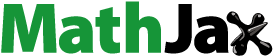
Abstract
Graphene oxide templated cobalt (GO–Co) and graphene oxide templated silver (GO–Ag) nanocomposites were synthesized and used as catalysts in the reduction of 4-Nitrophenol (4-NP), 2-Nitrophenol (2-NP) and 2-Nitroaniline (2-NA). Almost evenly distributed nanoparticles of Ag and Co were obtained on GO sheets and the diameters of most of the particles were in the range of 5–15 nm. The maximum apparent reduction rate (kapp) for 4-NP was found to be 0.58 min−1 and 0.67min−1 with corresponding activation energies of 28.79 and 16.54 kJ mol−1 in the presence of GO–Ag and GO–Co catalysts, respectively. The kapp for GO–Co cataly-zed reduction of 2-NP and 2-NA were found as 0.10 and 0.06 min−1, respectively. Catalytic activity of GO–Ag was decreased by 25% in the fifth cycle while that of GO–Co was decreased to 47% in the fourth cycle for the reduction of 4-NP. Overall, GO–Ag showed superior catalytic behaviour as compared to GO–Co.
1. Introduction
Water, being a universal solvent, is used in almost every industry from washing to synthetic steps of various products. Due to this extensive use, water is contaminated with many unwanted substances. When this contaminated water is added into industrial waste it enhances the toxicity of the industrial effluents. So, it is utmost important to remove the toxic contaminants from water before its disposal to avoid its hazardous effects on the environment, specifically on the ecosystem. The industrial effluents contain some nitrophenols such as 2-NP and 4-NP which have been listed as top organic/aromatic pollutants by United States Environmental Protection Agency (USEPA). Due to their carcinogenic, mutagenic and toxic effects, their removal from aqueous environment becomes necessary and urgent. The toxicity of these nitrophenols can be minimized by converting them into their reduced forms, aminophenols, which are also the most extensively used chemicals in hair dyeing, photographic developer, anticorrosion lubricant as well as in pesticides and surfactant industries. Aminophenols are also considered as important intermediates in many industrial syntheses like analgesic and some antipyretic drugs [Citation1]. Also, nitrophenols have been proven to be very useful starting materials for aminophenols and other related chemicals [Citation2,Citation3]. Various routes for nitrophenols reduction have been studied and reported by industrial-based researchers including electrolytic reduction, metal/acid reduction and borohydride reduction methods [Citation4–6]. Among these methodologies, borohydride reduction was found to be a promising and efficient method due to high % conversion efficiency [Citation5]. Also, this method does not generate any acid effluents and can be carried out under mild aqueous conditions [Citation6]. However, to proceed at a considerable rate, the borohydride reduction of nitrophenols needs the support of a catalyst [Citation7]. To this end, various catalysts have been reported and catalytic reduction has been considered as the most attractive method for the conversion of nitrophenols into aminophenols. So, researchers are focusing on the development of some highly efficient catalyst systems for the past few years [Citation8,Citation9] to eliminate/minimize the toxicity of nitrophenols from aqueous medium and to produce aminophenols effectively with better yield.
Among various catalysts, noble metal-based catalyst systems like silver nanoparticles-based systems are being frequently used for various organic transformation reactions [Citation10]. Das et al. have recently reported silver nanoparticles decorated nanocellulose-based membrane as an excellent catalyst for the reduction of toxic nitrocompounds [Citation11]. Another silver nanoparticle-based catalyst was developed by growing silver nanoparticles on dopamine functionalized hydroxyapatite and found effective in the reduction of 4-NP [Citation12]. In addition to silver, many catalysts have been designed based on palladium nanoparticles for the reduction of nitrocompounds along with some other reactions such as coupling reactions and oxidation of alcohols [Citation13–17]. However, some non-noble metal’s nanoparticles are also being used by researchers for catalytic applications for the sake of cost-effectiveness. Copper nanoparticle-based heterogeneous catalyst has been designed by the fabrication of copper nanoparticles on GO and the prepared catalyst was found to show good activity in the reduction of nitroarenes and organic dyes [Citation18]. Recently, Co nanoparticles have acquired growing attention for their potential reduction abilities [Citation19,Citation20] and easy synthesis. It is well known that these nanoparticles have been supported on different materials by many researchers to avoid their aggregation, further oxidation and to enhance their stability and surface reactivity. The most commonly used support materials include silica, zeolites, polymers and carbon-based materials [Citation21–23]. Among all those, carbonaceous materials have increased versatile applications in catalysis. GO, a two-dimensional sp2 hybridized carbon nanomaterial, was found to have some extraordinary properties like higher surface area, abundant hydrophilic epoxides, hydroxyl and carboxylic moieties, superior mechanical strength and flexibility [Citation24]. These properties of GO provide a brand-new avenue in combining different nanoparticles with two-dimensional surface of GO to obtain desirable material for catalytic hydrogenation [Citation25–28]. Das et al. have used GO sheets for the decoration of silver nanoparticles to design a catalyst for the rapid hydrogenation of 4-NP [Citation29]. Fakhri et al. reported the gold- and copper-doped GO nanosheets embedded in poly(vinylidene fluoride) to increase the content of electroactive phases for their possible application in sensors [Citation30]. GO-based nanocomposites have also been reported as excellent adsorbents owing to their high surface energy and good regeneration of active sites. For example, reduced graphene-iron oxide has been reported as an efficient adsorbent for the removal of phenazopyridine residues from aqueous medium [Citation31]. Various techniques have been used for the grafting of magnetic iron oxide nanoparticles on GO [Citation32]. In addition, GO-based nanocomposites have been proven as an efficient catalyst for various reactions. Hassandoost et al. have reported GO sheets anchored with magnetic nanoparticles as effective photocatalyst for the fast reduction of water pollutants [Citation33]. Similarly, Nasrollahzadeh et al. designed GO/ZnO as a heterogeneous catalyst for the synthesis of various tetrazols [Citation34]. Many other materials have also been fabricated on GO using different techniques and for different applications [Citation35–37]. The graphene-based catalysts are supposed to have benefits of high catalytic prowess, straightforward work-up and high catalytic recovery. Therefore, many heterogeneous catalysts based on graphene have been developed and used in the reduction of nitrocompounds [Citation38].
Considering the above-mentioned catalytic merits of graphene/nanoparticles system, we have fabricated stable Ag/graphene oxide and Co/graphene oxide nanocomposites using facile coprecipitation method for the catalytic hydrogenation of nitrophenol. Ag and Co nanoparticles have been chosen as the metal source because they are less expensive, easy to synthesize and handle, and above all because of their easier recovery and reusability. So, the present research work provides a wider range of applications of graphene-based nanomaterials in the fields of catalysis and wastewater treatment.
2. Experimental
Graphite powder (99.9%), sulphuric acid (H2SO4), nitric acid (HNO3), potassium permanganate (KMnO4), ice, silver nitrate (NaNO3, 99.5%), cobalt chloride hexahydrate (CoCl2.6H2O), sodium borohydride (NaBH4), and the aromatic pollutants 4-nitrophenol (4-NP), 2-Nitrophenol (2-NP), 2-Nitroaniline (2-NA) were purchased from Aldrich chemicals. Double distilled water (DDW) was used throughout in the experiment for apparatus cleansing and preparation of the solution.
2.1. GO–Co nanocomposite synthesis
First, the famous modified Hummers method was employed for GO preparation [Citation39]. Then this prepared GO was used to fabricate cobalt and silver nanoparticles. For the preparation of GO–Co nanocomposite, 30 mg of GO was dispersed in DDW (50 ml) in an ultra-sonication bath for about 1 h to fully exfoliate GO sheets. In the next step, 10 ml of 10 mM cobalt chloride hexahydrate (CoCl2.6H2O) solution was added into GO suspension and stirring was continued for about 30 min to develop cobalt ions interaction with GO sheets. Then 10 ml of sodium borohydride solution (NaBH4, 2g/L) was added to the reaction mixture and was kept on magnetic stirring for further 5 h for complete reduction. Upon completion of reaction time, the reaction mixture was centrifuged to get synthesized nanomaterial and then again dispersed in methanol and DDW for re-centrifugation. This process was repeatedly performed five times to eliminate any unreacted metal ions species. Lastly, nanocomposite was oven-dried at 60°C for 12 h.
2.2. GO–Ag nanocomposite synthesis
For GO–Ag nanocomposite synthesis, 30 mg of GO sheets was dispersed in DDW water (50 ml) in an ultra-sonication bath for about 1 h. Next, add 10 ml of 10mM AgNO3 solution (0.16987 g/100 ml) gradually to GO suspension along with continued stirring for 30 min so that silver ions can interact better with carboxyl groups of GO sheets. Then, 10 ml of sodium borohydride aqueous solution (NaBH4, 2g/L) as a reducing agent was added under vigorous magnetic stirring after which reaction was kept on stirring for about 5 h for complete reduction. It was noticed that NaBH4 addition induced a visible change in the colour of the reaction system from brown to grayish black. Upon completion of reaction time, synthesized nanocomposite was centrifuged. It was then re-dispersed in methanol and DDW and centrifuged for multiple times. Lastly, nanocomposite was oven-dried at 60°C for 12 h.
2.3. Catalytic experiment
Catalytic response of synthesized GO–Co and GO–Ag nanomaterials was examined thoroughly by employing them as nanocatalysts for the reduction of 4-NP. In a conventional experimental run, 30 ml of 0.001M 4-NP solution was taken in a flask and NaBH4 (0.113 g) was added as a reducing agent in it. Next, 3.6 mg nanocomposite (GO–Co/GO–Ag) was taken as a catalyst for the reduction reaction to start. Progress of the reaction was checked after specific time by withdrawing 0.5 ml sample from the reaction mixture and sample was diluted to certain ratio (15 times dilution for 4-NP) and absorbance values were analysed through UV–Vis spectrophotometer. UV–Vis spectrophotometer monitored catalytic reduction of 4-NP in terms of decrease in the intensity of absorbance peak of 4-NP at around 400 nm absorption maxima and corresponding reduction rate constants at different parameters were calculated. The effect of catalyst amount on reduction rate was explored by conducting reduction reactions with four different catalyst amounts (1.8 g, 3.6 g, 5.4 g, 7.2 mg). Temperature’s influence on reduction rates was tested at temperatures of 30°C, 40°C, 50°C and 60°C. Thermodynamic factors, e.g. activation energy (Ea), activation enthalpy (ΔH#) and activation entropy (ΔS#) were calculated using corresponding equations by employing temperature studies. Catalytic test was also performed in aqueous media obtained from different sources including tap water, dam water and distilled water to explore catalytic efficiency of prepared nanocomposites on the industrial level. Finally, the reusability of the nano-catalyst was investigated and evaluated by using the same catalyst for four successive cycles and after every cycle, the catalyst was filtered, washed with DDW and reused for another reaction under same reaction conditions. The catalytic reduction of 2-NP and 2-NA was conducted only at 30°C and with a catalyst amount of 3.6 mg while the rest of process was the same as adopted for 4-NP.
3. Results and discussion
3.1. Morphological characterization of GO and GO-based nanocomposites
Detailed morphological examination of prepared nanocatalysts was done by performing TEM, XRD, TGA, FT-IR characterization techniques.
Transmission Electron Microscopy (TEM) images were recorded to get information about morphological aspects of prepared materials. TEM images of both nanocomposites show that Ag and Co nanoparticles are dispersed evenly throughout GO. TEM images of synthesized materials are represented in Figure which reveal nanoparticles distribution over GO substrate. Figure (a) illustrates the TEM image of pure GO in which wavy, transparent, wrinkle design of GO can be seen. The image depicted in Figure (b) is the TEM image of GO loaded with silver nanoparticles. Dark spherical spots are visible throughout the GO surface that confirms the presence of the significant amount of Ag nanoparticles over GO. Figure (c) is the TEM image of graphene templated cobalt nanoparticles. From the TEM image of GO–Co nanocomposite, it can be seen clearly that the size of Co nanoparticles is uniform with very good monodispersity. The homogenous scattering of nanoparticles together with their even size morphology over GO matrix without significant agglomeration proved that GO can be used as a strong substrate for metal nanoparticles. In addition, the estimated diameters of most of the particles irrespective of the nature of metal were observed to be in the range of 5–15 nm. From these results, it can be concluded that GO sheets act as a nucleation and stabilization center for the growth of various metal-based nanoparticles [Citation39].
Figure represents XRD patterns of GO and GO templated Ag nanocomposites. As represented in Figure (a), the XRD pattern of GO is having a sharp intense peak at 2θ = 11.2°. The greater interlayer distance in GO network is solely due to various hydroxyl, carbonyl, carboxyl and epoxy groups [Citation20]. In the diffractogram of GO, no characteristic peak at 2θ = 26° was observed, confirming that graphite oxide was effectively oxidized. The diffraction pattern of GO templated silver nanocomposite shows all the characteristic peaks at 38.1°, 44.2°, 64.3° and 77.2°, corresponding to (111), (200), (220) and (311) lattice planes of face-centred cubic (fcc) structure of silver nanoparticles [Citation40]. All these characteristic peaks of GO templated silver nanocomposites matched well with the standard ICDD code 00-001-1167 of silver and JCPDS No. 04–0783 [Citation41]. The XRD pattern of GO–Co nanocomposite did not exhibit any sharp peak. This might be to the fact that very low amount of Co was loaded in GO and its characteristic diffraction was suppressed by massive amount of GO which was acting as continuous phase in GO–Co nanocomposite.
Figure represents FTIR spectra of GO, GO–Ag and GO–Co, respectively. The broad signal at 3195 cm−1 in FTIR spectrum of GO in Figure (a) is due to C-OH (hydroxyl group) stretching vibrations. A strong signal at 1714 cm−1 corresponds to stretching vibrations of C = O of carboxylic groups. The signal at 1615 cm−1 is attributed to skeletal vibrations of aromatic C=C bond or intramolecular hydrogen bonds. The signal at 1045cm−1 corresponds to C−O stretching vibration of the epoxy group. In the case of FTIR spectrum GO–Ag nanocomposite, Figure (b), characteristic signals of GO remain with similar shapes but with diminution in intensities. However, the intensity of C=O stretching vibrations (1714 cm−1) decreased along with peaks shifting and the appearance of new bands was noticed. The disappearance of bands at 3195cm−1 and 1045cm−1 strengthens the nanocomposite formation through carboxyl functionalities, whereas bands appeared at 1556cm−1 and 1365cm−1 are ascribed to symmetrical and asymmetric COO¯ vibrations convoluted with silver nanoparticles in composite [Citation42]. Similarly, the FTIR spectrum of GO–Co nanocomposite is shown in Figure (c). Here, the diminution of absorption bands at 3195 and 1045cm−1 also confirms the formation of GO complexed cobalt nanoparticles through carboxyl moieties. The symmetrical and asymmetrical COO¯ vibrations complexed with Co nanoparticles are represented by the bands at 1556 cm−1 and 1365 cm−1. Further, the FTIR spectra indicate that the growth of nanoparticles has not affected the GO structure.
TGA was performed to have a quantitative analysis of how much prepared GO and its Ag- and Co-based nanocomposites are thermally stable. The analysis was performed in the temperature range of 40–800°C and temperature was increased at the rate of 10°C min−1. The sample was analysed in nitrogen atmosphere maintained by flow rate 100 ml min−1. Moreover, thermograms also elaborate the enhancement of thermal stability of GO upon nanoparticles loading. Results are shown in Figure . TGA results show that prepared materials are thermally stable up to temperature around 200°C. Results also confirm that weight loss in the case of metal-loaded GO nanocomposites is much less, indicating an enhanced thermal stability in composites. About 15% weight loss of GO was noticed up to 100°C that is attributed primarily to the removal of water molecules present in the sample. At around 200°C, major weight loss of GO was noticed owing to the removal of oxygen functionalities (hydroxyl, carbonyl, epoxy and carboxyl) from GO sheets along with carbon skeleton breakdown [Citation39]. However, there is a significant decrease in decomposition temperature of nanocomposites where the first mass loss was lower than that noticed in GO and major weight loss was observed at around temperature 100°C and up to 250°C. This is primarily due to the removal of oxygen-bearing functionalities in the structure [Citation43,Citation44]. There was no weight loss observed from 250°C onwards in the case of nanocomposites indicating their much higher thermal stability as compared to pure GO. The overall TGA showed that upon heating to 800°C weight of GO, GO–Co and GO–Ag was decreased to 43%, 49.8% and 64.8%, respectively. So, the TGA results demonstrate that almost 6.8 weight % of Co was present in GO–Co and 21.8 weight % of Ag was present in GO–Ag. The presence of relatively larger contents of Ag as compared to Co in the nanocomposite can be attributed to the greater affinity of GO for Ag as compared to Co.
3.2. Catalysis
The catalytic reduction of 4-NP along with 2-NP and 2-NA was employed as a model reaction to test the catalytic performance of GO–Ag and GO–Co nanocomposites [Citation45].
The reduction of 4-NP was observed in terms of diminution in absorbance intensity of 4-nitrophenol at around 400 nm using UV–Vis spectrophotometer. Initially, only prepared nanocomposite was added in the aqueous solution of 4-NP and time-dependent UV–Vis spectra were recorded and very small decrease in the absorbance of 4-NP was observed as shown in Figure (a). This small decrease in absorbance can be attributed to the adsorption of 4-NP on the added nanocomposite. At the second step, only NaBH4 was added in the aqueous solution of 4-NP and time-dependent UV–Vis spectra were recorded. Results showed that very negligible amount of nitro compound reduction was observed (Figure b) due to the large kinetic activation energy barrier between reactants and products. However, as it is well known that a suitable catalyst can provide an alternative low activation energy path for the reduction of 4-NP. Considering this, prepared materials were employed as nanocatalysts for the reduction of 4-NP and a sharp decrease in intensity of 4-NP absorption peak was observed. The reason is that, by using catalyst the activation energy gets lowered thus providing an alternate route for the reaction to take place and making the reaction feasible. The pseudo first-order kinetic equation can be employed to calculate the rate constant values and is written as follows:
where Co refers to the initial concentration of 4-NP, Ct refers to the concentration of 4-NP at time t and kapp is the apparent rate constant.
Figure 5. Time-dependent UV–Vis absorption spectra of 4-NP (a) in the presence of catalyst only, (b) in the presence of NaBH4 only, (c) in the presence of catalyst and NaBH4. Reaction conditions: 1 mM aqueous solution of 4-Nitrophenol = 30 ml, NaBH4 = 0.113 g, GO–Co nanocatalyst = 3.6 mg, 30°C, 100 rpm.
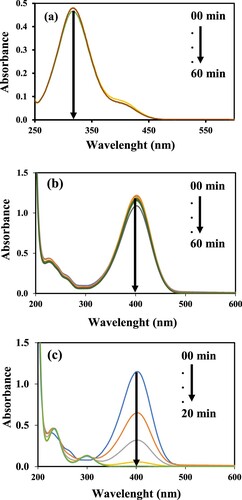
3.3. Catalytic experiment of GO–Co
The catalytic efficiency of the GO–Co nanocomposite was explored by employing a reduction reaction of 4-NP using NaBH4 as a reductant. UV–Visible absorption behaviour for the progressive 4-NP reduction in the absence of GO–Co catalyst and in its presence under mentioned operating conditions is shown in Figure (b and c). Figure (c) clearly shows that a drastic decrease in absorbance intensity was observed in the presence of nanocatalysts and almost complete nitrocompound was reduced within 20 min. After that, no change in absorbance maxima was observed which confirmed that the reduction process was finished. The GO–Co was then recycled by filtration and washing with water. This systematic experimental study reveals that the reduction of 4-NP was carried out by NaBH4 while the GO–Co nanocomposite played its role as a catalyst. The NaBH4 plays its role as a reducing agent in the reduction of 4-NP by producing borohydrides (BH4–), a strong nucleophile, which transfer electrons to catalyst and finally causes the reduction of 4-NP as reported earlier in details [Citation27]. Considering this much efficiency of GO–Co nanocatalyst, some additional parameters can also be tuned for the increase in % reduction.
3.4. Effect of catalyst amount
It is a parameter that is most fundamental and worth seeing in the reduction process because the progress depends on the surface area and the active binding sites of the catalyst. Different amounts of GO–Co nanocomposite were used, i.e. 1.8, 3.6, 5.4 and 7.2mg, and kinetics of the catalytic reactions was studied. It can be seen from Figure (a and b) that an increase in slope and hence an increase in the values of kapp from 0.28 to 0.68 min−1 (4.7 × 10−3–11.2 × 10−3 s−1) was observed as the amount of catalyst was increased from 1.8 to 7.2 mg. This increase in the apparent rate constant value confirms the availability of greater catalytic active sites and greater effective collision frequency. The maximum value of kapp which is 0.67 min−1 (11.2 × 10−3 s−1) in the current study is much larger than that reported by Cheng et al. in previous studies for 4-NP reduction reaction using GO-based nanocatalyst [Citation46]. These results indicate that the reduction process will take longer time if the amount of catalyst is taken lower than 1.8 mg. Also, with very a high amount of catalyst, the reduction process may become independent on the amount of catalyst owing to a decrease in the relative concentration of reactants [Citation47].
Figure 6. (a) Pseudo first-order plots for the reduction of 4-NP catalysed by different amounts of GO–Co nanocomposite at 30°C. (b) Linear graph of dependence of kapp on the amount of catalyst at 30°C. Reaction conditions; 1 mM aqueous solution of 4-Nitrophenol = 30 ml, NaBH4 = 0.113 g, GO–Co catalyst = 3.6 mg, 100 rpm.
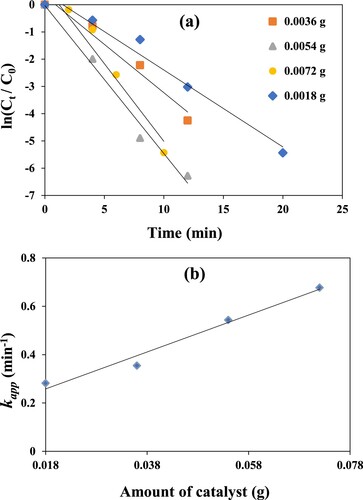
3.5. Effect of temperature
Temperature is an essential factor that affects the rate of reaction. The rate of diffusion of reactant molecules to the catalyst surface is highly influenced by temperature. Temperature increases the average kinetic energy of reactant molecules. It also gives information about the thermodynamics and energetic of catalytic reaction.
In this study, temperature’s effect on the catalytic efficiency of Co nanoparticles-based graphene oxide was also examined by performing reduction reaction at four temperatures of 30°C, 40°C, 50°C and 60°C, maintaining other parameters constant. It is clear from Figure (a) that a good linear trend of plots of ln (Ct/Co) against time, t, was noticed at all mentioned temperatures. Moreover, with the increase in temperature from 30°C to 60°C, the value of kapp increased from 0.20 to 0.58 min−1 (3.3 × 10−3–9.6 × 10−3 s−1). Furthermore, the consistency in linear trend illustrates the uniform distribution of Co nanoparticles on the surface of GO sheets. The experimental data obtained from temperature studies were also used for the calculation of the activation energy (Ea) of the reduction reaction as shown in Figure (b). Ea value was obtained by plotting ln kapp versus (1/T) (Arrhenius equation) and its value came out to be 28.79 kJ/mol. Experimental values from the temperature’s effect on the reduction reaction were further used to calculate other thermodynamic parameters, i.e. activation enthalpy, activation entropy and Gibbs free energy. Enthalpy and entropy values were calculated from the Eyring equation plot (ln k/T vs 1/T). From Figure (c), the enthalpy and entropy of activation for the reduction reaction of 4-NP using GO/Co nanocatalyst were found to be +26.18 kJ mol−1 and −206.26 J mol−1 K−1. Positive enthalpy value indicates the reduction process to be endothermic and the negative entropy value for reduction process depicts an associative mechanism for the formation of activated complex. While the positive Gibbs free energy, ΔG, values at all the mentioned temperatures (Figure d) depict non-spontaneity and non-feasibility of the reduction process under study. It’s obvious because of the huge activation energy barrier between reactants and products. However, by employing nanocatalyst, an alternate lower activation energy path between electron-rich and electron-deficient site makes reduction reaction feasible. These thermodynamic values are also in consistent with previous findings of the reduction process by graphene-based nanocomposites [Citation48].
Figure 7. (a) Pseudo first-order plots for the reduction of 4-NP catalysed by GO–Co nanocomposite at 30°C, 40°C, 50°C and 60°C. (b) Arrhenius plot for the determination of activation energy (Ea), (c) Eyring plot for the determination of thermodynamic parameters, for catalytic reduction the 4-NP using GO–Co nanocatalyst. (d) Plot of ΔG as a function of temperature. Reaction conditions; 1 mM aqueous solution of 4-Nitrophenol = 30 ml, NaBH4 = 0.113 g, GO–Co catalyst = 3.6 mg, 100 rpm.
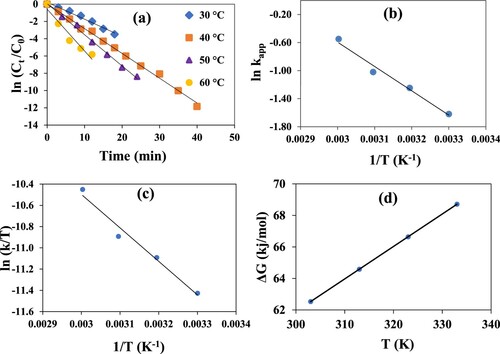
Prepared GO–Ag nanocomposite was also checked by carrying out reduction reaction of 4-NP using NaBH4 as a reducing agent under same operating conditions as used for GO–Co and same parameters were investigated. The UV–Vis absorption spectra for the gradual reduction of 4-NP over GO–Ag catalyst is shown in Figure (a). The catalytic efficiency of Ag-based graphene oxide was examined at two temperatures, i.e. 30°C and 60°C to compute the activation energy value of reduction reaction. The value of kapp was observed to get an increase from 0.3623 to 0.6547 min−1 (6.03 × 10−3–10.9 × 10−3 s−1) which is much greater than that in the case of GO–Co nanocomposite. Further, activation energy (Ea) was calculated using the Arrhenius equation at two different temperatures as shown in Figure (b) and came out to be 16.54 kJ/mol, much less than that we observed for GO–Co. The catalytic performance of GO–Co and GO–Ag in the reduction of 4-NP can be compared by considering the corresponding values of kapp and Ea. Relatively higher values of kapp both at lower and higher temperatures for the reduction of 4-NP catalysed with GO–Ag as compared to GO–Co predict that for this catalytic reaction (4-NP reduction), GO–Ag proved to be a far effective catalyst as compared to GO–Co. The Ea for the reduction of 4-NP catalysed with GO–Ag was also lower as compared to that observed GO–Co which also favours the higher reduction rate of this reaction in the presence of GO–Ag. These observations are very much related with previously reported results [Citation44–46]. The catalytic reduction was assumed to take place via Langmuir–Hinshelwood mechanism as reported earlier in the literature [Citation49]. Figure shows a schematic of catalytic reduction of 4-NP by the prepared nanocatalyst. Both the electron donor BH4¯ and acceptor 4-NP ion are adsorbed at the surface of nanoparticle integrated on GO sheet. First, BH4¯ donates electrons to the catalyst and produces hydride ions which then react with protons provided from water. This reaction results in the formation of hydrogen molecules which are further converted into active hydrogen atoms on the surface of nanoparticles. The active hydrogen atoms attack nitro groups of 4-NP adsorbed on the surface of nanoparticles. The reduction of 4-NP is then carried out through the formation of the intermediates containing nitroso (–NO) and hydroxylamino groups (–NHOH) as shown by the structural formulas on the surface of nanoparticles in Figure . Once 4-NP is reduced to the corresponding aminophenol, the aminophenol leaves the surface of nanoparticles. In this way, the surface of nanoparticles is regenerated for the next turn and so on.
3.6.. Catalyst reusability
The reusability of a catalyst is a significant parameter from the economical viewpoint and industrial applications. Therefore, the reusability of our prepared catalyst was also studied. The prepared nanocatalyst was recycled and used again and again for four cycles in a row. After completion of each cycle, insoluble nanocatalyst was removed from the reaction system by filtration, washed thoroughly with plenty of DDW and then it was reused for another reduction cycle of 4-NP [Citation50]. In this way, four consecutive cycles were performed. The catalytic activity decreased to 43% as we reached in the fourth consecutive run. This can happen possibly due to the loss of some amount of catalyst during the filtration and washing process. Figure shows the results of reusability of GO–Co nanocomposite for four consecutive runs and that of GO–Ag nanocomposite catalysts for five consecutive runs in terms of a plot between % activity and cycle number. The reusability of catalyst was checked for both the prepared nanocomposites under same reaction conditions. Although, as depicted from Figure , a decrease was observed in the catalytic activity upon recycling, but it is obvious because of the lifetime of every product. Surface roughness, occupation of active sites by any moieties other than reactants, oxidation of the metal nanoparticles and leaching of the catalytically active metal nanoparticles from the prepared composite catalyst may lead to suppression in the activity in every successive cycle [Citation51]. The plausible reason for the better performance of GO–Ag in recycling may be due to the more negative reduction potential of Cobalt ions (−0.29V) as compared to that of Ag ions (+0.81V) and due to this, Co+2 can readily be oxidized as compared to Ag+ forming metal oxide layer at metal nanocatalyst surface, thus hindering its catalytic activity. Due to this hindrance in the interaction between catalyst and substrate, rate of activated complex formation and thus rate of catalytic activity also get reduced in case of GO–Co. So, in addition to better performance in terms of kapp, GO–Ag was also found better catalyst than Go–Co in terms of recycling in the reduction of 4-NP.
3.7. Catalytic test using water from different resources
The catalytic efficiency of GO–Co nanocomposite was also evaluated in water samples obtained from different resources, i.e. DDW, tap water and dam water. 4-nitrophenol solution was prepared using these water samples as a solvent separately and catalytic behaviour was reported with the results in Figure . Results showed that catalytic reduction behaviour of GO–Co nanocomposite was rapid in DDW owing to the fact that DDW is free from impurities that can possibly block active sites on nanocomposite surface. However, a much-decreased catalytic behaviour of nanocomposite in dam water may be due to higher number of interfering impurities that block the active catalytic sites [Citation52,Citation53]. Catalytic behaviour of GO–Ag nanocatalyst was also checked using water from different sources just like in the case of GO–Co and results are also shown in Figure . Similar results were observed in the case of GO–Co that 4-NP reduction is highest in DDW and lowest in dam water. However, the magnitude of kapp was much higher with GO–Ag in every water sample as compared with GO–Co, confirming the high working efficiency of GO–Ag nanocomposite in the present study.
3.8. Catalytic test of GO–Co and GO–Ag on different nitro compounds
For catalytic reduction of water pollutant, an ideal catalyst system should have the ability to reduce the variety of pollutants from polluted water. So, to check this, GO–Co catalyst system was investigated for the reduction of 2-NP and 2-NA other than 4-NP and the corresponding UV–Vis spectra are shown in Figure (a) and (b), respectively. The values of kapp were calculated by pseudo first-order kinetics and given in Figure for the reduction of all the three nitrocompounds. When all the three aromatic nitrocompounds were reduced separately then it was observed that catalyst effectively reduce all the nitro pollutants but with different completion time and hence different activity. Reduction time of 2-NA, 2-NP and 4-NP under the same operating conditions were observed to be 60, 40 and 20 min, respectively, indicating much efficient 4-NP reduction by GO–Co. The possible reason for the fast reduction of 4-NP as compared to other nitro pollutants is its facile approach to the active sites of catalyst owing to the nitro group at its para position. The reactants with nitro groups at ortho positions, i.e. 2-NA and 2-NP may face any hindrance because of relatively less suitable orientation [Citation52]. The combined benefits of easy fabrication, good catalytic response toward different nitro pollutants and reasonable reusability suggests that GO–Co can be used in industries as a versatile and cost-effective catalyst for the reduction of nitrocompounds. GO–Ag nanocatalyst was also checked for the reduction of 2-NA, 2-NP in addition to 4-NP and results followed similar trends to that we observed in the case of GO–Co (Figure ). However, the reduction time of 2-NA, 2-NP and 4-NP under the same operating conditions were 18, 20 and 16 min respectively, much less than that in the case of GO–Co, further confirming the superiority of GO–Ag catalytic behaviour over GO–Co. So, the present research work demonstrates that GO–Ag nanocomposite is far effective nanocatalyst than GO–Co. Results also confirmed that more silver nanoparticles are exposed over the surface of graphene oxide and hence are more involved in the catalytic reaction as compared to later, where Co nanoparticles might get more entrapped within graphene layers thus suppressing its catalytic activity. This is also in correlation with previously reported results. Further, the electronic configuration and position of silver and cobalt in the periodic table seem to strengthen the observed results in the present study. The electronic configuration of cobalt is [Ar] 3d7 4s2 and that of silver is [Kr] 4d10 5s1. An effective and efficient catalyst can take and can also give electrons with ease. In case of cobalt, it easily gains electron from BH4¯ which is a strong nucleophile and electron goes into the inner 3d7 orbital and hence experience strong nuclear attraction, so it becomes difficult for Co to give that electron with ease to H+ thus suppressing its catalytic efficiency. Whereas in the case of Ag, electron from BH4¯ goes into outer 5s1 orbital so electron experiences weak nuclear pull and hence it becomes easy for Ag to lose that electron easily to H+ for catalytic reduction reaction [Citation54]. Consequently, the catalytic efficiency of Ag nanocomposite is much more pronounced than Co nanocomposite. The results of catalytic reduction for all the three studied compounds, i.e. 4-NP, 2-NP and 2-NA were also compared with recently published literature and the comparison has been presented in Table . The comparison shows that the reduction rates observed in the present work were much better as compared to recently published work.
Figure 12. Time-dependent UV–Vis absorption spectra of (a) 2-NP and (b) 2-NA catalysed with GO–Co nanocatalyst. Reaction conditions: 1 mM aqueous solution of nitrocompound = 30 ml, NaBH4 = 0.113 g, nanocatalyst = 3.6 mg, 100 rpm.
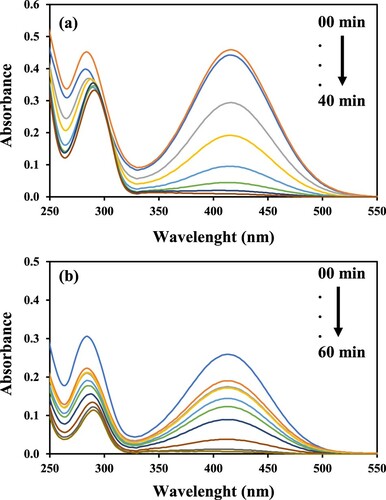
Figure 13. Catalytic efficiency of GO–Ag and GO–Co nanocatalysts for the reduction of different nitrocompounds. Reaction conditions: 1 mM aqueous solution of nitrocompound = 30 ml, NaBH4 = 0.113 g, nanocatalyst = 3.6 mg, 100 rpm.
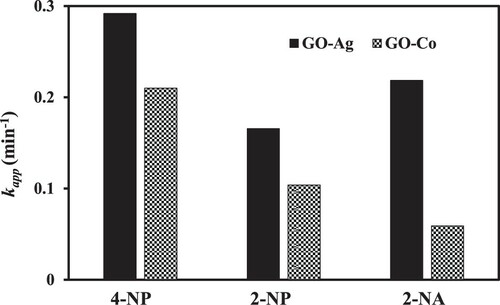
Table 1. Comparison of the kapp calculated in the present work with the reported literature.
4. Conclusion
Metal nanoparticles of Co and Ag were successfully synthesized by chemical reduction method on GO sheets which were readily prepared by modified Hummers method. Almost evenly distributed nanoparticles of Ag and Co were obtained on GO sheets and the diameters of most of the particles were in the range of 5–15 nm. Increased thermal stability was exhibited by GO–Co and GO–Ag nanocomposites as compared to GO. The prepared GO–Co and GO–Ag have shown venerable catalytic activity towards the reduction of 4-NP, 2-NP and 2-NA. With the aid of GO–Ag and GO–Co nanocomposite catalysts, the reduction rates of 4-NP were found as high as 0.66 and 0.58 min−1, respectively while the corresponding activation energies were found as 16.54 and 28.79 kJ mol−1, respectively. The positive value of activation enthalpy change (26.18 kJ/mol) for the reduction of 4-NP catalysed by GO–Co nanocomposite showed that the reaction was endothermic while negative value of activation entropy change (−206.26 J mol−1 K−1) depicted that the formation of the activated complex was followed by association mechanism. The apparent reduction rates for GO–Co catalyzed reduction of 2-NP and 2-NA were found as 0.10 and 0.06 min−1, respectively. Both the GO–Ag and GO–Co nanocomposite catalysts retained their activity for the reduction of 4-NP in tap water with corresponding kapp values of 0.12 and 0.08 min−1, respectively, as well as in dam water with corresponding kapp values of 0.02 and 0.05 min−1, respectively. Catalytic activity of GO–Ag was decreased by 25% in the fifth cycle while that of GO–Co was decreased to 47% in the fourth cycle for the reduction of 4-NP under the same set of reaction conditions. The comparison of the two prepared catalysts suggests that GO–Ag nanocomposite is a better catalyst than GO–Co nanocomposite for the nitrocompound reduction. However, owing to much lower cost GO–Co nanocomposite can be given priority over GO–Ag nanocomposite with a little compromise on reduction rate and recycling.
Disclosure statement
No potential conflict of interest was reported by the author(s).
References
- Chiou J-R, Lai B-H, Hsu K-C, et al. One-pot Green synthesis of silver/iron oxide composite nanoparticles for 4-nitrophenol reduction. J Hazard Mater. 2013;248:394–400.
- Zhang B, Xu P, Xie X, et al. Acid-directed synthesis of SERS-active hierarchical assemblies of silver nanostructures. J Mater Chem. 2011;21(8):2495–2501.
- Chang Y-C, Chen D-H. Catalytic reduction of 4-nitrophenol by magnetically recoverable Au nanocatalyst. J Hazard Mater. 2009;165(1–3):664–669.
- Hayakawa K, Yoshimura T, Esumi K. Preparation of gold− dendrimer nanocomposites by laser irradiation and their catalytic reduction of 4-nitrophenol. Langmuir. 2003;19(13):5517–5521.
- Naik B, Hazra S, Prasad VS, et al. Synthesis of Ag nanoparticles within the pores of SBA-15: an efficient catalyst for reduction of 4-nitrophenol. Catal Commun. 2011;12(12):1104–1108.
- Yamamoto H, Yano H, Kouchi H, et al. N, N-dimethylformamide-stabilized gold nanoclusters as a catalyst for the reduction of 4-nitrophenol. Nanoscale. 2012;4 (14):4148–4154.
- Naseem K, Farooqi ZH, Begum R, et al. Systematic study for catalytic degradation of nitrobenzene derivatives using core@ shell composite micro particles as catalyst. Colloids Surf A. 2020;124646.
- Feng J, Su L, Ma Y, et al. Cufe2o4 magnetic nanoparticles: a simple and efficient catalyst for the reduction of nitrophenol. Chem Eng J. 2013;221:16–24.
- Ghosh SK, Mandal M, Kundu S, et al. Bimetallic Pt–Ni nanoparticles can catalyze reduction of aromatic nitro compounds by sodium borohydride in aqueous solution. Appl Catal A. 2004;268(1–2):61–66.
- Lu Y, Mei Y, Schrinner M, et al. In situ formation of Ag nanoparticles in spherical polyacrylic acid brushes by UV irradiation. J Phys Chem C. 2007;111(21):7676–7681.
- Das TK, Remanan S, Ghosh S, et al. An environment friendly free-standing cellulose membrane derived for catalytic reduction of 4-nitrophenol: A sustainable approach. J Environ Chem Eng. 2021;9(1):104596.
- Das TK, Ganguly S, Bhawal P, et al. A facile Green synthesis of silver nanoparticle-decorated hydroxyapatite for efficient catalytic activity towards 4-nitrophenol reduction. Res Chem Intermed. 2018;44(2):1189–1208.
- Baran T, Nasrollahzadeh M. Facile synthesis of palladium nanoparticles immobilized on magnetic biodegradable microcapsules used as effective and recyclable catalyst in Suzuki–Miyaura reaction and p-nitrophenol reduction. Carbohydr Polym. 2019;222:115029.
- Baran NY, Baran T, Nasrollahzadeh M, et al. Pd nanoparticles stabilized on the Schiff base-modified boehmite: catalytic role in Suzuki coupling reaction and reduction of nitroarenes. J Organomet Chem. 2019;900:120916.
- Shokouhimehr M, Yek SM-G, Nasrollahzadeh M, et al. Palladium nanocatalysts on hydroxyapatite: Green oxidation of alcohols and reduction of nitroarenes in water. Applied Sciences. 2019;9(19):4183.
- Nasrollahzadeh M, Issaabadi Z, Safari R. Synthesis, characterization and application of Fe3O4@ SiO2 nanoparticles supported palladium (II) complex as a magnetically catalyst for the reduction of 2, 4-dinitrophenylhydrazine, 4-nitrophenol and chromium (VI): a combined theoretical (DFT) and experimental study. Sep Purif Technol. 2019;209:136–144.
- Nasrollahzadeh M, Issaabadi Z, Sajadi SM. Green synthesis of Pd/Fe3O4 nanocomposite using Hibiscus tiliaceus L. extract and its application for reductive catalysis of Cr (VI) and nitro compounds. Sep Purif Technol. 2018;197:253–260.
- Naghdi S, Sajjadi M, Nasrollahzadeh M, et al. Cuscuta reflexa leaf extract mediated Green synthesis of the Cu nanoparticles on graphene oxide/manganese dioxide nanocomposite and its catalytic activity toward reduction of nitroarenes and organic dyes. J Taiwan Inst Chem Eng. 2018;86:158–173.
- Li B, Cao H, Yin G, et al. Cu2O@ reduced graphene oxide composite for removal of contaminants from water and supercapacitors. J Mater Chem. 2011;21(29):10645–10648.
- Kamal A, Srinivasulu V, Murty JC, et al. Copper oxide nanoparticles supported on graphene oxide-catalyzed S-Arylation: an efficient and ligand-free synthesis of aryl sulfides. Adv Synth Catal. 2013;355(11-12):2297–2307.
- Wang S, Peng Y. Natural zeolites as effective adsorbents in water and wastewater treatment. Chem Eng J. 2010;156(1):11–24.
- Sun J, Fu Y, He G, et al. Catalytic hydrogenation of nitrophenols and nitrotoluenes over a palladium/graphene nanocomposite. Catal Sci Technol. 2014;4(6):1742–1748.
- Meng L, Zhang X, Tang Y, et al. Hierarchically porous silicon–carbon–nitrogen hybrid materials towards highly efficient and selective adsorption of organic dyes. Sci Rep. 2015;5(1):1–16.
- Nasrollahzadeh M, Issaabadi Z, Tohidi MM, et al. Recent progress in application of graphene supported metal nanoparticles in C− C and C− X coupling reactions. Chem Rec. 2018;18(2):165–229.
- Balandin AA, Ghosh S, Bao W, et al. Superior thermal conductivity of single-layer graphene. Nano Lett. 2008;8(3):902–907.
- Geim AK, Novoselov KS. The rise of graphene. Nat Mater. 2007;6(3):183–191.
- Naeem H, Ajmal M, Muntha S, et al. Synthesis and characterization of graphene oxide sheets integrated with gold nanoparticles and their applications to adsorptive removal and catalytic reduction of water contaminants. RSC Adv. 2018;8(7):3599–3610.
- Li P, Lu P, Zhai Y, et al. Low temperature SCR of NO with catalysts prepared by modified ACF loading Mn and Ce: effects of modification method. Environ Technol. 2015;36(18):2390–2400.
- Das TK, Bhawal P, Ganguly S, et al. A facile Green synthesis of amino acid boosted Ag decorated reduced graphene oxide nanocomposites and its catalytic activity towards 4-nitrophenol reduction. Surfaces and Interfaces. 2018;13:79–91.
- Fakhri P, Mahmood H, Jaleh B, et al. Improved electroactive phase content and dielectric properties of flexible PVDF nanocomposite films filled with Au-and Cu-doped graphene oxide hybrid nanofiller. Synth Met. 2016;220:653–660.
- Karimi-Maleh H, Shafieizadeh M, Taher MA, et al. The role of magnetite/graphene oxide nano-composite as a high-efficiency adsorbent for removal of phenazopyridine residues from water samples, an experimental/theoretical investigation. J Mol Liq. 2020;298:112040.
- Bagherzadeh M, Amrollahi M, Makizadeh S. Decoration of Fe3O4 magnetic nanoparticles on graphene oxide nanosheets. RSC Adv. 2015;5(128):105499–105506.
- Hassandoost R, Pouran SR, Khataee A, et al. Hierarchically structured ternary heterojunctions based on Ce3+/Ce4+ modified Fe3O4 nanoparticles anchored onto graphene oxide sheets as magnetic visible-light-active photocatalysts for decontamination of oxytetracycline. J Hazard Mater. 2019;376:200–211.
- Nasrollahzadeh M, Jaleh B, Jabbari A. Synthesis, characterization and catalytic activity of graphene oxide/ZnO nanocomposites. RSC Adv. 2014;4(69):36713–36720.
- Golikand AN, Bagherzadeh M, Shirazi Z. Evaluation of the polyaniline based nanocomposite modified with graphene nanosheet, carbon nanotube, and Pt nanoparticle as a material for supercapacitor. Electrochim Acta. 2017;247:116–124.
- Ahmadi N, Nemati A, Bagherzadeh M. Synthesis and properties of Ce-doped TiO2-reduced graphene oxide nanocomposite. J Alloys Compd. 2018;742:986–995.
- Ahmadi N, Bagherzadeh M, Nemati A. Comparison between electrochemical and photoelectrochemical detection of dopamine based on titania-ceria-graphene quantum dots nanocomposite. Biosens Bioelectron. 2020;151:111977.
- Nasrollahzadeh M, Nezafat Z, Gorab MG, et al. Recent progresses in graphene-based (photo) catalysts for reduction of nitro compounds. Mol Catal. 2020;484:110758.
- Naeem H, Ajmal M, Qureshi RB, et al. Facile synthesis of graphene oxide–silver nanocomposite for decontamination of water from multiple pollutants by adsorption, catalysis and antibacterial activity. J Environ Manage. 2019;230:199–211.
- Zhang K. Fabrication of copper nanoparticles/graphene oxide composites for surface-enhanced Raman scattering. Appl Surf Sci. 2012;258(19):7327–7329.
- Das TK, Ganguly S, Remanan S, et al. Mussel-inspired Ag/poly (norepinephrine)/MnO2 heterogeneous nanocatalyst for efficient reduction of 4-nitrophenol and 4-nitroaniline: an alternative approach. Res Chem Intermed. 2020;46(7):3629–3650.
- Vi T, Lue S. In preparation of silver nanoparticles loaded graphene oxide nanosheets for antibacterial activity). IOP conference series: materials science and engineering. IOP Publishing. 2016;012033.
- Barman BK, Nanda KK. The dual role of Zn–acid medium for one-step rapid synthesis of M@ rGO (M = Au, Pt, Pd and Ag) hybrid nanostructures at room temperature. Chem Commun. 2013;49(79):8949–8951.
- Wojtoniszak M, Zielinska B, Kalenczuk R, et al. Photocatalytic performance of titania nanospheres deposited on graphene in coumarin oxidation reaction. Mater Sci Poland. 2012;30(1):32–38.
- Hoseini SJ, Rashidi M, Bahrami M. Platinum nanostructures at the liquid–liquid interface: catalytic reduction of p-nitrophenol to p-aminophenol. J Mater Chem. 2011;21(40):16170–16176.
- Cheng Z, Liao J, He B, et al. One-step fabrication of graphene oxide enhanced magnetic composite gel for highly efficient dye adsorption and catalysis. ACS Sustain Chem Eng. 2015;3(7):1677–1685.
- Farooqi ZH, Khalid R, Begum R, et al. Facile synthesis of silver nanoparticles in a crosslinked polymeric system by in situ reduction method for catalytic reduction of 4-nitroaniline. Environ Technol. 2019;40(15):2027–2036.
- Wang X, Pan J, Guan W, et al. Selective removal of 3-chlorophenol from aqueous solution using surface molecularly imprinted microspheres. J Chem Eng Data. 2011;56(6):2793–2801.
- Ajmal M, Aftab F, Bibi I, et al. Facile synthesis of porous anionic hydrogel embedded with nickel nanoparticles and evaluation of its catalytic performance for the rapid reduction of 4-nitrophenol. J Porous Mater. 2019;26(1):281–290.
- Fakhri P, Jaleh B, Nasrollahzadeh M. Synthesis and characterization of copper nanoparticles supported on reduced graphene oxide as a highly active and recyclable catalyst for the synthesis of formamides and primary amines. J Mol Catal A Chem. 2014;383:17–22.
- Ajmal M, Demirci S, Siddiq M, et al. Simultaneous catalytic degradation/reduction of multiple organic compounds by modifiable p (methacrylic acid-co-acrylonitrile)–M (M: Cu, Co) microgel catalyst composites. New J Chem. 2016;40(2):1485–1496.
- Ajmal M, Siddiq M, Al-Lohedan H, et al. Highly versatile p (MAc)–M (M: Cu, Co, Ni) microgel composite catalyst for individual and simultaneous catalytic reduction of nitro compounds and dyes. RSC Adv. 2014;4(103):59562–59570.
- Ajmal M, Demirci S, Siddiq M, et al. Betaine microgel preparation from 2-(methacryloyloxy) ethyl] dimethyl (3-sulfopropyl) ammonium hydroxide and its use as a catalyst system. Colloids Surf A. 2015;486:29–37.
- Farooqi ZH, Iqbal S, Khan SR, et al. Cobalt and nickel nanoparticles fabricated p (NIPAM-co-MAA) microgels for catalytic applications. e-Polymers. 2014;14 (5):313–321.
- Korobeinyk AV, Whitby RD, Mikhalovsky SV, et al. In situ production of high purity noble metal nanoparticles on fumed silica and catalytic activity towards 2-nitrophenol reduction. J Phys Chem Solids. 2019;127:28–34.
- Ko JW, Li J, Suzuki A, et al. Reduction of 2-nitrophenol using a hybrid C–Ni nanocomposite as a catalyst. Micro Nano Lett. 2018;13(9):1310–1314.
- Farooqi ZH, Naseem K, Begum R, et al. Catalytic reduction of 2-nitroaniline in aqueous medium using silver nanoparticles functionalized polymer microgels. J Inorg Organomet Polym Mater. 2015;25(6):1554–1568.
- Zelekew OA, Kuo D-H. Facile synthesis of SiO2@ CuxO@ TiO2 heterostructures for catalytic reductions of 4-nitrophenol and 2-nitroaniline organic pollutants. Appl Surf Sci. 2017;393:110–118.