ABSTRACT
A series of novel complexes of La(III) with substituted pyrazolines and mixed ligand complexes with these pyrazolines and thio-ligands have been synthesized and characterized by elemental analysis, molecular weight measurement, Fourier transform infra-red, 1H and 13C NMR spectroscopy. The particle/crystallite sizes were confirmed by powder X-ray diffraction, scanning electron microscopy and transmission electron microscopic techniques. Optical characterizations have been performed by electronic absorption and fluorescence emission spectroscopies in order to investigate their luminescence behaviour, which reveals fairly good quantum yield and fluorescence life time for some of the complexes. Thermal analyses such as thermogravimetric and differential thermal analysis indicate that these complexes are thermally stable at high temperature. Investigations pertaining to solid-state optical absorption spectra and photoelectron yield spectroscopy reveal that upon thermal annealing beyond 300°C, these compounds form dark-brown to black semiconducting materials with an optical band gap between 1.9 and 2.3 eV.
Abbreviations: La-PyH : La-HPY = [La(C15H12N2OH)2(H2O)Cl]; La-PyH-dtc = [La(C15H12N2OH)(C5H10NS2)(2H2O)Cl]; La-OCPy-xan: [La(C15H12N2OOCH3)(C2H5OCS2)(2H2O)Cl]
1. Introduction
Conceiving the importance of lanthanides in different fields, such as organic light emitting diodes (OLEDs), liquid crystals, fluoroimmunoassays, laser technology, optical telecommunication systems [Citation1,Citation2], there are much concern about the study of the lanthanide complexes. One of the early lanthanide elements, lanthanum, is itself used in the field of ceramics, thermal stability, solar energy, catalysis, photostrictor, dye sensitizers, scintillators, optoelectronic and photonic studies [Citation3–12]. Due to wide applications in the field of luminescence [Citation13–15], lanthanum is attracting the attention in the photo-physical research area. The term photo-physical is mainly concerned with the absorption, excitation and emission processes [Citation16,Citation17], which leads to the luminescence characteristics. There are so many literature present on the luminescent/photo-physical behaviour of pyrazoline itself [Citation18–28]. Reported results reveal that the pyrazoline ligands offer wide-range applications such as light attenuation in scintillators, emission of blue fluorescence upon UV light excitation and fluorescence probe for metal ion detection by harnessing their luminescence behaviour [Citation29–32]. Having strong fluorescence property, the pyrazoline can be widely used as brighteners, chemosensors, OLEDs, and so on [Citation24,Citation31,Citation33–38]. Due to this unique fluorescent property, pyrazoline can also be used in cell biology as an emissive fluorescent probe [Citation39]. By having high quantum yield, pyrazoline can also act as a good fluorophore [Citation40,Citation41]. Pyrazoline rings are also capable of influencing photo-physical properties of lanthanides [Citation20] resulting in the antenna effect in the complexes [Citation42]. Considering these important properties of pyrazolines, we have chosen it as the main ligand of our complexes. Due to the high affinity of lanthanum (hard acid), towards the O-donor ligand (hard base) [Citation43], it was easy to proceed the reaction with pyrazolines in comparison to the S-donor (soft base) ligands such as sodium diethyldithiocarbamate and potassium ethylxanthate. Our motive is to study the coordination behaviour and effect of the surrounding environment on the luminescence behaviour of complexes. In continuation of our previous work [Citation44–46], we have investigated the complexation behaviour of various substituted pyrazolines and thio-ligands with lanthanum(III) followed by the characterization of the resulting complexes by various analytical as well as photo-physical techniques. Investigation of their luminescence behaviour was conducted utilizing electronic absorption, fluorescence emission and fluorescence life-time studies. Apart from this, some of the complexes have also been subjected to optoelectronic characterizations including the estimation of their optical band gap and energy of their highest occupied molecular orbital (HOMO) and lowest unoccupied molecular orbital (LUMO) in order to explore their potential for opto-electronic device applications.
2. Experimental
All the solvents (benzene, methanol, ethanol, and so on) were rigorously dried and purified by standard methods before use [Citation47]. The chemicals used were of analytical grade. Lanthanum chloride heptahydrate (S.D. Fine, India), diethyldithiocarbamate (S.D. Fine, India), o-hydroxyacetophenone (CDH, India) and benzaldeydes (Merck, India) were used as received. Pyrazolines and potassium ethylxanthate were prepared by the reported method [Citation48,Citation49]. All lanthanum complexes were synthesized by the typical conventional method.
2.1. Synthesis of [La(C15H12N2OX)2Cl.nH2O]
The complexes of lanthanum (III) with 3(2-hydroxy-phenyl)-5-(4-substituted phenyl) pyrazolines with the general formula [La(C15H12N2OX)2Cl·nH2O], (where X = H, OCH3, CH3 and Cl) were prepared by the reaction of pyrazoline ligand with lanthanum chloride in the 1:2 molar ratio as per the following reaction:
Freshly cut pieces of sodium were taken with an excess of isopropanol and refluxed for 30 min until a clear solution of sodium isopropoxide was obtained. The benzene solution of pyrazoline was then added in the content and the reaction mixture was further refluxed for 30 min, whereby a constant yellow colour solution was obtained. The reaction mixture was cooled to room temperature and then the methanolic solution of metal chloride was added with constant stirring. The reaction mixture was further stirred at room temperature for about 2 h, till the colour of the reaction mixture underwent a change from yellow to yellowish brown. The reaction mixture was filtered to remove precipitated NaCl. The solvent was removed under reduced pressure from the filtrate and the yellow-coloured solid thus was obtained. In this manner, Compounds 1–4 were prepared and their analytical details pertaining to the elemental analysis and physical characterizations are given in Tables and , respectively.
Table 1. Elemental analysis data of the complexes.
Table 2. Physical characterization data of the complexes under investigation.
2.2. Synthesis of [La(C15H12N2OX)(C5H10NS2)Cl.nH2O]
The mixed ligand complexes of the type [La(C15H12N2OX)(C5H10NS2)Cl.nH2O] were prepared by the following two steps:
Methanolic solution of lanthanum chloride was added to benzene solution of pyrazoline and refluxed it for about 6 h. The colour of the solution became yellow. The reaction mixture was cooled at room temperature. Now, the methanolic solution of sodium diethyldithiocarbamate was added, and the content was stirred at room temperature for about 4 h. The solvent was reduced in vacuum to ensure good yield and purity, and it was finally filtered. The yellow-coloured solid was washed with water and alcohol and dried. This general procedure was adopted to synthesize the four mixed ligand complexes (Compounds 5–8) having their analytical details as shown in Tables and .
2.3. Synthesis of [La(C15H12N2OX)(C2H5OCS2)Cl·nH2O]
The mixed ligand complexes of the type [La(C15H12N2OX)(C2H5OCS2)Cl·nH2O] were prepared by the following two steps:
Methanolic solution of metal chloride was added to the benzene solution of pyrazoline and refluxed it for about 6 h. The colour of the solution became dark yellow. After cooling at room temperature, the methanolic solution of potassium ethylxanthate was added and the content was stirred at room temperature for about 4 h. The solvent was reduced in vacuum to ensure good yield and purity and finally it was filtered. The brownish-yellow-coloured solid obtained was washed with water and alcohol and finally dried. Following this general method, four mixed ligand complexes (Compounds 9–12) were prepared and their analytical and physical characterization details are given in Tables and .
3. Characterization details
For determination of molecular weights and isotopic studies, the molecular ion peak is detected by direct analysis in real time (DART) mass spectra on a JMS-T100LC Accu TOF mass spectrometer. The accelerating voltage was 10 kV and spectra were recorded at room temperature. Elemental analysis (C, H, N and S) were carried out on Elementar Vario EL III C, H, N, S analyzer. Oxygen was analysed by the EuroVector elemental analyzer. Chlorine was estimated by Volhard’s method [Citation50]. Lanthanum was estimated as La2O3. FTIR spectra were recorded on a Thermo Nicolet (Model: 6700) spectrophotometer in the range 5000–50 cm−1. Scanning electron microscopy (SEM) studies have been carried out on JEOL Model JSM-6390LV at magnification from 5× to 300,000×. Powder X-ray diffraction (PXRD) studies were carried out on the advance X-ray diffractometer (Bruker AXS D8) in the temperature range from −170°C to + 450°C. Thermogravimetric analysis (TGA) and differential thermal analysis (DTA) for the compounds have been carried out using the Perkin Elmer thermal analysis system both in the presence as well as the absence of an inert atmosphere. Luminescence studies have been done by a Jobin Yvon Fluorolog-3-11 spectrofluorimeter with a xenon source lamp at 450 W, in the 200–700 nm range. Life-time measurement was carried out on the Jobin Vyon fluorocube, having a pulsed-diode excitation source in dichloromethane solution media. Electronic absorption spectra in solution (in dichloromethane) were recorded in a Varian, Cary 5000 UV-visible-NIR spectrophotometer within the range 200–1000 nm. For the solid-state electronic absorption spectral measurement, a thin film of the complex from their dichloromethane solution was cast on the glass followed by drying under vacuum. Spectra were recorded for the film before and after their post-heating at high temperature (300°C). Optical band gap (Eg) was calculated from the solid-state electronic absorption spectra, while ionization potential to determine the energy level of the HOMO was conducted using photoelectron yield spectroscopy (KV-200, Bunkokeiki, Japan).
4. Results and discussion
Complexes of the type [La(C15H12N2OX)2Cl.nH2O] are yellow-coloured solids, while mixed ligand complexes represented by the general formula [La(C15H12N2OX)(C5H10NS2)Cl·nH2O] and [La(C15H12N2OX)(C2H5OCS2)Cl·nH2O] are yellowish-brown solids. All the complexes synthesized for the present investigation are non-hygroscopic, stable in air and soluble in solvents such as dichloromethane, dimethylformamide. Molecular weight measurements show that these complexes are monomeric in nature. A perusal of the elemental analysis data for C, H, N, S, O, Cl and La reveals that they are in well agreement with the proposed stoichiometry as shown in . The molecular structure along with the coordination modes for some the representative lanthanum complexes have been shown in Figure .
Figure 1. The proposed structure of the lanthanum complex (a) [La(C15H12N2OH)2(H2O)Cl] and mixed ligand lanthanum complexes (b) [La(C15H12N2OH)(C5H10NS2)(2 H2O)Cl] and (c) [La(C15H12N2OOCH3)(C2H5OCS2)(2 H2O)Cl].
![Figure 1. The proposed structure of the lanthanum complex (a) [La(C15H12N2OH)2(H2O)Cl] and mixed ligand lanthanum complexes (b) [La(C15H12N2OH)(C5H10NS2)(2 H2O)Cl] and (c) [La(C15H12N2OOCH3)(C2H5OCS2)(2 H2O)Cl].](/cms/asset/a6d32b04-0a04-43ed-b68e-1031e8a51f64/tusc_a_1516028_f0001_ob.jpg)
4.1. Fourier transform infra-red spectral analysis
The FTIR spectral analysis for these lanthanum (III) complexes summarized in exhibits vibration bands appearing at 3400–3332, 1642–1610, 480–450, 400–360, 350–320 cm−1 are assigned to ν(N–H), ν(C=N), ν(La–O), ν(La–N) and ν(La–S), respectively [Citation51–60]. A broad band appearing between 3433 and 3410 cm−1 for La-PyH, La-PyH-dtc and La-OCPy-xan has been assigned to ν(O–H) [Citation58,Citation61]. All these infra-red (IR) band peaks are presented in . The appearance of ν(O–H) stretching band suggests the presence of water in these complexes which is also in accordance with weight loss shown by TGA.
Table 3. FTIR spectral data of the complexes (in cm−1).
4.2. Nuclear magnetic resonance spectroscopic characterization
4.2.1. Proton nuclear magnetic resonance
The proton nuclear magnetic resonance (1H NMR) spectra of these complexes have been recorded in deuterated chloroform (CDCl3) exhibiting the characteristic signals. Aromatic protons of pyrazolines were observed as a complex pattern in the region δ 7.88–6.01 ppm. The peak observed in the range δ 4.67–4.02 ppm is due to the hydroxyl proton in different complexes for protons of the water molecule [Citation62]. The appearance of peak at δ 4.93–4.80 ppm could be assigned to the N–H group indicating non-involvement of the –NH group [Citation63]. The skeletal protons of five-membered rings are observed at δ 3.72–3.31 ppm as a triplet. The peak at δ 3.76–3.13 could be assigned to –CH and –CH2 groups. The peak at δ 1.50–1.40 ppm indicates the presence of –CH3 [Citation44,Citation64]. The –OCH3 peak of La-OCPy-xan complex δ 3.52–3.57 also confirms the identity of this compound. The proton NMR spectral data for all of the 12 complexes under investigation have been summarized in .
Table 4. 1H NMR analytical data for the complexes in CDCl3.
4.2.2. Carbon nuclear magnetic resonance studies
The proton decoupled carbon nuclear magnetic resonance (13C NMR) spectra show the presence of all the important signals with reference to pyrazoline, diethyldithiocarbamate and ethylxanthate. The signals observed in the region δ 136.23–124.50 ppm as a complex pattern could be assigned to aromatic carbon atoms [Citation63]. The signals observed at δ 154.96–151.34 ppm due to imino carbon of C = N has been found to be shifted downfield as compared to the spectrum of free pyrazoline (δ 143.50 ppm), suggesting the involvement of imino nitrogen in the complex formation via coordination. The peak at δ 159.42–157.22 ppm could be assigned due to the > C = S group of diethyl dithiocarbamate and ethyl xanthate ligands. The peak at δ 77.68–76.98 ppm is due to C–O, indicating the complexation of oxygen to metal. The peaks due to –CH and –CH2 are observed at δ 62.87–28.87 ppm. The peak at δ 14.30–11.23 ppm could be assigned due to the –CH3 group of pyrazoline, diethyldithiocarbamate and ethylxanthate. Peak at δ 55.54–55.19 ppm is due to the –OCH3 group. The 13C NMR spectral data of all the complexes have been summarized in .
Table 5. 13C NMR spectral data for all of the complexes in the CDCl3.
4.3. Thermal analysis
TGA is a versatile tool to not only investigate the thermal stability of the material under investigation but it also provides vital information regarding nature of the molecules by weight loss as a function of temperature analysis. TGA thermograms for various complexes of type [La(C15H12N2OX)2Cl·nH2O], [La(C15H12N2OX)(C5H10NS2)Cl·nH2O] and [La(C15H12N2OX)(C2H5OCS2)Cl·nH2O] have been measured from 40°C to 730°C (non-inert atmosphere) not only to study their thermal stability but also to identify coordination moieties as proposed in the molecular structure shown in Figure . Weight loss of 2.74% at 214°C for complex 1 is attributed to the loss of one coordinated water molecule. The next weight loss of 5.41% at temperature 238°C is due to the loss of chlorine atom from the complex. Decomposition of the organic moiety is done at a temperature of 558°C. All the four complexes of the type [La(C15H12N2OX)2Cl·nH2O] follow the same decomposition pattern. Similarly, the weight can be seen in mixed ligand complexes in three consecutive steps, i.e. loss of coordinated water and chlorine atom in one step, decomposition of the thio-ligand in the second step and the last step leads to the decomposition of coordinated pyrazoline moiety. All the mixed ligand complexes follow the same order of decomposition. Figure shows the complete pyrolysis behaviour of these lanthanum complexes in air atmosphere. Thermal decomposition pattern of analysis for complexes with respect to temperature is shown in where the predicted weight losses are in good agreement with the experimentally observed values.
Figure 2. TGA thermogram of [La(C15H12N2OH)(C5H10NS2) (2 H2O)Cl], measured from room temperature to 730°C in air.
![Figure 2. TGA thermogram of [La(C15H12N2OH)(C5H10NS2) (2 H2O)Cl], measured from room temperature to 730°C in air.](/cms/asset/df0afd19-0702-4025-bcb4-ea695b9dc734/tusc_a_1516028_f0002_oc.jpg)
Among 12 lanthanum complexes synthesized in this study, three representative complexes having molecular structures shown in Figure were subjected for the thermal studies utilizing TGA and DTA under an inert (N2 flow rate 10 ml/min) atmosphere from room temperature to 500°C and the results are shown in Figure . It can be clearly seen from this figure that the mixed ligand complex La-OCPy-xan exhibits relatively much higher thermal stability under an inert atmosphere having a total weight loss 32% up to 500°C where DTA indicates clear structural changes in the temperature ranges of 350–450°C. This total 32% weight loss is associated with loss of two coordinated water, one chlorine atom and xanthate molecules as predicted in . On the other hand, La-PyH and La-PyH-dtc exhibit rather sharp weight loss of 24% and 38% in the temperature range of 350–500°C, respectively, which is well reflected and in DTA thermograms shown in Figure (b).
Figure 3. TGA (a) and DTA (b) thermograms for three representative La-complexes measured in an inert (N2) atmosphere.
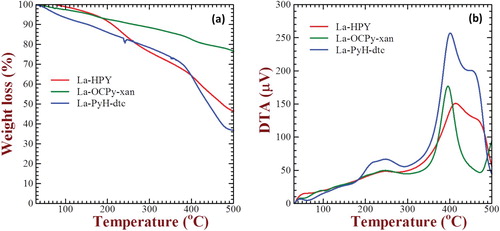
Table 6. Thermogravimetric analysis of some representative complexes, where measurement was taken in a non-inert (air) atmosphere.
4.4. PXRD, SEM and TEM microstructural investigations
The PXRD, SEM and transmission electron microscopic (TEM) investigations were done to observe the nature, particle/crystallite size and the surface morphology of the complexes. The PXRD peaks were indexed by EXPO2014 program. From this programme, the space groups, Schoenflies notation/point group, unit cell value and the crystal system were obtained. The complex La-PyH has space group P4132, Schoenflies notation “O” and unit cell value 9.93, whereas the complex La-PyH-dtc shows the Pn-3n space group, “Oh” Schoenflies notation and unit cell value 10.23. Similarly, the complex La-OCPy-xan have the space group I-43 m with point group “Td” and the unit cell value 10.37. All these complexes have the cubic crystal system. From the appearance of PXRD peaks, all the complexes seem to be amorphous in nature. A slight shift in the 2θ position of lanthanum, with respect to the literature [Citation65,Citation66], indicates the formation of the complex. The average crystallite size obtained by the Scherer equation [Citation67–71] reveals that all these complexes are nano sized (Supporting information S1).
SEM images signify the surface morphology of these lanthanum complexes. According to SEM images, the complex La-PyH has micro granule-like structure at its surface, which is in the form of agglomerates. The complex La-PyH-dtc and La-OCPy-xan show globular structures with some agglomeration. The SEM pictures show that the particle sizes are somewhat larger than the PXRD peak analysis, this is due to molecular association facilitated by the intermolecular hydrogen bonding. From Figure , it is clearly seen that the complexes La-PyH and La-OCPy-xan have the nano size, whereas the complex La-PyH-dtc is micron sized.
Figure 4. SEM for complexes (a) [La(C15H12N2OH)2(H2O)Cl], (b) [La(C15H12N2OH)(C5H10NS2)(2 H2O)Cl], (c) [La(C15H12N2OOCH3) (C2H5OCS2)(2 H2O)Cl].
![Figure 4. SEM for complexes (a) [La(C15H12N2OH)2(H2O)Cl], (b) [La(C15H12N2OH)(C5H10NS2)(2 H2O)Cl], (c) [La(C15H12N2OOCH3) (C2H5OCS2)(2 H2O)Cl].](/cms/asset/0303685b-948b-4524-a0b7-4e9dc1ec54c5/tusc_a_1516028_f0004_oc.jpg)
The TEM images, as shown in Figure , also confirm that these particles are in the nano range. According to TEM, the sizes of particles are comparatively higher than the sizes obtained from PXRD peak analysis. The difference in sizes can be justified by the fact that the Scherer equation gives the crystallite size value, whereas the TEM images indicate the sizes of particles. Since the particle is made up of so many crystallites, therefore it is obvious that the particle size should be larger than the crystallite sizes [Citation72]. All these average values obtained by the PXRD peak analysis, SEM and TEM images are given in .
Figure 5. TEM images for complexes (a) [La(C15H12N2OH)2(H2O)Cl], (b) [La(C15H12N2OH)(C5H10NS2)(2 H2O)Cl], (c) [La(C15H12N2OOCH3)(C2H5OCS2)(2 H2O)Cl].
![Figure 5. TEM images for complexes (a) [La(C15H12N2OH)2(H2O)Cl], (b) [La(C15H12N2OH)(C5H10NS2)(2 H2O)Cl], (c) [La(C15H12N2OOCH3)(C2H5OCS2)(2 H2O)Cl].](/cms/asset/a7ac91c4-5eaf-4368-baad-317b7098394a/tusc_a_1516028_f0005_ob.jpg)
Table 7. PXRD, SEM and TEM analysis data for some of the representative complexes.
4.5. Opto-electronic characterization
Electronic absorption spectra of two representative lanthanum complexes synthesized in this work such as La-PyH-dtc and La-OCPy-xan in the dimethylformamide solution are shown in Figure (a). La-PyH-dtc and La-OCPy-xan exhibit main electronic absorption peaks at 300 and 325 nm, respectively, and are associated with the intra-ligand π–π* electronic transition of the pyrazoline ligand [Citation57,Citation58]. A bathochromic red-shift (25 nm) observed for the La-OCPy-xan as compared to that of the La-PyH-dtc could be attributed to the presence of the electron donating methoxy group in the pyrazoline ligand. In order to explore the application of these complexes for device applications, two of the complexes having fairly good solubility in the chloroform solution namely La-PyH and La-PyH-dtc were utilized for the fabrication of thin films on the glass substrates followed by heating at 300°C for 30 min under an inert atmosphere. Solid-state electronic absorption spectra of La-PyH and La-PyH-dtc before and after thermal annealing are shown in Figure (b). A perusal of the absorption spectra of La-PyH-dtc in solution and solid state as shown in Figure (a,b), respectively, reveals a clear red-shift of about 50 nm in the optical absorption edge which is attributed to the enhanced intermolecular association due to inter-ligand π–π interactions. At the same time, upon thermal annealing, there is even further red-shift in the optical absorption especially in the visible region suggesting the formation of new type of complexes after the removal of coordinated water and chlorine along with the dithiocarbamate ligands. This was further confirmed by clear changes in the colour of the complexes from yellow to brown by naked eye after the thermal annealing.
Figure 6. Electronic absorption spectra of representative La-complexes in chloroform solution (a) and film casted on glass before and after heating at 300°C for 30 min (b).
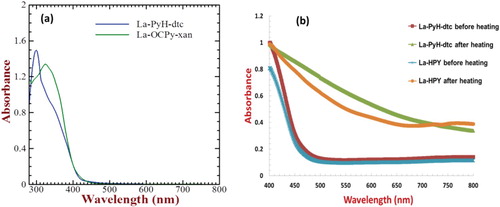
This visible light absorption of the complexes after thermal annealing interested us to have an in-depth insight about the nature of these complexes after thermal annealing. Visible light absorption indicates the lower band gap (Eg) of the complexes from the insulator (wide Eg) to the semiconductor, indicating their potentiality as a visible light absorbing complex or as a semiconductor. The Tauc plot represented by plotting (αhν)2 as a function of photon energy obtained from the solid-state electronic absorption spectrum has been widely used for the estimation of optical Eg of semiconducting materials [Citation73]. The Tauc plot for the thin films of La-PyH-dtc and La-PyH on glass after the thermal annealing has been shown in Figure (a). Optical band gap estimated from the Tauc plot for La-PyH-dtc and La-PyH suggests that these complexes are moderate gap semiconductors having Eg of 1.90 and 2.31 eV, respectively. In order to utilize a material for the solid-state device applications such as either active semiconducting component or semiconducting light absorber (sensitizer), they must also fulfil the requirements of forming energetic cascade with the other device component materials. Taking this point into consideration, ionization potential measurement using photoelectron yield spectroscopy (PYS) was also conducted in order to estimate the energy of their valance band or HOMO. PYS measurement conducted for La-PyH and La-PyH-dtc after thermal annealing provided their HOMO energy to beat −5.39 eV and −5.32 eV, respectively. Energy of the conduction band or LUMO was estimated from the relation LUMO = HOMO + Eg and calculated to be −3.69 and −3.08 eV, respectively, utilizing the respective Eg obtained from the Tauc plot. This has helped us to construct the energy band diagram of the lanthanum complexes under investigation along with the well-known wide band gap semiconductor TiO2 which has been shown in Figure (b) [Citation74]. A perusal of this figure clearly indicates that lanthanum complexes such as La-PyH and La-PyH-dtc form energetic cascade and matching TiO2 and can be used as a sensitizer for this. At the same time for the solid-state, p–n junction-type applications, their p-type semi-conductor nature has to be verified by the Hall-effect measurement and needs further in-depth investigation and is beyond the scope of the work presented here and will be published in future.
4.6. Luminescence and life-time study
As discussed earlier, lanthanum complexes La-PyH, La-PyH-dtc and La-OCPy-xan exhibit absorption maximum in the wavelength region of 280–325 nm. It has been found that all of these compounds exhibit the fluorescence emission with emission maximum from 430 to 570 nm in the visible region of the solar spectrum. On the basis of fluorescence analyses, the quantum yield (φ) for the ligands as well as lanthanum complexes was calculated and it has been found to be between 0.50 and 0.74. Dynamics of the fluorescence decay has also been investigated for the representative lanthanum complexes such as La-PyH, La-PyH-dtc and La-OCPy-xan and their fluorescence decay curves are shown in Figure . The optical properties of the ligands in terms of absorption and emission maximum, Stoke-shift, quantum yield and fluorescence life time are summarized in . A perusal of Figure and indicates that complexes under investigation show relatively moderate fluorescence life life (τ) in nanosecond time domain (Supporting information S2). At the same time, mixed ligand lanthanum complexes such as La-PyH-dtc and La-OCPy-xan exhibit much improved life time as compared to the lanthanum complex bearing only the pyrazoline ligand (La-PyH). This could be associated with the very large Stoke-shift (>2 times) in the La-PyH as compared to that of La-PyH-dtc and La-OCPy-xan. A large Stoke-shift suggests higher flexibility for the conformational changes in the excited state providing alternate decay pathways. It can be clearly seen from that newly synthesized complexes are luminescence active and provide luminescence to the complexes also [Citation75]. At the same time, it can be seen to form the luminescence behaviour of ligands and their respective complexes, it has been found that the complex La-PyH (having only the pyrazoline ligand) has a lower quantum yield, whereas the mixed ligand complexes La-PyH-dtc and La-OCPy-xan (having pyrazoline and thio-ligands) are found to have a higher quantum yield. It was found that that pyrazoline ligand exhibits hampered fluorescence property upon complexation with lanthanum, while there was enhanced luminescence when the metal complexation of pyrazoline occurs with the thio-ligands.
Figure 8. Emission spectra of complexes: (a) [La(C15 H12N2OH)2(H2O)Cl], (b) [La(C15H12N2OH)(C5H10NS2)(2 H2O)Cl], (c) [La(C15H12N2OOCH3)(C2H5OCS2)(2 H2O)Cl].
![Figure 8. Emission spectra of complexes: (a) [La(C15 H12N2OH)2(H2O)Cl], (b) [La(C15H12N2OH)(C5H10NS2)(2 H2O)Cl], (c) [La(C15H12N2OOCH3)(C2H5OCS2)(2 H2O)Cl].](/cms/asset/dcf0e184-cf34-4096-9314-9225a476a048/tusc_a_1516028_f0008_oc.jpg)
Table 8. Quantum yield and life-time analysis for the ligands and some of the representative lanthanum complexes used in this work.
From , it can also be seen that the complex La-PyH, having the lowest quantum yield as comparable to ligand pyrazoline and other mixed ligand complexes, liberates its 50% absorbed energy in the form of light and rest as heat. Due to high Stoke-shift of these complexes, the fluorescence resonance energy transfer/Forster resonance energy transfer (FRET) mechanism occurs which leads to quenching of fluorescence [Citation76–78]. The FRET mechanism also reveals that with the presence of an acceptor, the life time decreases [Citation79], which is clearly seen in . While the mixed ligand complexes have the antenna effect [Citation80] and thus showing a high quantum yield and life time, which releases most of its energy in the form of light.
5. Conclusions
In this work, a series of novel mixed ligand complexes of lanthanum(III) of the type [La(C15H12N2OX)2(H2O)Cl], [La(C15H12N2OX)(C5H10NS2)(2 H2O)Cl] and [La(C15H12N2OX)(C2H5OCS2)(2 H2O)Cl] have been successfully synthesized. The IR spectral data indicate the bidentate mode of coordination of pyrazoline, dithiocarbamate and xanthate ligands. NMR confirms the proposed structure of the complexes. The PXRD, SEM and TEM studies indicate that they are amorphous solids consisting of small nanoparticles which make larger particles due inter-molecular hydrogen bond formation. DART mass spectrometry shows the monomeric nature of these complexes. Putting all facts together, the most possible geometry around the lanthanum(III) atom is octahedron (Figure (a)), pentagonal bipyramidal or trigonal prismatic (Figure (b), (c)). Optoelectronic characterizations reveal that they exhibit a moderate optical band gap of 1.90–2.31 eV and exhibit energetic cascade with the TiO2 which is most widely used in the wide band gap semiconductor. Their energetics and band gap indicate that they can be used as a sensitizer for TiO2 or as an active semiconductor material for device applications. It has also been demonstrated that La-PyH (having only nitrogen and oxygen donor ligand) has a lower value of Φ as compared to that of La-PyH-dtc and La-OCPy-xan (having nitrogen, oxygen and sulphur donor ligands).
Supplemental Material
Download MS Word (372.8 KB)Acknowledgements
The authors are grateful to SAIF, CDRI, Lucknow, India, SAIF, IIT Madras, India, STIC, Cochin, India, for providing the necessary spectral and analytical data. They are also thankful to Department of Chemistry, D. D. U. Gorakhpur University, Gorakhpur, India, for providing the laboratory and library facilities. Finally, they are thankful to Prof. Shuzi Hayase, Graduate School of LSSE, Kyushu Institute of Technology, Japan, for the support regarding measurement of PYS and solid-state electronic absorption spectra.
Disclosure statement
No potential conflict of interest was reported by the authors.
ORCID
Iffat Ameen http://orcid.org/0000-0003-0077-1203
Abhishek Kumar Tripathi http://orcid.org/0000-0003-1210-9516
Afshan Siddiqui http://orcid.org/0000-0003-4858-399X
Gaurav Kapil http://orcid.org/0000-0002-5066-4978
Shyam S. Pandey http://orcid.org/0000-0001-8102-1003
Additional information
Funding
References
- Desreux JF, Choppin GR, Bunzli JC. Lanthanide probes in life, chemical and earth sciences. Amsterdam: Elsevier; 1989.
- Guo L, Wu S, Zeng F, et al. Synthesis and fluorescence property of terbium complex with novel Schiff-base macromolecular ligand. Eur Polym J. 2006;42:1670–1675. doi: 10.1016/j.eurpolymj.2006.01.025
- Sasidharan S, Komban R, Nambiar S, et al. Sol-gel materials for energy, environment and electronic applications. Cham: Springer International Publishing AG; 2017.
- Cao X, Vassen R, Fischer W, et al. Lanthanum–cerium oxide as a thermal barrier coating for high temperature applications. Adv Mater. 2003;15:1438–1442. doi: 10.1002/adma.200304132
- Sani E, Mercatelli L, Meucci M, et al. Lanthanum hexaboride for solar energy applications. Sci Rep. 2017;7:1–7. doi: 10.1038/s41598-017-00749-w
- LeVan T, Che M, Tatibouet JM. Effect of strontium doping on catalytic behaviour of lanthanum oxide on oxidative coupling of methane. Catal Lett. 1992;14:321–329. doi: 10.1007/BF00769670
- Nonaka K, Akiyama M, Xu CN, et al. Enhanced photovoltaic response in lead lanthanum zirconate-titanate ceramics with A-site deficient composition for photostrictor application. Jpn J Appl Phys. 2000;39:5144–5145. doi: 10.1143/JJAP.39.5144
- Iltis A, Mayhugh MR, Menge P, et al. Lanthanum halide scintillators: properties and applications. Nucl Instrum Methods Phys Res Sect A. 2006;563:359–363. doi: 10.1016/j.nima.2006.02.192
- Evans NA, Leaver IH. Dye sensitized photooxidation of 1,3-diphenyl-2-pyrazoline. Aust J Chem. 1974;27:1797–1803. doi: 10.1071/CH9741797
- Evans NA. Dye-sensitized photooxidation of some substituted 1,3-diphenyl-2-pyrazolines. Aust J Chem. 1975;28:433–437. doi: 10.1071/CH9750433
- Davidson RS, Pratt JE. An example of how photophysical properties can influence the magnitude of solvent isotope effects upon photo-oxidation reactions. J Photochem Photobiol. 1984;40:23–28. doi: 10.1111/j.1751-1097.1984.tb04548.x
- Marina OA, Canfield NL, Stevenson JW. Thermal, electrical, and electrocatalytical properties of lanthanum-doped strontium titanate. Solid State Ionics. 2002;149:21–28. doi: 10.1016/S0167-2738(02)00140-6
- Akbar R, Baral M, Kanungo BK. Experimental and theoretical approach of photophysical properties of lanthanum(III) and erbium(III) complexes of tris(methoxy-methyl)-5-oxine podant. Spectrochim Acta Part A. 2014;129:365–376. doi: 10.1016/j.saa.2014.03.045
- Jennings JJ, Bhatt CP, Franz AK. Lanthanum(III)-catalyzed three-component reaction of coumarin-3-carboxylates for the synthesis of indolylmalonamides and analysis of their photophysical properties. J Org Chem. 2016;81:6211–6222. doi: 10.1021/acs.joc.6b00541
- Mahata P, Ramya KV, Natarajan S. Pillaring of CdCl2-like layers in lanthanide metal-organic frameworks: synthesis, structure and photophysical properties. Chem Eur J. 2008;14:5839–5850. doi: 10.1002/chem.200800240
- Bäuerle D. Laser processing and chemistry. Berlin: Sprin-ger-Verlag; 2011.
- Fox RB. Photophysical processes and their role in polymer photochemistry. Washington (DC): Naval Research Laboratory.
- Lin J, Rivett DE, Wilshire JFK. The preparation and photochemical properties of some 1,3-diphenyl-2-pyrazolines containing a heteroaromatic substituent. Aust J Chem 1977;30:629–637. doi: 10.1071/CH9770629
- Moura NMM, Faustino MAF, Neves MGPMS, et al. Novel pyrazoline and pyrazole porphyrin derivatives: synthesis and photophysical properties. Tetrahedron. 2012;68:8181–8193. doi: 10.1016/j.tet.2012.07.072
- Fahrni CJ, Yang L, VanDerveer DG. Tuning the photoinduced electron-transfer thermodynamics in 1,3,5-triaryl-2-pyrazoline fluorophores: X-ray structures, photophysical characterization, computational analysis, and in vivo evaluation. J Am Chem Soc 2003;125:3799–3812. doi: 10.1021/ja028266o
- Wilkinson F, Kelly GP, Michael C, et al. A study of the photophysical properties of various triaryl-2-pyrazolines in solution and microcrystalline form. J Photochem Photobiol A: Chem. 1990;52:309–320. doi: 10.1016/1010-6030(90)80009-M
- Xiao D, Xi L, Yang W, et al. Size-tunable emission from 1,3-diphenyl-5-(2-anthryl)-2-pyrazoline nanoparticles. J Am Chem Soc. 2003;125:6740–6745. doi: 10.1021/ja028674s
- Singh P, Negi JS, Singh K, et al. Synthesis and structure dependent photophysical properties of novel 2-pyrazolines. Synth Met. 2012;162:1977–1980. doi: 10.1016/j.synthmet.2012.09.006
- Sandler SR, Tsou KC. Fluorescence spectral study of wavelength shifters for scintillation plastics. J Chem Phys. 1963;39:1062–1067. doi: 10.1063/1.1734359
- Sandler SR, Loshaek S, Broderich E, et al. 1,3,5-Triaryl-2-pyrazolines as wave length shifters in scintillation plastics. J Chem Phys 1962;66:404–408. doi: 10.1021/j100809a006
- Neunhoeffer O, Rosahl D. About the fluorescence of organic compounds upon excitation by X-rays. Z Elektrochem. 1953;57:81–87.
- Rivett DE, Rosevear K, Wilshire JFK. The preparation and spectral properties of some monosubstituted 1,3,5-triphenyl-2-pyrazolines. Aust J Chem 1976;32:1601–1612. doi: 10.1071/CH9791601
- Strahle M, Seitz W, Gusten M. Substituent effects on the fluorescence of l,3-diphenyl-2-pyrazoline. Naturforsch Teil B. 1976;31:1248. doi: 10.1515/znb-1976-0917
- Leggate P, Owen D. The fluorescence and scintillation properties of new oxazoles. Oxadiaz Pyrazolines Mol Crystals. 1968;4:357–373. doi: 10.1080/15421406808082923
- Hasan A, Abbas A, Akhtar MN. Synthesis, characterization and fluorescent property evaluation of 1,3,5-triaryl-2-pyrazolines. Molecules. 2011;16:7789–7802. doi: 10.3390/molecules16097789
- Wang P, Onozawa-Komatsuzaki N, Himeda Y, et al. 3-(2-Pyridyl)-2-pyrazoline derivatives: novel fluorescent probes for Zn2+ ion. Tetrahedron Lett. 2001;42:9199–9201. doi: 10.1016/S0040-4039(01)01970-0
- Zhen-Bo D, Lee ST, Li-Chun C, et al. Electroluminescence of polymer doped with triphenyl-2-pyrazoline. Acta Phys Sin. 1997;6:921–926.
- Dorlars A, Schellhammer CW, Schroeder J. Heterocycles as structural units in new optical brighteners. Angew Chemie. 1975;14:665–679. doi: 10.1002/anie.197506651
- Ma Z, Sonar P, Chen ZK. Recent progress in fluorescent blue light-emitting materials. Curr Org Chem. 2010;14:2034–2069. doi: 10.2174/138527210793351562
- Borsenberger PM, Schein LB. Hole transport in 1-phenyl-3-((diethylamino)styryl)-5-(p-(diethylamino)phenyl)pyrazoline-doped polymers. J Phys Chem. 1994;98:233–239. doi: 10.1021/j100052a039
- Wagner A, Schelhammer CW, Schroeder J. Aryl-Δ2-pyrazolines as optical brighteners. Angew Chem. 1966;5:699. doi: 10.1002/anie.196606991
- SarkerA K. Fluorescent whitening agents. Watford: Merrow; 1971.
- Venkataraman K. The chemistry of synthetic dyes. New York (NY): Academic Press; 1971.
- Mason WT. Fluorescent and luminescent probes for biological activity: a practical guide to technology for quantitative real-time analysis. San Diego (CA): Academic Press; 1999.
- Gusten H, Heinrich G, Bunsen-Ges B. Fluoreszenz von 4,4′-disubstituierten 1,3-diphenyl-2-pyrazolinen mit donator-akzeptor-substituenten. J Phys Chem. 1977;81:810–815.
- Rivett DE, Rosevear J, Wilshire JFK. The preparation and spectroscopic properties of some di- and tri-substituted 1,3,5-triphenyl-2-pyrazolines and related 2-pyrazolines. Aust J Chem. 1983;36:1649–1658. doi: 10.1071/CH9831649
- Carlos LD, Ferreira RAS, Bermudez VD, et al. Lanthanide-containing light-emitting organic–inorganic hybrids: a bet on the future. Adv Mater. 2009;21:509–534. doi: 10.1002/adma.200801635
- Huheey JE, Keiter EA, Keiter RL. Inorganic chemistry: principles of structure and reactivity. Delhi: Pearson Education; 2000.
- Tripathi UN, Bipin PP, Mirza R, et al. Synthesis and characterization of O,O′ dialkyl and alkylene dithiophosphates of lanthanum(III) and their adducts with nitrogen and phosphorus donor bases. J Coord Chem. 2002;55:1111–1118. doi: 10.1080/0095897021000026191
- Tripathi UN, Ahmad MS, Mirza R, et al. Synthesis and spectral characterization of O,O′- dialkyl and alkylene dithiophosphates of neodymium (III). Phosphorous Sulphur Silicon Relat. Elem. 2007;182:1779–1792. doi: 10.1080/10426500701313961
- Tripathi UN, Mirza R, Ahmad MS. Synthesis and characterization of samarium (III) tris(O,O′ -dialkyl and alkylene dithiophosphates) and their adducts with nitrogen and phosphorus donor bases. Phosphorous Sulphur Silicon Relat. Elem. 2007;182:1291–1305. doi: 10.1080/10426500601160827
- Vogel AI. A text book of quantitative organic analysis. London: ELBS and Longman; 1978.
- Drake JE, McDonald CLB, Kumar A, et al. The triethyl ammonium salt of O,O′-bis(o-tolyl) dithiophosphate [Et3NH]+[(2-MeC6H4O)2PS2]-. J Chem Crystallogr. 2005;35:447. doi: 10.1007/s10870-005-2218-4
- Zohir N, Mustapha B, Elbaki DA. Synthesis and structural characterization of xanthate (KEX) in sight of their utilization in the processes of sulphides flotation. J Miner Mater Charact Eng. 2009;8:469–477.
- Vogel AI. Textbook of qualitative inorganic analysis. London: ELBS and Longman; 1973.
- Tripathi UN, Venubabu G, Ahmad MS, et al. Synthesis, spectral and antimicrobial studies of diorganotin(IV)3(2′- hydroxyphenyl)-5-(4-substituted phenyl) pyrazolinates. J Appl Organomet Chem. 2006;20:669–676. doi: 10.1002/aoc.1074
- Tripathi UN, Venubabu G, Ahmad MS, et al. 3(2'-Hydroxyphenyl)-5-(4-Substituted phenyl) pyrazolinates of Tin (IV); synthesis and spectral characterization. Main Group Met Chem. 2006;29:39–46. doi: 10.1515/MGMC.2006.29.1.39
- Tripathi UN, Ahmad MS, Venubabu G, et al. Synthesis, spectral and antimicrobial studies of chlorodiorganotin(IV)[3(2′-hydroxyphenyl)-5-(4-substitutedphenyl) pyra-zolinates]. J Coord Chem. 2007;60:1777–1788. doi: 10.1080/00958970601183391
- Tripathi UN, Venubabu G, Ahmad MS. Synthesis, spectral and antimicrobial studies of triorganotin(IV) 3(2’-hydroxyphenyl)-5-(4-substituted phenyl) pyrazolinates. J Coord Chem. 2007;60:1709–1720. doi: 10.1080/00958970601110865
- Tripathi UN, Venubabu G, Ahmad MS. Synthesis, spectral characterization of chlorodiorganotin(IV) di[3(2’-hydroxyphenyl)-5-(4-substituted phenyl) pyrazolinates Turkish. J Chem 2007;31:45–54.
- Tripathi UN, Solanki JS, Bhardwaj A, et al. Synthesis, spectral study and antimicrobial activity of bismuth(III) 3(2’-hydroxyphenyl)-5-(4-substituted phenyl) pyrazolinates. J Coord Chem. 2008;61:4025–4032. doi: 10.1080/00958970802199964
- Tripathi UN, Ahmad MS, Solanki JS, et al. Synthesis, spectral study and antimicrobial activity of chloroantimony(III) 3(2’-hydroxyphenyl)-5-(4-substituted phenyl) pyrazolinates. J Coord Chem. 2009;62:636–644. doi: 10.1080/00958970802244976
- Sharma KV, Sharma V, Tripathi UN. Iron(III)5(2’-hydroxy-phenyl)-3-(4-X-phenyl)pyrazolinates and their addition complexes with N,P donor ligands: synthesis, spectral and antimicrobial investigation. J Coord Chem. 2008;61:3314–3328. doi: 10.1080/00958970802044160
- Ferraro JR. Low frequency vibrations of inorganic and coordination compounds. New York (NY): Plenum; 1971.
- Nakamoto K. Infrared spectra of inorganic and coordination compounds. New York (NY): Wiely-Interscience; 1970.
- Ameen I, Tripathi AK, Mishra RL, et al. A study on enhancing the quantum yield and antimicrobial activity of Pr(III) by varying the coordination environment. RSC Adv 2018;8:8412–8425. doi: 10.1039/C7RA13035J
- Liu XH, Pan H, Mazur P. Permeation and toxicity of ethylene glycol and methanol in larvae of Anopheles gambiae. J Exp Biol. 2003;206:2221–2228. doi: 10.1242/jeb.00420
- Silverstein RM, Webster FX. Spectrometric identification of organic compounds. New York (NY): Wiley; 1998.
- Tripathi UN, Bipin PP, Mirza R, et al. Synthesis and spectroscopic characterization of addition complexes of copper dithiophosphates with heterocyclic nitrogen donor bases. Polish J. Chem. 1999;73:1751–1756.
- Saravani H, Khajehali M. Synthesis and characterization of lanthanum oxide and lanthanumoxide carbonate nanoparticles from thermalizes of [La(acacen)(NO3)(H2O) complex. Oriental J Chem. 2015;31:2351–2357. doi: 10.13005/ojc/310464
- Zhang QB, Yang C, Hua YX, et al. Electrochemical preparation of nanostructured lanthanum using lanthanum chloride as a precursor in 1-butyl-3-methylimidazolium dicyanamide ionic liquid. Phys Chem Chem Phys. 2015;17:4701–4707. doi: 10.1039/C4CP05266H
- Ain Q, Pandey SK, Pandey OP, et al. Synthesis, spectroscopic, thermal and antimicrobial studies of neodymium(III) and samarium(III) complexes derived from tetradentate ligands containing N and S donor atoms. Spectrochim Acta Part A. 2015;140:27–34. doi: 10.1016/j.saa.2014.12.040
- Holzwarth U, Gibson N. The scherrer equation versus the ‘Debye-Scherrer equation. Nat Nanotechnol. 2011;6:534. doi: 10.1038/nnano.2011.145
- Rathore KS, Patidar D, Janu Y, et al. Structural and optical characterization of chemically synthesized ZnS nanoparticles. Chalcogenide Lett. 2008;5:105–110.
- Altomare A, Giacovazzo C, Guagliardi A, et al. New techniques for indexing: N-TREOR in EXPO. J Appl Crystallogr. 2000;33:1180–1186. doi: 10.1107/S0021889800006427
- Laugier J, Bochu B. LMGP-suite of programs for the interpretation of X-ray experiments. Saint Martin d’Hères: ENSP; 2002.
- Available from: http://prism.mit.edu/xray.
- Vigneshwaran M, Ohta T, Iikubo S, et al. Facile synthesis and characterization of sulfur doped Low bandgap bismuth based perovskites by soluble precursor route. Chem Mater. 2016;28:6436–6440. doi: 10.1021/acs.chemmater.6b02315
- Narayanaswamy K, Swetha T, Kapil G, et al. Simple metal-free dyes derived from triphenylamine for DSSC: a comparative study of two different anchoring group. Electrochim Acta. 2015;169:256–263. doi: 10.1016/j.electacta.2015.04.071
- Quici S, Cavazzini M, Marzanni G, et al. Visible and near-infrared intense luminescence from water-soluble lanthanide [Tb(III), Eu(III), Sm(III), Dy(III), Pr(III), Ho(III), Yb(III), Nd(III), Er(III)] complexes. Inorg Chem. 2005;44:529–537. doi: 10.1021/ic0486466
- Cheng PC. The contrast formation in optical microscopy. In: Handbook of biological confocal microscopy. New York (NY): Springer; 2006. p. 162–204.
- Helms V. Fluorescence resonance energy transfer, principles of computational cell biology. Weinheim: Wiley; 2008.
- Harris DC. Applications of spectrophotometry, quantitative chemical analysis. 8th ed. New York (NY): W. H. Freeman and Co; 2010.
- Clegg RM. Förster resonance energy transfer-FRET: what is it, why do it, and how it's done. In: Laboratory techniques in biochemistry and molecular biology. Vol. 33. Amsterdam: Elsevier; 2009. p. 1–57.
- Sabbatini N, Guardigli M. Luminescent lanthanide complexes as photochemical supramolecular devices. Coord Chem Rev. 1993;123:201–228. doi: 10.1016/0010-8545(93)85056-A