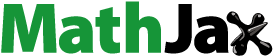
Abstract
In this work, Amberlite IRC-50 cation exchanger has been chemically functionalized with 8-hydroxyquinoline chelator to enhance its sorption capacity and selectivity toward transition metals. The resin characterized with X-ray photoelectron spectroscopy (XPS), attenuated total reflection infrared spectroscopy (ATR-IR), scanning electron microscope (SEM), and elemental analysis. Under optimum conditions, the sorption capacity values for Mn (II), Cu(II), Co(II) and Ni(II) ions have been increased by 50% or more in comparison with plain resin. The calibration curves for the studied ions were linear over the concentration range from 0 (blank) to 50 ng/ml. with R2 from 0.994–0.999. The LOD values for Mn(II), Cu(II), Co(II) and Ni(II) were 0.024, 0.035, 0.048, and 0.058 ng ml−1 respectively. The sorbent worked well as an efficient SPE material for the studied ions from groundwater real samples prior to their analysis by ICP-MS. The acceptable recovery and accuracy values prove its competence to extract these metals from groundwater matrices.
1. Introduction
The increased levels of metal ions in environment become a significant global dilemma [Citation1]. Various anthropogenic activities associated with industrial development, fossil fuels combustion, agricultural practices, mining works, as well as some natural process like atmospheric emissions are accountable for the release of heavy metals in the environment. Therefore, the monitoring of heavy /trace metals in environmental samples e.g. groundwater is essential to spot the occurrence of any significant variations [Citation2]. The progress in many technologies over the last decades steered the development of large number of sophisticated analytical instruments that facilitate the determination of heavy metals even at very low levels in wide range of matrices with high precision. Among these instruments are; atomic absorption spectrometry (AAS) [Citation3,Citation4], inductivety coupled plasma optical emission spectrometry (ICP-OES) [Citation5], and inductivety coupled plasma mass spectrometry (ICP-MS) [Citation6]. However, the accurate analysis of trace elements directly from complex matrices is too difficult because of their existence at low levels (e.g. in environmental samples) and the associated matrix interferences. Therefeore, the use of an appropriate sample preparation to clean up, isolate, and preconcentrate analytes, is usually prerequist prior to trace metals analysis [Citation7–11].
SPE is one of the best sample preparation approch for the extraction and preconcentration of inorganic and organic analytes from various matrices [Citation12–14]. The technique offers mnay advantages over classica sample preparation methods, such as flexibility, low cost, rapid extraction time, reduced solvent usage, simplicity, reusability of solid sorbents, ecologically-safety, and the enhanced preconcentration factor [Citation15]. In addition, it offers the potential to be hyphenated/integrated in an on-line mode with many analytical instruments [Citation14]. Forthermore, the availablity of enourmous selective sorbent materials from many commericial sources, makes SPE a highly selective procedure [Citation16,Citation17].
Conventitially, SPE materials are prepared either using physical (impregnation) or chemical linkage of cerian reactive moieties/functionality (e.g. chelating agent for metals) to a wide range of natural and pre synthesised solid supports. Among the favered preferred subtances are; silica gel [Citation18–20], activated carbon [Citation21,Citation22], chelating ion exchange resins [Citation23], naphthalene [Citation24], zeolite [Citation25], C18 [Citation26], cellulosic derivatives [Citation27], alumina [Citation28,Citation29], and XAD resins [Citation30]. Extensive overview with demonstrative examples of the diffrent preparation methods for chelating resins can be found in many comperhensive reviews [Citation30–32].
Principally, synthetic polymers are desired supporting matrices for the immobilisation of selective chelating agents for extration of trace elements due to their good physical properties, low cost and the aptness to be used over wide pH range, [Citation33]. Among these polymers, amberlite XAD (commercially available polystyrene resins) such as, XAD-1, XAD-2, XAD-4 and XAD-16, have atracted speceical attention due to thier large surface areas that can be exploited for the attachment of numerous chelating moieties [Citation34]. Good examples show most of the recently developed modification approaches for polystyrene polymers, particularly Amberlite XAD series are well precented in a recent review [Citation30].
Preparation of metal chelators from pre-synthesized polymers can be achieved throughout different chemical reactions sequences [Citation35,Citation36]. For example, chelating ligands are anchored onto the surface of PS–DVB copolymers via different bridging groups such as the methylene (-CH2-) spacer and azo (-N = N-). The nitration/diazotization route is a reliable modification protocol to functionalize Amberlite series and other polystyrene polymers with large number of chelating moieties. Essentially, the polymer substrates (beads) are treated with a mixture of HNO3 and H2SO2 to create nitro groups, which are subsequently reduced to form aromatic amines that are subsequently diazotized with chelating ligand. The chelating ligand separated from support by azo group spacer [Citation36]. Many examples demonstrating the eminent chemical modifications for polystyrene and alike polymeric substrates together with some of their applications in analytical sciences can be found in many reviews [Citation30–32].
The Amberlite IRC-50 resin is a weak acidic cation exchanger consisting of methacrylic acid-divinylbenzene copolymer with carboxylic function groups. It commercialized as white opaque beads of 16–50 mesh (0.33-0.50 mm) and occasionally it contains 43-53% of moisture [Citation37]. Extensive literature survey shows that most of the earlier chemical modifications reported for Amberlite IRC-50 have been carried out through carboxylic acid group, whereby the acid is converted into chlorocarboxylate resin by chlorinating agent to facilitate ligand coupling [Citation38,Citation39].
Hitherto, there is no published report on the chemical modification of Amberlite IRC-50 with 8-HQ or other oxins. Therefore, the aim of this research was to design a novel chelating resin encompassing 8-hydroxquinline moiety anchored onto the phenyl ring onto the backbone of Amberlite IRC-50 via nitration/ diazotization. Hence, the proposed resin postures both ion exchange and chelating properties with enhanced sorption capacity and selectivity.
2. Materials and methods
2.1. Chemical reagents
All chemicals used throughout this work were from analytical reagent grades. The Amberlite IRC-50 resin (16-50 mesh size) obtained from Merch, (Darmstadt, Germany). The chelating agent; 8-Hydroxyquinoline and ethanol were purchased from Scharlab (Barcelona, Spain), and hydrochloric acid from Philip Harris (Cheshire, UK). The standard and working solutions were prepared from stock solution (1 mg/ml) of Mn(II), Co(II), Ni(II), Cu(II), and the reducing agent; sodium hydrosulfite (Na2S2O4) obtained from Acros Organics (Geel, Belgium). The diazotizing agent; (NaNO2) was purchased from Lobal Cheme (Gujarat, India). Ammonium acetate, acetic acid and liquid ammonia solution which have been used to prepare buffer solution were from Sigma Aldrich, (St. Louis, MO, USA). The chelating resin: Chelex-100, which used for buffer purification obtained from BioRad (Hercules, CA, USA). To avoid contamination, all laboratory glassware that used in this work were soaked overnight in 5% nitric acid solution from Chem-Lab NV (Zedelgem, Belgium) the ultrapure water used throughout the work was produced from a Millipore Milli-Q water purification (Burlington, MA, USA).
2.2. Instrumentation and measurements
The SPE experiments were performed making use of a 12-way standard SPE manifold (Ato Science, China). The chelating resin packed into SPE cartridges (bond straight barrel; Agilent Technologies) to make diskettes of 5-10 mm height sandwiched between pair of porous Teflon filters. The SPE manifold was vacuumed using AP-9950 pump (Ato Science, China). The determination of metal ions performed using ICP-MS 7500 series (Agilent technologies, UAS). The digital pH meter model HI22111 pH/ORP meter (HANNA Instrument Company, (Leighton Buzzard, UK)) employed to check the pH of solutions. CHN elemental analysis was performed using a CHNSO Perkin Elmer 2400 Instrument (Waltham, MA, USA). SEM images were taken by a JSM-6380 LA scanning electron microscope (SEM ssx 550, Shimadzu, Kyoto, Japan). X-ray photoelectron spectroscopy (XPS) was applied using ESCALAB 250 XPS spectrometer (Thermo-Fisher Scientific, Waltham, MA, USA) and the spectra were obtained by energy source of Al Kα X-ray radiation (1361.6 ev of protons and Pass Energy 150.0 eV with a step size of 1.000 eV). ATR-IR spectra were recorded in the range of 200-4000 cm−1 on a Fourier transform infrared instrument, Thermo NICOLET 380 FTIR with attenuated total reflection (ATR) accessory (Thermo-Fisher Scientific, Waltham, MA, USA).
2.3. Preparation of 8-HQ-Amberlite IRC-50 chelating resin
The synthesis of 8-HQ-Amberlte IRC-50 chelating resin was undertaken according to the following reaction sequence;
First, 10.0 g of commercial Amberlite IRC-50 resin was activated using a mixture of ethanol, hydrochloric acid and water (2:1:1), and then washed several times with distilled water until the supernatant liquid become neutral to remove any impurities or activation mixture residual. Next, the activated resin was transferred into a mixture of concentrated nitric acid (10 ml) and concentrated sulfuric acid (25 ml) and was stirred constantly at 60°C for 1 h on a water bath for nitration. Then the nitrated mixture was filtered and washed thoroughly with distilled water to remove residual acids. Subsequently, the nitro groups on the surface of Amberlite IRC-50 were reduced (aminated) by the addition of sodium hydrosulfite (Na2S2O4) 5% (50 ml) in a locked flask at 45 °C for 24 h, to convert all -NO2 groups to -NH2. After that, the amino polymer was rinsed with cold distilled water in order to eliminate any retained regent [Citation22,Citation40,Citation41].
Upon the completion of amination step, and to prevent the degradation of the intermediates the product was cooled to temperature of 0–5 (±0.2) °C and treated with HCl (100 ml, 2M) for 30 min, washed with distilled water to eliminate any HCl residual, then suspended in ice-cold water (250 ml) and mixed with HCl (1M) and NaNO2 (2.0 ml) with continues stirring. After 10 min., 50 ml of 2% w/v 8-HQ solution in ethanol added dropwise to the mixture for 4 h to anchor the 8-HQ chelator to resin by diazotization. The resulting deep brown chelating resin was filtered, washed with ethanol and deionized water many times till the filtrate was colorless. Finally, the product has been dried under vacuum at 70°C for 3 h and kept in desiccator until use [Citation34,Citation42].
2.4. Extraction study
2.4.1. Batch method
The performance of the prepared chelating sorbent influenced by several factors including loading capacity, pH, the mass of sorbent, and contact time. Thus, these parameters were assessed using stagnant batch sorption experiments as described elsewhere [Citation43]. Concisely, 0.05 g of the dry chelator was transferred into 50 ml polyethylene sample tube and 10 ml solution contain 100 µg ml−1 of the metal ions (individually) prepared in ammonium acetate buffer (0.2 M, pH 6) was added. The solution was left over a mechanical shaker for 24 h at room temperature, and centrifuged (3500 rpm, 10 min) to separate chelator mass. Then, the quantity of metals remained in the supernatant solution were quantified with ICP-MS and compared to the initial concentration.
2.4.2. SPE procedure
The dynamic SPE experiments were performed using a conventional 12-way SPE apparatus. The SPE cartridges were packed with 500 mg of chelator that sandwiched between pair of porous filters. The SPE protocol executed as follow. Initially, 5 ml of buffer solution passed through the SPE cartridges at 1 ml/min flow rate for conditioning. Then, the anticipated volume of standards/sample solutions (i.e. 50 ml) were loaded through the cartridges at a flow rate of 0.5 ml/min. after that, the cartridges were flushed with 5 ml of distilled water to remove any unchelated metals residual or retained matrix components. Finally, the sequestered metal ions were eluted with HNO3 (5 ml, 1.5 M) at a flow rate of 2 ml/min and collected into 10 ml PTFE sample vials for the ICP-MS analyses. This SPE procedure has been employed for the standards, and the real samples through this work.
3. Results and discussion
3.1. Synthesis of 8-HQ-Amberlite IRC-50 chelator
The chemical attachment of 8-HQ chelator to Amberlite IRC-50 substrate was accomplished using the traditional nitration/diazotization protocol as schematically depicted in Figure . The process involves the creation of nitro groups onto the surface of Amberlite IRC-50 polymer using a mixture of nitric and sulfuric acids. The nitro groups are then reduced into amines with sodium hydrosulfite (Na2S2O4). Thus, the diazo coupling of 8-HQ chelator to the aminated Amberlite IRC-50 is realized. The development of the eminent deep brown colure of the final product indicates the completion of the modification process. However, for confirmation the resin has been characterized with ATR-IR, elemental analysis, XPS and SEM imaging.
3.2. Characterization of 8-HQ-Amberlite IRC-50 resin
3.2.1. ATR-IR
Attenuated total reflection infrared spectroscopy (ATR-IR) is an ideal means to identify the chemical structure of substances in powder form. Thus, it has been used in this work to confirm the attachment of 8-HQ moieties onto the Amberlite IRC-50 surface. The ATR-IR spectrum of bare Amberlite IRC-50 and 8-HQ-Amberlite IRC-50 chelator show characteristics common bands in both of them. The most shared peak observed at 1682 cm−1 which confirms the existence of carbonyl group (C = O) (Figure ). In addition, the stretched bands appeared at 2995, and 1558 cm−1 evident the existence of aromatic proton and carbon individually. The distinguished band seen in the region 3446 cm−1 is assigned for the –OH groups, which also clear in the spectrum of the bare and the functionalized resins.
Perhaps, the successfulness of immobilization of 8-HQ moieties on the resin surface can be confirmed from the characteristics bands shown at 1338, 1508 and 1634 cm−1 which proof the presence of (C–N), (N = N), and (C = N) respectively [Citation22,Citation44,Citation34]. This finding supports that 8-HQ ligand is effectively anchored onto Amberlite IRC-50 surface through diazotized (N = N) coupling.
3.2.1.1. Elemental analysis
The elemental analysis has been used to examine the success of chemical attachment of the 8-HQ chelating agent on the Amberlite resin by comparing the percentage of carbon, hydrogen, and nitrogen contents of modified and the plain Amberlite IRC-50. Obviously, the carbon ratio in the modified resin is relatively higher compared to its percentage in pure Amberlite IRC-50 Table . Remarkably, the appearance of nitrogen (2. 87%) in the case of the 8-HQ-Amberlite IRC-50 resin confirm that the 8-HQ functional groups were grafted onto the surface of resin [Citation45].
Table 1. percentage of CNH of the plan amberlite IRC-50 and 8-HQ-Amberlite IRC-50.
3.2.2. XPS
X-ray Photoelectron Spectroscopy (XPS) is a useful technique for surface analysis. It has been used to study the surface composition of Amberlite IRC-50 resins before and after modification with 8-HQ moieties. The spectra in Figures 3.1 and 3.2 display two dominant photoelectron lines for C1s, and O1s of the plain Ambrelite IRC-50 and C1s, O1s, and N1s of 8-HQ-Amberlite IRC-50. Figure .
XPS survey of Amberlite IRC-50 shows different peaks for C1s that could be referred to (C = C) and (COOH), and O1s peak that assign to (C = O) and (O-H). Apparently, 8-HQ-Amberlite IRC-50 shows a characteristic peak for N1s with a binding energy value 400.08 eV in addition to the distinguished C1s and O1s peaks which is ascribed to the presence of pyridinic nitrogen and N = N respectively [Citation45–48]. The appearance of the characteristic N1s peak in 8-HQ-Amberlite IRC-50 confirms the successful immobilization of 8-HQ onto amberlite surface. The results of XPS and the elemental analysis (section 3.2.1) indicated that 8-HQ is introduced successfully to the Amberlite IRC-50.
3.2.3. SEM
Scanning electron microscope (SEM) is a reliable analytical technique to examine topography and chemical structure of material surfaces. In this method, a focused beam of electrons is used to generate images for the investigated samples [Citation44]. The SEM analysis reveals a very valuable information about surface morphology and elemental composition. The SEM micrographs in Figure shows the morphological images of Amberlite IRC-50 polymer before and after the immobilization of 8-HQ. It can be observed that in plain resin the beads are clear and flat with no external surface coverage substance, while the modified resin displays notable change in surface morphology e.g. white rough spots covering part of the surface, which are expected to increase the active sorption area and make available more active sites for metal sorption. This signifies that the ligand loads onto the support. The difference of material topography was in consistent with former metal chelators reported elsewhere [Citation49,Citation50].
3.2.3.1. pH effect
The pH of solution has a potential effect on complexation process, and hence it determines the fraction of metal ion participate in the chelating interaction operation. Regularly, it is observed that the fraction of metal ions uptaken by chelating sorbent is increased as the solution shifted from acidic medium to neutral or alkaline one [Citation40]. From chemistry point of view, it is anticipated that metal sorption (chelation) commences when most of the acidic chelating sites start to replace hydronium ions (H+) with metals and the sorption capacity reaches the highest value at the pH at which the entire chelating sites take part in the chelating process [Citation22,Citation51]. In this work, the pH influence on the performance of metal chelator sorbent was investigated utilizing 0.2 M ammonium acetate buffer in the pH range 2 - 8. The Mn(II), Cu(II), Co(II), and Ni(II) metals ions were selected as demonstrating examples.
As shown in Figure , at low pH, minute recovery values for all ions were observed because the elevated concentration of hydrogen ions (H+) compete with metal ions, thus less metal ions could bind to the chelating sites. Therefore, the extraction efficiency is diminished. Meanwhile as the pH shifted to neutral medium (e.g. pH 6) the recovery values have increased steadily. This is probably taken place because the dissociation of chelating groups on the chelator enhance the tendency for retention of metal ions by complexation. However, the relatively slight decrease in extraction efficiency at higher pH, might ascribed to the precipitation of metals in form of M(OH) [Citation52].
Noticeably, The best recovery for Cu(II) was seen in the pH range 4–6. This finding coincident with result reported by other investigator for Amberlite XAD-16-HQ [Citation44]. However, it seems that the pH range 6–8 was the best for the recovery of the Mn(II) ion. Meanwhile, the maximum sorption for Co (II) and Ni(II) ions were occurred in the pH range from 5 to 7. The highest recovery for the investigated ions were seen at pH 6. At this pH value the recovered percentage for Mn(II), Co(II) and Ni(II) were 93.69, 105 and 115% in the same order.. Therefore, this pH value has been selected as an optimal value for SPE of these ions. Previous investigation showed that the optimum sorption foe Mn(II), Co(II), Ni(II) and Cu(II) ions by 8-hydroxyquinoline attached onto Amberlite XAD-2000 take place in the pH range 6–8 [Citation53].
3.3. Sorption capacity
The sorption capacity is a convenient measure for the performance of chelating resins. It is a useful evaluation method to estimate the amount of resin required to quantitatively extract the analyte from a given solution matrix. In this work, the resin static capacity was calculated for Mn(II), Co(II), Ni(II) and Cu(II) at pH 6. The variance in the concentration values (before/after) has been employed to compute sorption capacity using equation (Equation1(1)
(1) ) [Citation54].
(1)
(1)
Where SC is the batch sorption capacity in (mM g−1), C0 and Ce are the initial equilibrium concentrations of metal ion in µg ml−1. V is the volume of solution (ml), m is the quantity of chelator in (g), Mr is the relative atomic mass of investigated metal ion. The results were compared with values of sorption capacity of the bare Amberlite IRC-50 under the same circumstances.
As can be seen from values in Table , the sorption capacity for the modified resin; 8-HQ-AmberliteIRC-50 was enhanced by more than 50% in comparison to that of the plain resin for all the investigated metal ions. The observed significant enhancement in the values of sorption capacity of the functionalized Amberlite IRC-50 could be ascribed to the attachment of 8-HQ moieties onto the phenyl ring, besides the carboxylic acid groups on the original resin. As a result, the interaction of metal ions with chelating resin anticipated to take place by both ion exchange (Lewis acid) and a coordination mechanism. [Citation55,Citation56].
Table 2. sorption capacities (mMg−1) of modified and non-modified resins.
The sorption capacities for Mn(II),Co(II), Ni(II), and Cu(II) of this resin are compared with that obtained in previous studies for chelating sorbents in which the 8-HQ moiety has been used as a ligand immobilized on different supporting materials (Table ). The present resin shows comparable sorbent or higher sorption capacities for the studied metal ions. It showed a higher sorption capacity than the reported values for Amberlite XAD-4 [Citation55], XAD-4[Citation56], silica gel[Citation43], vinyl polymer [Citation57]. However, the higher sorption capacity (0.62 mM/g for Cu ion) that has been reported with 8-HQ cellulose chelator could be due to the large surface area of sorbent [Citation58].
Table 3. Comparison of sorption capacities (mM g−1).
3.4. Effect of chelator mass
The sorption process is highly influenced by the mass of sorbent. Herein, variable resin masses (0.1-1.0 g) of 8-HQ-Amberlite-IRC-50 resin used to examine the effect of sorbent mass on the recoveries of Mn(II), Co(II), Ni(II), and Cu(II). Figure displays that the acquired percentage for the investigated ions were increased steadily as the chelator mass increased from 0.1 up to 0.2 g. However, the chelator mass between 0.5 and 1.0 g approximately give similar recoveries percentage and any further addition of resin did not show any significant changes. The enhancement in the recovery values with the increment of chelator mass is predictable due to the improved surface area of the chelating resin and the availability of additional adsorption (chelating) centers [Citation59,Citation60]. Evidently, 0.5 g of 8-HQ-Amberlite IRC-50 chelator is practically sufficient to recover maximum percentage of the investigated metal ions [Citation61].
3.5. Effect of contact time
The contact time of analytes with sorbent is essential parameter in SPE process. In this work, the influence of this factor on metal extraction by 8-HQ-Amberlite-IRC-50 chelator was investigated over the time span extended from 2 to 100 min. at room temperature. Commonly, the sorption of metal ions is enhanced as the contact (shaking) time increased [Citation62]. Figure shows that more than %50 of Co(II), Cu(II) metals were taken up by the resin in the first 5 min, while 10 min. is required to retain 60.1, and 64.78% of Mn(II), and Ni(II) respectively. An equilibration time of 30 min was found to be sufficient for reach 100% sorption of Co(II), whereas the maximum recovery (100%) of Mn(II), Cu(II), and Ni(II) were attained after 60 min.[Citation63]. The steadily increase in sorption rate from start to 60 min. is due to the high concentration of ions as well as to the availability of more chelating sites that could be involved in the sorption of metal ions. However, the stability of sorption value released when contact time increased beyond 60 min indicates that that the sorption equilibrium is reached [Citation64].
3.6. Analytical figures of merit
The analytical figure of merits such as precisions (RSD%), linearity, and limit of detection (LODs) were investigated at the optimum SPE experimental conditions and the results presented in Table . The calibration standards employed for the determination of metal ions were prepared in aqueous solution containing 1% nitric acid and buffered with ammonium acetate 0.2M immediately before being loaded into the SPE cartridge. Then the sequestered metal ions were eluted with 1.5 M HNO3, diluted with water and quantified with the ICP-MS.
Table 4. Figure of merits from the modified matrix with 8-HQ-Amberlite IRC-50.
As can be seen from the table, the calibration curve for Mn(II), Co(II), Ni(II), and Cu(II) metal ions were linear over the concentration range; 0.5-50 ng ml−1 with correlation coefficient; R2 values 0.99 for all ions. The calculated detection limit (LOD) values ranged from 0.024 ng ml−1 for Mn to 0.057 ng ml−1 for Cu. The values of RSD% was low for all metals at 1.08 ng ml−1–1.66 ng ml−1. This finding emphasizes the precision of the SPE method and its viability for the preparation of real samples.
3.7. Method application: the analysis of groundwater samples
The performance of the chelator as SPE material for the extraction/preconcentration of trace metals was tested using groundwater samples collected from five wells (72–134 m depth) located in Swary valley (upper catchment of Alwadi Almubarak) as depicted in the map (Figure ). At sampling time, the total dissolved solid (TDS) ranged from 354 to 894 mg/L and pH was in the range 7.4-8.1. The samples (1 L) were acidified to pH lower than 2, filtered in field and transported to laboratory in iceboxes. For the commencement of sample preparation, the samples were buffered using ammonium acetate solution (0.2M) and processed following the SPE procedure in section 2.5. The concentration of the analyzed metal ions in groundwater samples, together with the recovery values (against spiked samples) are represented in Table .
Table 5. Characteristic and trace metal analysis of groundwater real samples following SPE by 8-HQ-Amberlite IRC-50.
The recovered values calculated from spiking the real samples with 5 ng/ml of the studies ions were in the range of 88.7-105.9%, 102.26- 107.7%, 97.16 −112.5%, and 88-103.9% for Mn(II), Co(II), Ni(II), and Cu(II) respectively. This good to excellent recovery values for all the tested metal ions verifies the efficacy of the prepared chelating resin as an adequate SPE sorbent for the extraction of the investigated ions from groundwater samples.
As can be seen from results summarized in Table , the concentrations of the analyzed metal ions in ground water samples were in the range (3.595-4.667), (1.373- 1.195), (1.105-1.647) and (3.201- 7.039) ng ml−1 for Mn(II) Co(II) Ni(II) and Cu(II) in same order. Obviously, there is no significant disparity in the concertation values of the investigated metal ions in the groundwater samples and the slight variations due to the geological composition. Comparatively, the obtained concentrations for the studied metal ions were relatively lower than the values reported in groundwater samples from Almedinah AlMunawarah [Citation65].
3.8. Resin reusability
The amberlite-8-HQ chelating resin synthesized in this work demonstrated an excellent stability when used as a packed chelator in standard SPE cartridges. It has been used for more than 50 SPE cycles, using metal solutions of different concentrations and 1.5 M nitric acid as an eluent solution, without any observed diminution in its sorption capacity. The resin also suspended into acidic (2 M nitric acid) and basic (0.5 M ammonium hydroxide pH 11) solutions to evaluate its stability in harsh environment. The resin was agitated in acidic and basic media for 3 h with no significant change in capacity values for the investigated metal ions. Additionally, the resin has been stored for more than one year without any noticeable change in its performance as SPE material for the studied metal ions.
4. Conclusion
The chemical attachment of 8-HQ moieties onto the surface of weak cation exchanger polymer; Amberlite IRC-50 was fund to enhance its sorption capacity by more than 50% for Mn(II), Co(II), Ni(II), and Cu(II) from standard solutions. The chelator shows optimal extraction performance for the studied metals at pH 6 using 0.5 g and during a contact time of 60 min. It has demonstrated satisfactory performance as sample preparation material for the extraction/preconcentration of the investigated metal ions from groundwater real samples obtained from Swary valley. The average recovery values from real samples for Mn(II) and Cu(II) were in the range 88-105% while that of Co(II) and Ni(II) were ranged from 97 to112%. Therefore, the resin is perfect SPE material for trace metals from similar water matrices.
Acknowledgment
The authors appreciate the generous financial support from King Abdulaziz city for Science and Technologies (KACST) for financial support (grant No: 1-17-01-006-0004).
Disclosure statement
No potential conflict of interest was reported by the author(s).
Additional information
Funding
References
- Ullah AKMA. Preconcentration of copper, cadmium and lead on Amberlite XAD-4 resin functionalized with 2-hydroxynaphthalene-1-carbanaldehydethiosemicarbazone and their determination in green tea leaves by GF-AAS. Am J Chem Eng. 2014;2(4):35. doi:10.11648/j.ajche.20140204.11.
- Türker AR. Separation, preconcentration and speciation of metal ions by solid phase extraction. Sep Purif Rev. 2012;41(3):169–206. doi:10.1080/15422119.2011.585682.
- Oral EV, Dolak I, Temel H, et al. Preconcentration and determination of copper and cadmium ions with 1,6-bis(2-carboxy aldehyde phenoxy)butane functionalized Amberlite XAD-16 by flame atomic absorption spectrometry. J HazardMater. 2011;186(1):724–730.
- Tewari PK, Singh AK. Synthesis, characterization and applications of pyrocatechol modified amberlite XAD-2 resin for preconcentration and determination of metal ions in water samples by flame atomic absorption spectrometry (FAAS). Talanta. 2001;53(4):823–833.
- Guo Y, Din B, Liu Y, et al. Preconcentration and determination of trace elements with 2-aminoacetylthiophenol functionalized Amberlite XAD-2 by inductively coupled plasma-atomic emission spectrometry. Talanta. 2004;62(1):209–215. doi:10.1016/S0039-9140(03)00423-5.
- Felipe S, Veguería J, Marcus J, et al. Trace element determination in seawater by ICP-MS using online, of fl ine and bath procedures of preconcentration and matrix elimination. Microchem J. 2013;106:121–128. doi:10.1016/j.microc.2012.05.032.
- Ridgway K, Lalljie SPD, Smith RM. Sample preparation techniques for the determination of trace residues and contaminants in foods. J Chromatogr A. 2007;1153(1–2):36–53. doi:10.1016/j.chroma.2007.01.134.
- Bader NR. Sample preparation for flame atomic absorption spectroscopy: an over view. Rasayan J Chem. 2011;4(1):49–55. doi:10.1016/0584-8547(96)01507-8.
- Salgado AL, Veloso AMO, Pereira DD, et al. Recovery of zinc and manganese from spent alkaline batteries by liquid – liquid extraction with Cyanex 272. J Power Sour. 2003;115(2):367–373. doi:10.1016/S0378-7753(03)00025-9.
- Feist B, Mikula B. Preconcentration of some metal ions with lanthanum-8-hydroxyquinoline co-precipitation system. Food Chem. 2014;147:225–229. doi:10.1016/j.foodchem.2013.09.149.
- Heiden ES, Gore DB, Stark SC. Transportable EDXRF analysis of environmental water samples using Amberlite IRC748 ion-exchange preconcentration. Int J. 2010;39(3):176–183. doi:10.1002/xrs.1244.
- Tiwari S, Ruhil J, Saxena R. A solid phase extraction method for determination of Cadmium in industrial water samples using Flame atomic absorption spectrometry. Int J Eng Technol SciRes. 2016;3(2):64–70.
- Tokal Ş, Vedat Y, Kartal Ş. Solid phase extraction of Cu (II), Ni (II), Pb (II), Cd (II) and Mn (II) ions with 1- (2-thiazolylazo) -2-naphthol loaded Amberlite XAD-1180. Environ Monit Assess 2009;152(1–4):369–377. doi:10.1007/s10661-008-0322-6.
- Tian H, Chang X, Hu Z, et al. Activated carbon modified with 4- (8-hydroxyquinoline-azo) benzamidine for selective solid-phase extraction and preconcentration of trace lead from environmental samples. Microchim Acta. 2010;171(3):225–232. doi:10.1007/s00604-010-0434-x.
- Türker AR. New sorbents for solid-phase extraction for metal enrichment. Clean Soil Air Water. 2007;35(6):548–557. doi:10.1002/clen.200700130.
- Orlando RM, Rath S, Rohwedder JJR. Semiautomated solid-phase extraction manifold with a solvent-level sensor. Talanta. 2013;116:39–44. doi:10.1016/j.talanta.2013.04.077.
- Camel V. Solid phase extraction of trace elements. Spectrochim Acta A. 2003;58:1531–1540. doi:10.1016/S0584-8547.
- Liu C, He S, Shen K, et al. (2015). l-Cysteine functionalized silica gel as an efficient adsorbent for the determination of heavy metals in foods by ICP-MS.
- Nema T, Chan ECY, Ho PC. Application of silica-based monolith as solid phase extraction cartridge for extracting polar compounds from urine. Talanta. 2010;82(2):488–494. doi:10.1016/j.talanta.2010.04.063.
- AlSuhaimi AO. Preparation of silica-4-(2-pyridylazo) Resorcinol chelator for solid phase extraction of transition metals from groundwater. J Chem Soc Pak. 2019;41(1):151–160.
- Albishri HM, Marwani HM. Chemically modified activated carbon with tris(hydroxymethyl)aminomethane for selective adsorption and determination of gold in water samples. Arabian J Chem. 2016;9:S252–S258. doi:10.1016/j.arabjc.2011.03.017.
- AlQadhi NF, AlSuhaimi AO. Chemically functionalized activated carbon with 8-hydroxyquinoline using aryldiazonium salts/diazotization route: Green chemistry synthesis for oxins-carbon chelators. Arabian J Chem. 2020;13(1):1386–1396. doi:10.1016/j.arabjc.2017.11.010.
- Leinonen H, Lehto J. Ion-exchange of nickel by iminodiacetic acid chelating resin Chelex 100. React Funct Polym. 2000;43(1):1–6. doi:10.1016/S1381-5148(98)00082-0.
- Cesur H, Bati B. Determination of copper by FAAS after preconcentration with lead-4-methylpiperidinedithiocarbamate on microcrystalline naphthalene by solid-phase extraction. Anal Lett. 2000;33(3):489–501. doi:10.1080/00032710008543068.
- Al-Degs YS, El-Sheikh AH, Al-Ghouti MA, et al. Solid-phase extraction and simultaneous determination of trace amounts of sulphonated and azo sulphonated dyes using microemulsion-modified-zeolite and multivariate calibration. Talanta. 2008;75(4):904–915. doi:10.1016/j.talanta.2007.12.032.
- Liu X, Yu Y, Zhao M, et al. Solid phase extraction using magnetic core mesoporous shell microspheres with C18-modified interior pore-walls for residue analysis of cephalosporins in milk by LC-MS/MS. Food Chem. 2014;150:206–212. doi:10.1016/j.foodchem.2013.10.145.
- De Oliveira FM, Somera BF, Corazza MZ, et al. Cellulose microfiber functionalized with N,N′-bis (2-aminoethyl)-1,2-ethanediamine as a solid sorbent for the fast preconcentration of Cd(II) in flow system analysis. Talanta. 2011;85(5):2417–2424. doi:10.1016/j.talanta.2011.07.088.
- Ezoddin M, Shemirani F, Abdi K, et al. Application of modified nano-alumina as a solid phase extraction sorbent for the preconcentration of Cd and Pb in water and herbal samples prior to flame atomic absorption spectrometry determination. J Hazard Mater. 2010;178(1–3):900–905. doi:10.1016/j.jhazmat.2010.02.023.
- Tavallali H, Malekzadeh H, Dadvar F, et al. Chemically functionalized γ-alumina with Alizarin red-s for separation and determination of trace amounts of Pb(II) and Ag(I) ions by solid phase extraction–flame atomic absorption spectrometry in environmental and biological samples. Arabian J Chem. 2017;10:S2090–S2097. doi:10.1016/j.arabjc.2013.07.040.
- Ahmad A, Siddique J.A. L, Kumar R MA, et al. New generation Amberlite XAD resin for the removal of metal ions: A review. J Envir Sci. 2015;31(1):104–123. doi:10.1017/CBO9781107415324.004.
- Garg BS, Sharma RK, Bhojak N, et al. Chelating resins and their applications in the analysis of trace metal ions. Microchem J. 1999;61(2):94–114. doi:10.1006/mchj.1998.1681.
- Kantipuly C, Katragadda S, Chow A, et al. Chelating polymers and related supports for separation and preconcentration of trace metals. Talanta. 1990;37(5):491–517. doi:10.1016/0039-9140(90)80075-Q.
- Masqué N, Marcé RM, Borrull F. New polymeric and other types of sorbents for solid-phase extraction of polar organic micropollutants from environmental water. TrAC, Trends Anal Chem. 1998;17(6):384–394. doi:10.1016/S0165-9936(98)00019-3.
- Sharma RK, Pant P. Preconcentration and determination of trace metal ions from aqueous samples by newly developed gallic acid modified Amberlite XAD-16 chelating resin. J Hazard Mater. 2009;163(1):295–301. doi:10.1016/j.jhazmat.2008.06.120.
- Leon-Gonzalez ME, Pérez-Arribas LV. Chemically modified polymeric sorbents for sample preconcentration. J Chromatogr. 2000;902:3–16.
- Sahni SK, Reedijk J. Coordination chemistry of chelating resins and ion exchangers. Coord Chem Rev. 1984;59(C):1–139. doi:10.1016/0010-8545(84)85053-5.
- Hodge P, Houghton MP, Chakiri A. A new route to functional polymers: synthesis of functional polymer-supported esters from a weak acid ion exchange resin. Reac Funct Polym. 2012;72(12):967–972. doi:10.1016/j.reactfunctpolym.2011.11.007.
- Roy K, Basu S. Separation of gold and silver using a chelating resin - Thiosemicarbazide incorporated Amberlite IRC-50. Indian J Chem Sect A. 2005;44(3):531–534.
- Roy K, Basu S, Ramaswami A, et al. Incorporation of thiosemicarbazide in Amberlite IRC-50 for separation of astatine from α-irradiated bismuth oxide. Appl Radiat Isot. 2004;60(6):793–799. doi:10.1016/j.apradiso.2003.12.011.
- Fan J, Wu C, Wei Y, et al. Preparation of xylenol orange functionalized silica gel as a selective solid phase extractor and its application for preconcentration-separation of mercury from waters. J Hazard Mater. 2007;145(1–2):323–330. doi:10.1016/j.jhazmat.2006.11.025.
- Scheuerman RA, Tumelty D. The reduction of aromatic nitro groups on solid supports using sodium hydrosulfite (Na2S2O4). Tetrahedron Lett. 2000;41(34):6531–6535. doi:10.1016/S0040-4039(00)00959-X.
- Islam A, Zaidi N, Ahmad H, et al. Synthesis, characterization, and systematic studies of a novel aluminum selective chelating resin. Environ Monit Assess. 2014;186(9):5843–5853. doi:10.1007/s10661-014-3823-5.
- AlSuhaimi AO, McCready T. Rapid immobilisation of 8-hydroxyquinoline onto silica materials and its application for on- line solid-phase extraction of transition metas from environmental samples before ICP-OES determination. Global Nest J. 2012;14(1):55–65.
- Saxena R, Meena PL. Flow injection online solid phase extraction system using Amberlite XAD-16 functionalized with 8-hydroxyquinoline for copper and zinc determination by flame atomic absorption spectrometry. RSC Adv. 2014;4(39):20216–20225. doi:10.1039/C4RA01260G.
- Qiu X, Hu H, Yang J, et al. Hydrometallurgy removal of trace copper from simulated nickel electrolytes using a new chelating resin. Hydrometall. 2018;180:121–131. doi:10.1016/j.hydromet.2018.07.015.
- Liu B, Zhao H, Tan Y, et al. Novel crosslinkable epoxy resins containing phenylacetylene and azobenzene groups : from thermal crosslinking to fl ame retardance. Polym Degrad Stab. 2015;122:66–76. doi:10.1016/j.polymdegradstab.2015.10.009.
- Mazzotta E, Rella S, Turco A, et al. XPS in development of chemical sensors. RSC Adv. 2015;101:83164–83186. doi:10.1039/c5ra14139g.
- Xu Y, Li Z, Zhang F, et al. New nitrogen-rich azo-bridged porphyrin- conjugated microporous networks for high performance of gas capture and storage. RSC Adv. 2016;6(36):30048–30055. doi:10.1039/c6ra04077b.
- Popa A, Davidescu CM, Negrea P, et al. Synthesis and characterization of phosphonate ester/phosphonic acid grafted styrene - divinylbenzene copolymer microbeads and their utility in adsorption of divalent metal ions in aqueous solutions. Ind Eng Chem Res. 2008;47(6):2010–2017. doi:10.1021/ie070886g.
- Qureshi I, Memon S. Synthesis and application of calixarene-based functional material for arsenic removal from water. Appl Water Sci. 2012;2(3):177–186. doi:10.1007/s13201-012-0035-4.
- Fateme and Faghihian. Degradation of Alizarin Yellow R using UV / H 2 O 2 Advanced Oxidation process. Environ Sci Technol. 2015;33(2):482–489. doi:10.1002/ep.
- Daneshfar A, Ghaedi M, Vafafard S, et al. Amberlite IR-120 modified with 8-hydroxyquinoline as efficient adsorbent for solid-phase extraction and flame atomic absorption determination of trace amounts of some metal ions. Biol Trace Elem Res. 2012;145(2):240–247. doi:10.1007/s12011-011-9171-1.
- Gundogdu A. Determination of some trace metals in environmental samples by flame AAS following solid phase extraction with Amberlite XAD-2000 with 8-hydroxyquinoline. Chin J Chem. 2007;25(2):196–202. doi:10.1002/cjoc.200790040.
- Vassileva E, Furuta N. Application of iminodiacetate chelating resin muromac A-1 in on-line preconcentration and inductively coupled plasma optical emission spectroscopy determination of trace elements in natural waters. Spectrochim Acta Part B. 2003;58(8):1541–1552. doi:10.1016/S0584-8547.
- Çekiç SD, Filik H, Apak R. Use of an o -aminobenzoic acid-functionalized XAD-4 copolymer resin for the separation and preconcentration of heavy metal (II) ions. Anal Chim Acta. 2004;505(1):15–24.
- Karadaş C, Kara D. On-line preconcentration and determination of trace elements in waters and reference cereal materials by flow injection – FAAS using newly synthesized 8-hydroxy-2-quinoline carboxaldehyde functionalized Amberlite XAD-4. J Food Comp Anal. 2013;32(1):90–98.
- Dierssen H, Balzer W, Landing WM. Simplified synthesis of an 8-hydroxyquinoline chelating resin and a study of trace metal profiles from Jellyfish Lake, Palau. Marine Chem. 2001;73(3–4):173–192.
- Edebali S, Pehlivan E. Evaluation of chelate and cation exchange resins to remove copper ions. Powder Technol. 2016;301:520–525. doi:10.1016/j.powtec.2016.06.011.
- Srikanth MV, Sunil SA, Rao NS, et al. Ion-Exchange resins as controlled drug delivery carriers. J Sci Res 2010;2(3). doi:10.3329/jsr.v2i3.4991.
- Gurnani V, Singh AK, Venkataramani B. Cellulose functionalized with 8-hydroxyquinoline: New method of synthesis and applications as a solid phase extractant in the determination of metal ions by flame atomic absorption spectrometry. Anal Chim Acta. 2003;485(2):221–232. doi:10.1016/S0003-2670(03)00416-1.
- Khalil TE, El-Dissouky A, Rizk S. Equilibrium and Kinetic studies on Pb2+, Cd2+, Cu2+and Ni2+adsorption from aqueous solution by resin 2, 2’- (ethylenedithio)diethanol immobilized Amberlite XAD-16 (EDTDE-AXAD-16) with Chlorosulphonic acid. J Mol Liq 2016;219:533–546. doi:10.1016/j.molliq.2016.03.063.
- AlSuhaimi AO, AlRadaddi SM, Al-Sheikh Ali AK, et al. Silica-based chelating resin bearing dual 8-hydroxyquinoline moieties and its applications for solid phase extraction of trace metal from seawater prior to thier analysis by ICP-MS. Arabian J Chem. 2019;12(3):360–369. doi:10.1016/j.arabjc.2017.10.006.
- Khazaeli S, Nezamabadi N, Rabani M, et al. A new functionalized resin and its application in fl ame atomic absorption spectrophotometric determination of trace amounts of heavy metal ions after solid phase extraction in water samples. Microchem J. 2013;106:147–153. doi:10.1016/j.microc.2012.06.002.
- Zhuo W, Xu H, Huang R, et al. A chelating polymer resin: synthesis, characterization, adsorption and desorption performance for removal of Hg(II) from aqueous solution. J Iran Chem Soc. 2017;14(12):2557–2566. doi:10.1007/s13738-017-1190-1.
- Alsuhaimi AO. Preparation of silica-4-(2-pyridylazo) resorcinol chelator for solid phase extraction of transition metals from groundwater. J Chem Soc Pak. 2019;41(1):151–160.