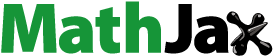
ABSTRACT
A proficient, eco-friendly, and cost-effective catalyst for remediation of organic contaminants in the environment is a burning issue in recent decades. In the present study, the cobalt doped MnO (Co-MnO) photocatalyst was synthesized via cost-effective and environmentally friendly reverse micelle route. The X-ray diffraction (XRD) results confirmed the synthesis of well-defined cubic-phased MnO and Co-MnO. XRD structural parameters and Williamson-Hall plot of MnO and Co-MnO samples were also explained in detail. Optical band gap analysis revealed an enhanced red-shift of 2.16 eV for Co-MnO photocatalyst as compared to pristine MnO (2.81 eV). The remediation efficiency of pristine MnO and its cobalt doped sample was also was tested using an organic contaminant crystal violet (CV) dye. Excellent synergistic effects of adsorption and photocatalysis at normal conditions, simple degradation mechanism, impressive kinetic investigation, and the low dose utilization proved that the newly synthesized Co-MnO photocatalyst might have commercial applications for environmental applications.
1. Introduction
The rapidly increasing trend of industrialization to meet the demands of the ever-growing world population has created many environmental concerns. Among the all commonly established industries, the textile industries pose more threat to both environmental as well as aqueous life. This is because textile industries employed numerous toxic organic dyes and oxidizing agents for colouring and bleaching purposes. Unfortunately, most of the textile sectors discharge their toxic waste into the running fresh water without any initial treatment. Hence, the toxic chemical present in the textile sector effluents can negatively affect the quality of water and can be harmful to aquatic life on digestion [Citation1–3]. The polluted environment in connection with excessive utilization of hydrocarbon-based resources buried in the heart of the earth has created a new era of discussion among researchers and scientists to cope up with the increasing demand for clean and sustainable energy in the last three decades [Citation4,Citation5].
The self-designed nanoparticle building units into the highly ordered assembly of nanostructures is one of the challenging research areas in material science and material technology. Such type of hierarchical masterpieces shows potential applications in electronics, optics, catalysis, optoelectronics, etc. [Citation6,Citation7]. Numerous transition metal oxide like TiO2, RuO2, MnO2, NiO, V2O5, Co3O4, etc. have been studied as photocatalysts for the degradation of organic wastes but nanostructured manganese and cobalt-based oxides are the superior due to their natural abundance, non-toxic nature, and variable oxidation states [Citation8–10]. In this running decade, different varieties of metal-based oxides such as Co3O4, CuO, MnO2, ZnO, NiO, CuO, TiO2, etc., have been discovered and synthesized in the laboratory. Among these metal oxides, cobalt oxides, manganese oxides or doped manganese oxides revealed many potential applications in scientific research that tries to resolve environmental and energy-related issues [Citation9,Citation11].
Photodegradation of organic pollutants, dye-sensitized solar cells, water splitting for hydrogen evolution, dye-sensitized lithium-ion batteries are examples of the solution to energy and environmental problems. Nowadays, advanced oxidation processes are being successfully utilized for the degradation of pollutants in environments [Citation12–14]. Among these treatment methods, the semiconductor photocatalyst technique emerged as a green technology has resolved the burning issue of purification of non-selective organic pollutants floating in the environment and water [Citation3,Citation15,Citation16].
Controlled crystal growth and dense packing of prepared nanocrystals can enhance the photocatalytic activity and performance of photocatalysts. The efficiency of photocatalysts is based on the fast interparticle electron transfer between nanoparticles [Citation17]. Commonly, the transition metal oxides like manganese oxide have a wider bandgap, that is why it showed photocatalytic activity only under UV light irradiation. Doping of transition metal ions such as cobalt ions into manganese oxide makes its bandgap narrow that not only enhanced its photocatalytic activity but also increase its chemical stability [Citation18].
The doping of a suitable transition metal cation in MnO crystal lattice seems to be a very attractive approach due to three reasons. Firstly, a suitable metal dopant can lower the optical energy band gap of the MnO photocatalyst by introducing a sub-energy level in the bandgap of the host material. Bashir et al. decrease the band gap of MnO2 from 2.49 eV to 2.19 eV via copper cation doping [Citation19]. Aadil et al. described that the optical band gap of Co3O4 can be reduced from 2.48 eV to 2.29 eV via Ag doping [Citation20]. Secondly, the probability of hole-electrons recombination process can be minimized via transition metal doping, this is because transitional metal cations have partially filled d-orbital and have a strong affinity to trap the photo-generated electrons [Citation21]. Thirdly, the strategy of transition metal cation doping to enhance the electrical conductivity of the host material is much better than its composite formation with the conductive matrix [Citation22]. The photocatalyst with superior electrical conductivity facilitates the charge transport from their generation site toward their reaction site.
Chen et al has synthesized nickel and cobalt doped MnO2 catalyst for the oxidation of propane. It was noticed that the Co-doped MnO2 exhibited 27.3%, whereas Ni-doped MnO2 showed 25.7% oxidation. Thus it can be concluded that Co can be used dopant to improve the properties of catalyst materials [Citation23]. Dheeraj Mondal synthesized copper doped MnO2 and employed in supercapacitive and photocatalytic applications. Copper doped MnO2 proved to be a good photocatalyst having 73% degradation efficiency in 180 min [Citation24]. Despite the fact, the morphological, structural, and optical properties of nanostructured transition metal oxides especially depend on their phase purity, crystallite size, and optical characteristics [Citation25,Citation26]. The synthesis approach mostly influences the solubility of foreign impurity ions (dopant) in the crystal lattice and the control over new secondary phases. These secondary phases also appear after post heat treatment in some cases. The reverse micelle route has proved to be the best way to control the particle size, dimensions or geometry, surface area, and particularly the implantation of foreign impurity(dopant) [Citation24,Citation27]. To synthesize single-phase material with good adsorption characteristics, the reverse-micelle route is the best technique. Therefore, to prepare the single-phase, cheap, porous, and nature-friendly MnO sample the reverse-micelle route is the best choice [Citation28,Citation29]. The search for an economical and efficient transition metal oxide-based photocatalyst is the need of the present day. To account for the advantage of the synergistic impact of photocatalysis and good adsorption characteristics, we synthesized the MnO and Co-MnO photocatalysts via a cost-effective reverse micelle route for environmental remediation applications that have not been investigated previously according to the best of our knowledge.
2. Experimental sections
2.1. List of chemicals used
The chemicals of our experimental section were utilized throughout without any processing. They were used as they were received. Manganese acetate [(Mn (CH3COO)2. 4H2O), Merck company, 98%], liquid Paraffin [Merck company], Triton X-100 [C34H62O11, Sigma-aldrich], Cobalt Chloride [CoCl2. 6H2O, Sigma-Aldrich, > 98%], Sodium hydroxide [NaOH, Daejung, 99%, purity], 1-butanol [Merck, ??98% purity], and Ethanol [Daejung, 98%].
2.2. Characterization techniques
Structural elucidation of MnO and Co-MnO samples were performed by XRD (X rays powder diffraction) using Philips X’ Pert PRO (3040–60) diffractometer. Optical analysis (Absorption spectra, bandgap determination, degradation UV-Visible spectra) was done using instrument Carry 60 UV-Visible spectrophotometer.
2.3. Synthesis scheme
The MnO and Co-doped MnO samples were synthesized via the economical reverse micelle method. Three Co-doped MnO samples were synthesized using 0.4%, 0.8%, and 1.6% cobalt ion molar percentage and expressed as Co-MnO-A, Co-MnO-B, and Co-MnO-C respectively. A low-cost surfactant or templating agent (TritonX-100) with a co-templating agent (1-butanol) was used. Common low-cost paraffin oil was employed as an oil phase. salt solutions of manganese and cobalt were prepared first according to stoichiometric ratios. Using the reverse-micelle method the mixture TritonX-100, templating agent / 1-butanol, co-templating agent / liquid Paraffin, oil-phase was added in a reaction mixture containing the salt solution. Precipitating agent, 1 Molar sodium hydroxide solution was poured drop by drop to raise the pH value of reaction media at 11. The reaction mixture was kept on continuous stirring for 2 h. Washed the precipitates with solvent ethanol and deionized water repeatedly to eliminate the excess surfactant, contaminants, by-products, and unreacted ions and to adjust the neutral pH value. Then, dried the remaining material at 105 °C in an oven. In the end, the annealing of the synthesized samples was made in a vacuum condition at 400 °C for 4 h.
2.4. Photocatalysis study
In this typical process of photocatalyst based experimental investigation, a power lamp of 60-Watt was used as a visible source of light. CV an organic dye was employed as a model toxic chemical contaminant for degradation experimentation. In this typical method, 20 mg of catalyst was transferred in a 50 cm3 solution of crystal violet dye solution of 7 ppm concentration. At first, the entire system was placed in a dark condition to ensure adsorption–desorption equilibrium for 45 min. Then, the reaction container or system was put under the 60-Watt power lamp i.e. under visible light exposure with continuous stirring. After 15 min of time duration, the 3 cm3 sample from the reaction mixture was taken with the help of a pipette and centrifuged to obtain the supernatant liquid without solid particles with a regular break of time. Repeat the preceding process for an hour. Absorbance/amount of CV in the supernatant liquid was determined using a UV–Visible spectrophotometer. Finally, calculate the percentage degradation value by using formula (1) and (2).
(1)
(1) C0 = Concentration of CV at zero time; C1 = Concentration of CV at any time
(2)
(2) Here, the value of concentration (C) is analogous to the value of absorbance (A).
3. Results and discussion
3.1. XRD study
Structural elucidation of synthesized MnO and Co-MnO were carried out using X-rays diffraction technique and obtained diffraction profiles are shown in Figure .
It is clear that the diffraction peaks at 2θ values, 35.61°, 41.35°, 60.09°, 71.66°, and 75.70° corresponded to 111, 200, 220, 311, and 222 diffraction plans of MnO and well-matched with ICCD data card no. 01-075-0257. Moreover, two additional diffraction peaks at 44.12° and 64.5° were also detected in the XRD profiles of all three Co-doped MnO samples. In fact, these two newly detected diffraction may obese due to the diffractions from the 400 and 440 crystal planes of Co-dopant (ICCD # 43–1003) [Citation30]. The intensity of dopant ion peaks is highest for the Co-MnO-C sample due to the higher percentage molar ratio of dopant metal cation was maximum (1.6%) during the fabrication of this sample. The Co dopant preferred to crystallize along 400 and 440 diffraction plans hence the characteristic intensity of diffraction from these plans was gone to increase with the increasing Co contents. It was also found that the Co-MnO-B and Co-Mn-C samples have prominent additional peaks (400, 440), indicating the formation of a new Co3O4 phase in these samples.
The unit cell parameters including cell length and volume for each all four samples are tabulated in Table [Citation31]. All these values were calculated via cell software and Highscore software.
Table 1. XRD structural parameters’ calculations of MnO and its three Co-doped samples.
The average crystallite sizes of MnO and its three Co-doped samples were calculated by using the following Sherrer and Williamson-Hall plot relationships [Citation32,Citation33].
(3)
(3)
(4)
(4) It was noted that the crystallite size of the MnO phase calculated by two different approaches was identical in comparison. However, the Sherrer method was giving slightly greater than the Williamson-Hall plot method, which was due to the microstrain and variation in geometry of the material [Citation34]. The microstrain calculated from the W-H plot method was due to compression of the crystal lattice of MnO and increases as a result of increasing Co content (Figure ). Moreover, the irregularity induced in the crystal lattice of MnO in pure and doped samples was studied using dislocation density. It was found that with the increase in Co content, MnO crystal lattice becomes more and more regular and thus generated more crystallinity.
3.2. Optical properties analysis
3.2.1. Band gap investigation
To investigate the optical properties in detail, the UV–Visible absorption spectroscopy is a versatile approach. The optical absorption spectra of the MnO and Co-MnO samples were obtained to analyze their optical characteristics (Figure ).
In general, the absorbance depends on numerous parameters, such as the optical band gap, defects due to oxygen deficiency, impurity centres, and surface roughness [Citation35]. The bandgap of MnO and Co-MnO can be investigated by the absorption spectra. To compute the optical band gap, a graph of (αhν)1/n versus energy (hν) was plotted for different “n” values (value of n may be 1/2, 3/2, 2) using the Tauc equation [Citation36]. The Tauc plots for the MnO and its three cobalt doped samples are displayed in Figure . The best fit value was estimated for 1/n = 2 which is regarded as a direct allowed transition of material. After interpreting the intercept of the line at point α = 0, the value of the bandgap or optical absorption edge was calculated. A higher redshift value (2.16 eV) for the Co-doped MnO sample was observed as compared to pristine MnO (2.81 eV) for the optimal content of Cobalt in MnO. This reduction of optical band gap energy value may be attributable to the doping induced energy band edge bending [Citation37]. It was also noticed that by increasing the cobalt content in MnO, the value of the bandgap was increased (2.20 and 2.48 eV) which is justified as the appearance of the secondary phase in the material. As, Cobalt is not completely dissolved into manganese oxide matrix, so there is a strong possibility that some of the Cobalt atoms may be decorated or located at the surface of manganese oxide in the form of oxides, which could respond to create some new allowed energy states very close to the conduction band [Citation40]. Similar findings were also observed in Aluminum-doped Zinc Oxide, Tin-doped Zinc Oxide, and Indium-doped Zinc Oxide where the secondary oxides arose/decorated over ZnO as aluminum oxide, tin oxide, and indium oxide respectively [Citation37–39].
3.2.2. Photocatalytic activity
The degradation efficiency of pristine MnO and Co-doped-MnO (Co-MnO) was examined using CV organic dye as a source contaminant material in visible light irradiation. Removal profile of CV organic dye using MnO and its three cobalt doped samples as a photocatalyst are displayed in Figure . It can be seen that Co-MnO-B and Co-MnO-C photocatalyst showed excellent adsorption properties while pristine MnO and Co-MnO-A photocatalyst showed superior photodegradation properties.
Figure 5. Removal profile of CV organic dye using the XRD pattern of MnO and its three cobalt doped samples.
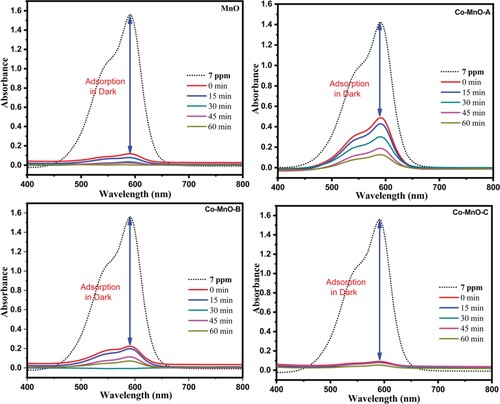
It was found that pristine MnO exhibited 99.93% overall removal with 7% photocatalytic degradation value while in comparison the photocatalytic degradation efficiency of the Co-MnO-A was increased up to 26% (Figure ). The CV organic dye removal efficiency of Co-MnO-B and Co-MnO-C photocatalyst were good but the photocatalytic degradation efficiency was smaller as compared to Co-MnO-A which is justified by the optimal content of Cobalt in the MnO matrix.it was also observed that all the samples removed the dye even in dark amazingly due to excellent adsorption characteristics while the remaining contents of dye were degraded photo catalytically.
Figure 6. The Percentage removal of CV via degradation and adsorption using MnO and its three cobalt doped samples.
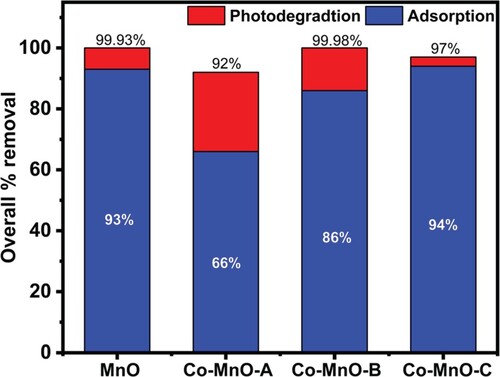
In comparison, Co-MnO-B expressed an excellent aptitude to remove the dye via both adsorption (dark scenario) and photodegradation. The synthesized materials have excellent adsorption characteristics which is the key feature of this new reverse micelle synthesis procedure. Excellent photocatalytic activity is justified by the fact that as Cobalt may not be completely dissolved into MnO lattice, so some Cobalt content may be decorated at the surface of MnO in the oxide form which could be responsible for the generation of some new allowed energy states very close to the conduction band.
3.2.4. Kinetics investigation
The kinetic investigation of the overall reaction presented that bare manganese oxide headed with rate constant 0.0071 min−1, whereas it’s Co-MnO-A sample preceded with 0.10 min−1. The calculated rate constants of Co-MnO-B and Co-MnO-C samples were 0.023 and 0.008 min−1, respectively. It is obvious from the rate constant values that pristine manganese oxide showed degradation efficiency at a slower rate as compared to the sample Co-MnO-C. The error bars demonstrating the standard deviation of the triplicate measurements for percent degradation and rate constant are displayed in Figure .
3.2.5. Reusability tests
The recycling test is a crucial feature of the new fabricated catalyst. The stability of the catalyst is an important feature that determines the potential use of photocatalyst in real applications. The reusability of the catalyst was tested for five cycles (Figure ).
Figure 8. Removal efficiency (%) of the Co-MnO-A photocatalyst as a function of the number of cycles.
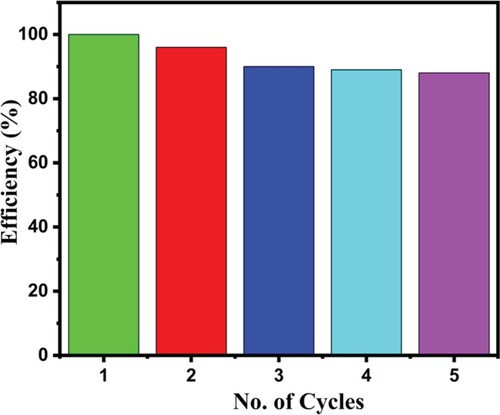
The catalyst (Co-MnO-A) was recovered by simple centrifuging and collecting at the end of the photocatalytic experiment. The recovered material was washed three times with de-ionized water and ethanol (2:1). Five cycles were performed and an overall decrease in all fie cycles was observed by almost 10%. The decrease in efficiency may be due to the mass loss of the catalyst or catalyst deactivation during the recovery procedure. The experiment validates that the fabricated catalyst is reusable and chemically stable.
The error bars demonstrating the standard deviation of the triplicate measurements for percent degradation and rate constant are displayed in Figure .
3.2.6. Mechanism of photocatalysis
In these processes, photo-induced holes and extremely reactive radicals such as OH• radicals can easily oxidize or degrade a wide range of organic pollutants. Literature reports revealed a possible mechanism scheme for the photodegradation process. Considering this mechanism, the photodegradation process of organic pollutants by Co-MnO can be proposed as follows. Enhancement in the photocatalytic activity of Co-MnO was justified by the fact that the content of Cobalt over the MnO surface may stimulate the transfer of photogenerated electrons to the exterior systems. The transport of electrons towards the decorated metal prompts those to change into negatively charged [Citation40]. Hence, in actual the Cobalt decorated on the manganese oxide surface enhanced the photocatalytic activity by boosting up the transmission of electrons to dissolve O2 molecules. Consequently, superoxide anion radical O2.- was produced as a result of O2 molecule dissolution by the transmission of trapped electrons from cobalt to O2. As a result, the recombination of the photonic generation of charge carriers was successfully suppressed and photo-oxidation efficiency was enhanced (scheme 1). Another possible justification for enhanced photocatalytic degradation mechanism is that the Fermi levels present in the orbitals of cobalt and manganese oxide join to form a modified new equilibrium Fermi level. In the interim of the irradiation process, electrons are transferred easily from newly generated fermi levels to the conduction band of manganese oxide through the surface plasmon phenomenon which enhances the photoelectronic mob and as a result the photocatalysis increases (scheme 2) [Citation10]. Actually, when Co-MnO particles were exposed to light, electrons jump from the valence band after absorbing some energy and traverses to the conduction band after being excited. Empty spaces left by electrons in valence bands turn into holes. The generation of electrons and holes is a simultaneous process. The induced holes directly interact with the surface of organic pollutants to produce very reactive radicals OH• and this OH• radical oxidizes the pollutants. The photo-generated electrons in the conduction band of MnO2 were transferred to the conduction band of Co and thus reduces the electrons holes pair recombination. The photo-generated electrons, in the meanwhile, interact with the absorbed O2 to produce O2.-. The generated O2.- then further interact with the positive hydrogen ion (H+) to yield HO.2 that can further combine with the shared electrons to produce reactive species OH•. Thus, when Co was placed in contact with MnO2, a heterojunction was developed. This heterojunction was responsible for the superior photocatalytic degradation efficiency of MnO. All these reactive radicals such as OH•, HO.2, O2.-, and holes can easily oxidize or degrade the organic pollutants to some extent. The above analysis of the photocatalytic activity reaction can be written as follows.
(3)
(3)
(4)
(4)
(5)
(5)
(6)
(6)
(7)
(7)
(8)
(8)
Our study revealed an authentic approach for the manufacturing of Co-MnO samples which show themselves as good photocatalysts. These manganese oxide-based photocatalysts possess a wide potential window in environmental remediation, solar cells, gas sensor, and optoelectronics.
To understand the photocatalytic performance of the as-prepared Co-MnO-A photocatalyst, the detailed comparison of the current work with the other reported work is given in Table .
Table 2. Comparison of photocatalytic aptitude of Co-MnO with reported photocatalysts.
4. Conclusion
In summary, MnO and Co-doped MnO (Co-MnO) photocatalyst were successfully synthesized and utilized for the removal of environmental contaminants. XRD investigation confirmed the well-defined single-phase cubic structure of MnO and the appearance of the Co3O4 phase in the Co-MnO sample at a higher concentration of Cobalt. XRD structural parameters (lattice parameters, crystallite size, strain, dislocation density) and Williamson-Hall plot of the MnO phase in Co-MnO sample were also investigated in detail. The optical band gap results showed that the bandgap of the pristine MnO sample reduced from 2.81 eV to 2.16 eV for the Co-MnO-A sample that pointed out its effective utilization in photocatalysis research. Percentage removal efficiency in dark conditions suggested its good adsorption behaviour and this reward goes to the adaptation of effective synthesis reverse micelle route. Excellent remediation efficiency of bare MnO and Co-doped MnO is due to its effective adsorption-photocatalysis synergy at normal conditions, simple degradation mechanism, fast kinetics, the low dose utilization of photocatalyst (0.2 g L−1) within 60 min contact time, and utilization of low power source (60 watt) motivated that the synthesized material is the best for environmental remediation applications and can be proficiently utilized in other applications like catalysis, supercapacitor electrode material, bio-sensors, etc.
Acknowledgements
Authors are thankful to the Islamia University of Bahawalpur (Pakistan) and Higher Education Commission of Pakistan for financial support of this project through research grant number 6276/Punjab/NRPU/ R&D/HEC/2016. Authors from King Saud University would like to extend their sincere appreciation to the King Saud University for their contribution in this research through researchers supporting project (RSP-2020/163).
Disclosure statement
No potential conflict of interest was reported by the author(s).
Correction Statement
This article has been republished with minor changes. These changes do not impact the academic content of the article.
References
- Simeon M, Adoghe AU, Wara ST, et al. Renewable energy integration enhancement using energy storage technologies. 2018 IEEE PES/IAS PowerAfrica. IEEE; 2018. p. 864–868.
- Azam Q, Mazlan NM, Arshad MO, et al. A study on aviation fuels measuring spray quality. Materials Today: Proceedings. 2020;21:1685–1688.
- Santhosh C, Malathi A, Daneshvar E, et al. Photocatalytic degradation of toxic aquatic pollutants by novel magnetic 3D-TiO 2@ HPGA nanocomposite Scientific reports 2018;8:1–15.
- Sillanpää M, Ncibi MC, Sillanpää ME. Sustainable bioeconomy. London: Springer; 2017.
- Bilgen S, Sarıkaya İ. Energy conservation policy and environment for a clean and sustainable energy future. Energ Sourc B Energ Econ Plann. 2018;13:183–189.
- Torres LEF, Roche S, Charlier J-C. Introduction to graphene-based nanomaterials: from electronic structure to quantum transport. London: Cambridge University Press; 2020.
- Yang X, Lin X, Zhao YS, et al. Recent advances in Micro-/nanostructured metal–organic frameworks towards photonic and electronic applications. Chem Eur J. 2018;24:6484–6493.
- Pawar SA, Patil DS, Shin JCJCAP. Transition of hexagonal to square sheets of Co3O4 in a triple heterostructure of Co3O4/MnO2/GO for high performance supercapacitor electrode. Curr Appl Phys. 2019;19:794–803.
- Khan I, Sadiq M, Khan I, et al. Manganese dioxide nanoparticles/activated carbon composite as efficient UV and visible-light photocatalyst. Environ Sci Pollut Res. 2019;26:5140–5154.
- Karimi-Maleh H, Kumar BG, Rajendran S, et al. Tuning of metal oxides photocatalytic performance using Ag nanoparticles integration. J Mol Liq. 2020;314:113588.
- Zhang H, Lin C, Han T, et al. Engineering, visualization of the formation and 3D porous structure of Ag doped MnO2 aerogel monoliths with high photocatalytic activity. ACS Sustain Chem Eng. 2016;4:6277–6287.
- Varjani S, Joshi R, Srivastava VK, et al. Treatment of wastewater from petroleum industry: current practices and perspectives. Environ Sci Poll Res. 2020;27:27172–27180.
- Liu J, Wu P, Yang S, et al. A photo-switch for peroxydisulfate non-radical/radical activation over layered CuFe oxide: Rational degradation pathway choice for pollutants. Appl Catal B Environ. 2020;261:118232.
- Ravelli D, Dondi D, Fagnoni M, et al. Photocatalysis. A multi-faceted concept for green chemistry. Chem Soc Rev. 2009;38:1999–2011.
- Baig MM, Zulfiqar S, Yousuf MA, et al. Structural and photocatalytic properties of new rare earth La3+ substituted MnFe2O4 ferrite nanoparticles. Ceram Int. 2020;46(14):23208–23217.
- Koe WS, Lee JW, Chong WC, et al. An overview of photocatalytic degradation: photocatalysts, mechanisms, and development of photocatalytic membrane. Environ Sci Pollut Res. 2020;27:2522–2565.
- Neppolian B, Wang Q, Yamashita H, et al. Synthesis and characterization of ZrO2–TiO2 binary oxide semiconductor nanoparticles: application and interparticle electron transfer process. Appl Catal A: Gen. 2007;333:264–271.
- Barick K, Singh S, Aslam M, et al. Porosity and photocatalytic studies of transition metal doped ZnO nanoclusters. Micropor Mesopor Mat. 2010;134:195–202.
- Warsi MF, Bashir B, Zulfiqar S, et al. Mn1-xCuxO2/ reduced graphene oxide nanocomposites: synthesis, characterization, and evaluation of light mediated catalytic studies. Ceram Int. 2020;46(7):9913–9923.
- Aadil M, Zulfiqar S, Shahid M, et al. Binder free mesoporous Ag-doped Co3O4 nanosheets with outstanding cyclic stability and rate capability for advanced supercapacitor applications. J Alloys Compd. 2020;844:156062.
- Bashir B, Khalid MU, Aadil M, et al. CuxNi1-xO nanostructures and their nanocomposites with reduced graphene oxide: synthesis, characterization, and photocatalytic applications. Ceram Int. 2020.
- Ali A, Aadil M, Rasheed A, et al. Honeycomb like architectures of the Mo doped ZnS@Ni for high-performance asymmetric supercapacitors applications. Synth Met. 2020;265:116408.
- Chen L, Song X. Facile fabrication of macroscopic self-standing Ni or Co-doped MnO2 architectures as catalysts for propane oxidation. Technologies. 2019;7:81.
- Mondal D, Das S, Paul BK, et al. Size engineered Cu-doped α-MnO2 nanoparticles for exaggerated photocatalytic activity and energy storage application. Mater Res Bull. 2019;115:159–169.
- Li W, Cui X, Zeng R, et al. Performance modulation of α-MnO2 nanowires by crystal facet engineering. Sci Rep. 2015;5:8987.
- Truong TT, Liu Y, Ren Y, et al. Morphological and crystalline evolution of nanostructured MnO2 and its application in lithium–air batteries. ACS Nano. 2012;6:8067–8077.
- Bumajdad A, Al-Ghareeb S, Madkour M, et al. Mechanisms, catalysis, synthesis of MgO nanocatalyst in water-in-oil microemulsion for CO oxidation. React Kinet Mech Cat. 2017;122:1213–1229.
- Fu X, Qutubuddin SJC, Physicochemical SA, et al. Synthesis of titania-coated silica nanoparticles using a nonionic water-in-oil microemulsion. Colloids Surf A Physicochem Eng Asp. 2001;179:65–70.
- Baig MM, Yousuf MA, Agboola PO, et al. Optimization of different wet chemical routes and phase evolution studies of MnFe2O4 nanoparticles. Ceram Int. 2019;45:12682–12690.
- Aadil M, Zulfiqar S, Sabeeh H, et al. Enhanced electrochemical energy storage properties of carbon coated Co3O4 nanoparticles-reduced graphene oxide ternary nano-hybrids. Ceram Int. 2020;46:17836–17845.
- Yousuf MA, Baig MM, Al-Khalli NF, et al. The impact of yttrium cations (Y3+) on structural, spectral and dielectric properties of spinel manganese ferrite nanoparticles. Ceram Int. 2019;45:10936–10942.
- Aadil M, Shaheen W, Warsi MF, et al. Superior electrochemical activity of α-Fe2O3/rGO nanocomposite for advance energy storage devices. J Alloys Compd. 2016;689:648–654.
- Yousaf S, Aadil M, Zulfiqar S, et al. Hierarchically porous CuO microspheres and their r-GO based nanohybrids for electrochemical supercapacitors applications. J Mater Res Technol. 2020;9(6):14158–14167.
- Yousaf S, Zulfiqar S, Shahi MN, et al. Tuning the structural, optical and electrical properties of NiO nanoparticles prepared by wet chemical route. Ceram Int. 2020;46:3750–3758.
- Arshad M, Azam A, Ahmed AS, et al. Compounds, effect of Co substitution on the structural and optical properties of ZnO nanoparticles synthesized by sol–gel route. J Alloys Compd. 2011;509:8378–8381.
- Tauc J. Amorphous and liquid semiconductors. London: Springer Science & Business Media; 2012.
- Ahmad M, Ahmed E, Zhang Y, et al. Preparation of highly efficient Al-doped ZnO photocatalyst by combustion synthesis. Curr Appl Phys. 2013;13:697–704.
- Prajapati C, Sahay PJCR. Technology, growth, structure and optical characterization of Al-doped ZnO nanoparticle thin films. Cryst Res Technol. 2011;46:1086–1092.
- Wu C, Shen L, Yu H, et al. Synthesis of Sn-doped ZnO nanorods and their photocatalytic properties. Mat Res Bull. 2011;46:1107–1112.
- Behnajady MA, Modirshahla N, Shokri M, et al. Enhancement photocatalytic activity of ZnO nanoparticles by silver doping with optimization of photodeposition method parameters. J Environ Sci Heal A. 2009;44:666–672.
- Zhang Z, Liu H, Lin Y, et al. Influence of La doping on magnetic and optical properties of bismuth ferrite nanofibers. J Nanomater. 2012;2012:5.
- Zhang N, Chen D, Niu F, et al. Enhanced visible light photocatalytic activity of Gd-doped BiFeO 3 nanoparticles and mechanism insight. Sci Rep. 2016;6:1–11.
- Kaur M, Yadav K, Uniyal P. Investigations on multiferroic, optical and photocatalytic properties of lanthanum doped bismuth ferrite nanoparticles. Adv Mater Lett. 2015;6:895–901.
- Irfan S, Rizwan S, Shen Y, et al. Mesoporous template-free gyroid-like nanostructures based on La and Mn co-doped bismuth ferrites with improved photocatalytic activity. RSC Adv. 2016;6:114183–114189.
- Sharma R, Kumar V, Bansal S, et al. Boosting the catalytic performance of pristine CoFe2O4 with yttrium (Y3+) inclusion in the spinel structure. Mater Res Bull. 2017;90:94–103.
- Gabal M, Al-Harthy E, Al Angari Y, et al. MWCNTs decorated with Mn0. 8Zn0. 2Fe2O4 nanoparticles for removal of crystal-violet dye from aqueous solutions. Chem Eng J. 2014;255:156–164.
- Rahimi-Nasrabadi M, Behpour M, Sobhani-Nasab A, et al. Nanocrystalline Ce-doped copper ferrite: synthesis, characterization, and its photocatalyst application. J Mater Sci: Mater Electron. 2016;27:11691–11697.
- Baig MM, Zulfiqar S, Yousuf MA, et al. DyxMnFe2-xO4 nanoparticles decorated over mesoporous silica for environmental remediation applications. J Hazard Mater. 2020;402:123526.