D. R. and Jimenez, J. L. 2005. Time- and size-resolved chemical composition of sub-micron particles in Pittsburgh: Implications for aerosol sources and processes. J. Geophys. Res. 110, D07S09, DOI: 10.1029/2004JD004649.
- Zhang, R., Jing, J., Tao, J., Hsu, S. C., Wang, G., and co-authors. 2013. Chemical characterization and source apportionment of PM2.5 in Beijing: Seasonal perspective. Atmos. Chem. Phys. 13, 7053–7074. DOI: 10.5194/acp-13-7053-2013.
- Zhou, L.M., Kim, E., Hopke, P. K., Stanier, C. O. and Pandis, S. N. 2005. Mining airborne particulate size distribution data by positive matrix factorization. J. Geophys. Res. 110, D07S19. DOI: 10.1029/2004JD004707.
- Zhu, Y., Hinds, W. C., Kim, S., Shen, S. and Sioutas, C. 2002. Study of ultrafine particles near a major highway with heavy-duty diesel traffic. Atmos. Environ. 36, 4323–4335. DOI: 10.1016/S1352-2310(02)00354-0.