Abstract
The frosting processes on the wing surface before and after removing the scale of the Catocala electa Borkhauson were observed by using a self-assembled experiment device. The results showed that the formation process of frosting on the wing surface with or without scales was similar. However, the scale could affect the attachment shape of water vapour on the wing surface and the time when frost began to form. The formation time of frosting on the wing surface without scales was earlier than that on the wing surface with scales, which was about 614 s. The influence mechanism of the scales on the frosting process was analyzed from the wettability and morphology of the scales on the wing surface Catocala electa Borkhauson. The scale on the wing surface of Catocala electa Borkhauson showed superhydrophobic properties, and the scale surface had criss-cross ribs, which formed many special micro-nano spaces. Superhydrophobicity could promote the rolling of water on the scales and reduce the adhesion area between water and scale surface. The special space structure could delay the cooling of droplets on the scale surface of Catocala electa Borkhauson. The study might be helpful to design or optimize the functional surfaces with anti-frosting properties based on the structure of the scale surface.
1. Introduction
The frosting is a common phenomenon, which is common in many fields, especially in an environment with low temperature and high humidity. The increase in the thickness of the frost layer on the surface of the component will help the formation of ice on the material surface under certain conditions. The layer of frost or ice formed on the surface will be harmful to the engineering field, such as aerospace, refrigeration, telecommunication, etc. [Citation1–4]. For example, the frost layer formed on the surface of the aircraft wing increases flight resistance and affects operational safety [Citation5,Citation6]. The surface of the evaporator, heat exchanger, heat pump, condenser, and other refrigeration equipment is easy to frost, which reduces the heat exchange efficiency and the cooling rate, increasing energy consumption and economic costs [Citation7–10]. When the frost layer forms on the surface of the telecommunication components, such as antennas and radars, it interferes with the intensity and accuracy of the signal transmission and the response sensitivity of the system [Citation11]. The operational performance of the wind turbine located in the high altitude area is also affected by frosting [Citation12].
Hence, anti-frosting methods have been developed and applied in the engineering field to minimize the hazards of frosting or ice formed by the frost layer. Based on anti-frosting principles, these methods can be separated into three categories: mechanical methods, heating approaches, and chemical methods [Citation8,Citation13–16]. Although the conventional methods can get good anti-frost effects, they are often at the cost of high energy consumption, high cost, environmental pollution, and corrosion parts [Citation13,Citation15,Citation16]. For example, electric heating de-frosting enhances the use cost of the equipment and improves the complexity of the device. Many researchers have carried out the mechanism analysis of the frost formation and its influencing factors to reduce the shortcomings of the existing anti-frosting methods and develop new strategies. Based on the observation of the frosting process on the cold metal surface, Hayashi et al. [Citation17] and Tao et al. [Citation18] divided the frost formation process into three stages: the frost crystals formation, the frost layer growth, and the full growth. Na and Webb [Citation19], and Piucco et al. [Citation20] analyzed phase transition kinetics in the process of frost crystal nucleation to better understand the frosting mechanism. In order to better understand the mechanism of frost formation and develop new anti-frosting methods, the researchers took the finite element simulation and mathematical modeling to predict the frost formation process and its growth rate on the cold surface [Citation21–26]. And the proposed prediction model has good consistency with the experimental results. Meanwhile, the effect of influencing factors on the frosting process was investigated, such as the ambient air relative humidity, temperature, and cold surface temperature, air quality, atmospheric pressure [Citation27–30].
However, due to the discovery of the lotus leaf effect and the development of material preparation technology, many scholars have devoted themselves to improving the wettability of the material surface to inhibit the accumulation of water vapour on the material surface and interfering with the formation of frost on the material surface [Citation31,Citation32]. The superhydrophobic surface is one of the promising anti-frosting methods that can reduce the amount of water vapour absorbed on the surface. However, the published literature has shown that the superhydrophobic surface has limitations, such as the destruction of superhydrophobic surface microstructure, anti-icing durability, mechanical properties, and pollution resistance [Citation33–39]. Hence, if the superhydrophobic surface is used in actual use, these shortcomings should be overcome.
After millions of years of evolution, the creatures in nature have developed the ability to adapt to the environment and maintain survival. For example, the water strider can walk on water due to the leg with the superhydrophobic property. Since the biological surface has formed the self-cleaning characteristic, butterflies, dragonflies, moths, and other organisms living in a humid environment, such as grassland and forest, can ensure sufficient flight ability in rainy weather. Anti-frosting performance is very important for organisms living in moist environments. Many studies on the microstructure and wetting characteristics of the biological surface have been carried out to fabricate the superhydrophobic surface [Citation40–42]. Yuan et al. [Citation40] used a natural taro leaf as a template to fabricate a polystyrene film with superhydrophobic properties. Zang et al. [Citation42] found that the surface of the lotus seedpod exhibited superhydrophobic property, and the static contact angle of the water droplet on the surface could reach 153.9° ± 2.7°. Inspired by this, a bionic AZ91D Mg alloy super-hydrophobic surface was prepared. Moreover, researchers have also been inspired by the surface properties of some organisms to prepare functional surfaces with hydrophobic surfaces, such as mosquito eyes, butterfly wings, and rice leaves [Citation43–46]. Li and Guo have analyzed the anti-icing strategies and mechanisms of superhydrophobic surfaces from the characteristics of biological surfaces [Citation46]. However, there are few studies on the influence of scales on the anti-frosting and hydrophobic properties of the moth.
As one kind of moth, Catocala electa Borkhauson often lives in a humid environment and is mainly distributed in northern China, such as Changchun, Harbin, Dandong, etc. Autumn in the north of China starts early, the temperature difference between day and night is significant, and frost is prone to appear in the morning in autumn. Hence, Catocala electa Borkhauson was regarded as the sample during the study. The study took the comparative experiment method, and the purposely built microscopic observation device was used to observe the frosting process on the wing surface of the Catocala electa Borkhauson. Based on the experimental results, the influence mechanism of scales on the anti-frost and hydrophobic properties of biological samples was analyzed. The study provides an experimental basis for preparing bionic anti-frosting materials and analyzing the hydrophobic mechanism with scales.
2. Materials and methods
2.1 Materials
The Catocala electa Borkhauson belongs to the 10th species of Noctuidae, which is not a nationally protected or rare species [Citation47]. During the experiment, the adult samples of Catocala electa Borkhauson were collected from Changchun, P. R. China (Nanhu Park, Animal and Botanical Park, Jingyuetan Park). Regarding the literature [Citation48], the collected samples were distinguished and identified as Catocala electa Borkhauson by the entomologists. Adult biological samples were used in the experiment (as shown in Figure ), and the rest have been released into nature.
A copper sheet (Xingye Copper International Group Limited) with a diameter of 6 mm and a thickness of 0.5 mm was used to fix the biological sample. The intercepted wings of Catocala electa Borkhauson with a size of approximately 3 mm × 2 mm (L×W) were pasted on the copper sheet surface.
2.2 Experimental details
2.2.1 Test apparatus
The frosting process on the sample surface was observed by the self-made apparatus. As shown in Figure , the observation device was composed of a microscope with a CCD camera, PC with the collection system, refrigerated state, DC power, and a climate chamber. The climate chamber was used to control the environmental temperature and humidity of the observation test. The refrigeration state was at different temperatures by adjusting the DC power voltage, and the lowest temperature could reach −20 °C. The frosting process on the sample surface was collected through a microscope with a CCD camera simultaneously. The time interval between the two collected pictures was one second.
Figure 2. Experimental apparatus for observation of the frosting process on the sample surface. 1. PC (acquisition system); 2. temperature controller; 3. DC power; 4. climate chamber; 5. microscopic observation device; 6. microscope with a CCD camera; 7. sample; 8. cooling state; 9. copper plater with 3M thermally conductive tape.
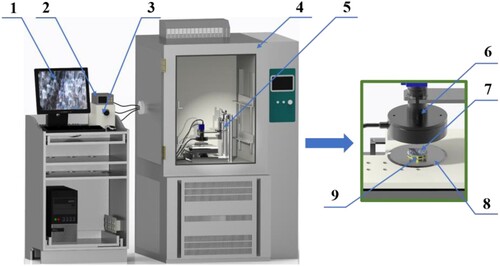
2.2.2 Experiment conditions
Surface hydrophobicity is often characterized by the rolling angle and contact angle; however, the rolling angle is always used to evaluate the self-cleaning ability of the material surface. During the experiment, the static angle of water on the sample surface was regarded as the evaluation standard for the sample wettability. A contact angle measurement system (OCA 30-2, Data-physics Instruments Gmbh, Germany) with a base device was adopted to collect the static angle, and the average value was calculated.
To remove the scales on the wing surface, the wing surface of Catocala electa Borkhauson was vibrated firstly and then soaked in pure water for 3 h. During the experiment, the wing surface of Catocala electa Borkhauson was sliced and pasted on the copper surface with 3M 8810 thermal conductive tape. In the test of the influence of the scales on the frosting process of Catocala electa Borkhauson, the temperature and humidity of the test environment were set as T1 = 18 °C and Rh = 80% by using the climate chamber, respectively. The surface temperature of the cooling state reached T2 = −15 °C by adjusting the refrigeration controller and was monitored by a K-type thermocouple. The observation device was placed in the climate chamber. The control accuracy of temperature and humidity is ±0.5 °C and ±2.5% RH, respectively.
3. Results
3.1 Frost formation on wings with scales
The frosting process on the wing surface with the scales was shown in Figure . With the continuous cooling of the wing surface, there was a large temperature difference between the wing surface temperature and the ambient temperature. Due to the effect of temperature difference, the water vapour in the environment continuously adhered to the wing surface and gradually formed smaller and sparsely distributed water droplets with a spherical shape, as shown in Figure (images a∼d). Owing to the small attachment area between the water droplets and the wing surface, the small water droplets that were continuously condensed under the drive of the gravity of the water droplets roll and fall off the wings of the Catocala electa Borkhauson. The small water droplets attached to the wing surface were taken away and merged to form larger water droplets during the rolling process. The water droplets no longer rolled and reached a stable state until the gap between the adjacent scales.
As shown in Figure (images e∼f), the droplets from 1 to 8 on the fin table were combined to form larger droplets A, B, C, and D, respectively. Meanwhile, the vapour in the environment continued to adhere and condense on the wing surface with scales and formed many small droplets. Due to the droplets with different volumes attached to the wing surface, the small droplets began to phase change and freeze into ice from the freezing time of 884 s to 944 s. The larger droplets did not occur during the phase transformation and were still in the supercooled state during this period. At the same time, frost crystals began to appear on the wing surface of Catocala electa Borkhauson. With the continuous cooling of the wing surface, frost crystals formed on the wing surface, and the frost layer became thicker gradually. Meanwhile, vapour in the environment continued to adhere to the scales and was phased into ice. Repeatedly, the frost layer on the surface of the wings gradually thickened, as shown in Figure . l.
The formation process of frost on the wing surface with scale was summarized, as shown in Figure . The frosting process on the wing surface with scales could be separated into six stages: vapour condensation, droplets growth, coalescence and freezing into ice, and frost crystal formation and frosty layer growth. Moreover, the droplets were distributed in a spherical shape on the scale surface. It illustrated that the scale surface of the wing surface of Catocala electa Borkhauson had a superhydrophobic performance.
3.2 Frost formation on wings without scales
Compared with the frosting process on the wing surface with scales, the frosting process on the wing surface after scale removal was observed, as shown in Figure . The vapour gradually condensed on the surface without scales to form droplets, which were densely distributed and increased continuously, as shown in Figure (images a∼d). It could be seen from Figure (images e∼g) that the small droplets rolled on the surface with the scales removed and continued to merge to form larger water droplets. It would result in a water film on the wing surface without scales of the Catocala electa Borkhauson. After a short period of refrigeration, the droplets attached to the surface without scale began to undergo a phase change and freeze into ice, as shown in Figure . h. With the continuous cooling, the frost crystals appeared on the wing surface without scales and continued to increase in volume, as shown in Figure (images i∼l). Additionally, a frost layer with uneven thickness was formed.
Figure was the formation process of frost on the wing surface without scales. Combined Figures and , the frosting process on the wing surface before and after scale removal could be summarized as the appearance of the droplets, the increase in the number of distribution and the volume, droplets rolling and merging, freezing into ice, the emergence of frost crystals, the growth of frost layer. However, the condensation time of droplets on the non-scaled wing surface and the appearance of frost crystals were earlier than those on the wing surface with scales. And the water droplets were densely distributed on the non-scale surface, and the freezing time was earlier. For example, the frozen time difference between the water droplets on the two kinds of wing surfaces was about 598 s, the appearance time difference of the frost crystals was about 614 s. It could be determined by the light transmittance and colour change of the water droplets attached to the wing surface of Catocala electa Borkhason. And the water droplets on the wing surface with scales were approximately spherical.
4. Discussion
4.1 Effect of wing surface wettability on frosting
The chemical components of the wing surface of Catocala electa Borkhason were also analyzed by using the energy dispersive spectrometry (Oxford-instruments X-Max, UK), as shown in Figure . It could be seen from that the scales on the wing surface of Catocala electa Borkhauson were mainly composed of C, N and O elements. Additionally, the scale surface of the Catocala electa Borkhauson has proteins, lipids, chitin, and other materials with poor thermal conductivity [Citation49–52] and hydrophobicity, which can delay the freezing time of droplets attached to the scale surface.
The static contact angle was used to evaluate the wettability of the wing surface of Catocala electa Borkhauson before and after scale removal. The average contact angle of water droplets on the wing surface with scales was approximate 151 °, as shown in Figure . a. Meanwhile, the rolling angle of water on different wing surfaces was measured. The average rolling angle of water on the scale surface was 4.5 °, and the standard deviation was 0.58 °. The rolling angle of water on the wing surface without scales of Catocala electa Borkhason exceeds 60 °. During the measured process, it was difficult for the droplet to adhere to the wing surface with scales, and the only way to make the droplet fall on the wing surface was by shaking the pipette. Combined with the result, it showed that the scale surface had low adhesion characteristics. During the observation test, the condensed droplets rolled quickly on the surface of scales, the droplets were spherically and sparsely distributed on the wing surface, as shown in Figure (images c∼k). Combined with the contact angle of the droplet on the wing surface with scales, the wing surface of Catocala electa Borkhauson showed low adhesion and superhydrophobicity.
Figure 8. The contact angle of water droplets on the specimens. (a) contact angle on the wing surface with scales; (b) contact angle on the wing surface without scales.
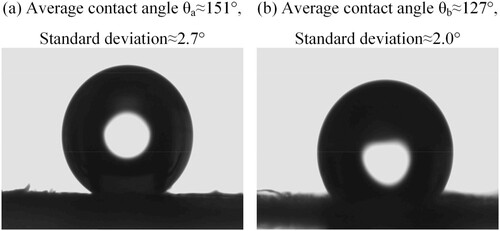
Compared with the contact angle of the wing surface with scales, the contact angle of the wing surface was significantly reduced after removing the scales, and the contact radius between the water droplet and wing surface was increased, as shown in Figure . b. The contact radius of droplets on the wing surface without scales was approximate 1.49 times that of the scaled wing surface. Combined with the observation test, the droplets on the wing surface without scales were not easy to roll and fall off. It could be seen that after the wing surface was processed to remove the scales, the contact area between the droplets and the wing surface was increased, and the rolling speed of the droplets was reduced. It increased the number of water droplets distributed on the wing surface without scales and made droplets easier to adhere to and freeze. It could be seen from Figures and .
4.2 Effect of wing surface morphology on frosting
A stereo microscope (Zeiss Stemi 2000-C, Oberkochen, Germany) was used to observe the microscopic morphology of the wing surface of Catocala electa Borkhauson before and after the scales were removed, as shown in Figure (images a and b). The tips of scales were serrated, and the scales were distributed on the wing surface in a tiled form. And the microscopic structure of the scale was scanned by atomic force microscope (ICON2-SYS, Bruker Nano Inc., USA). It was found that the scale surface had a staggered morphology of transverse ribs with similar spacing and orderly distribution of longitudinal ribs, as shown in Figure . c. The scale surface formed many micro-nano spaces that could hold air.
Figure 9. The microstructure of the wing surface of Catocala electa Borkhauson. (a) observed the wing surface without scales by Zeiss Stemi 2000-C; (b) observed the wing surface with scales by Zeiss Stemi 2000-C; (c) microscopic structure of the scale by atomic force microscope.
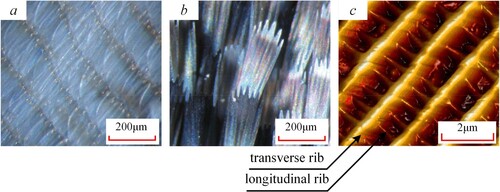
Moreover, the morphological feature of the wing of the Catocala electa Borkhason was observed by scanning electron microscopy (XL-30 ESEM FEG Scanning Electron Microscope FEI Company, USA), and the figure has been added in the revised manuscript, as shown in Figure . As could be seen from the observed Figure and 9. c, the scales on the wing surface of Catocala electa Borkhason had a criss-cross structure, forming a special micro-nano space. The longitudinal and transverse ribs were orderly distributed on the scale surface. The spacing of the transverse ribs was approximate 2 μm, and the spacing of longitudinal ribs between transverse ribs was different. According to Figure b, there were many nipples under the scale surface, so that the scale could not fit on the wing surface. It could also delay the condensation of water droplets on the scale surface.
Figure 10. Scanning electron microscopy image of the wing of the Catocala electa Borkhason. (a) wing with scales; (b) wing without scales.
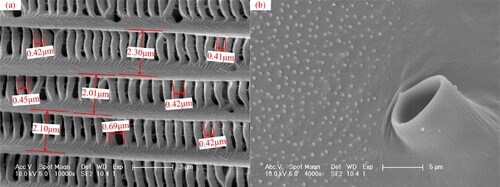
Researchers have found that the wing surface scales of Catocala electa Borkhauson are composed of low thermal conductivity materials such as chitin [Citation49–52], and the criss-cross microstructures on the surface made the wing surface of Catocala electa Borkhauson form a micro-nano non-smooth morphology. It could significantly delay the formation of water droplets on the wing surface. When the surface temperature of the scales of Catocala electa Borkhauson could reach the dew point temperature, the small water droplets were completely immersed in the criss-cross microstructure of the scale surface, which conformed to the Wenzel model.
In short, the water droplets were attached in a spherical shape on the scale surface with superhydrophobic property, reducing the contact area and decreasing the heat transfer rate. The cooling time of water droplets on the wing surface with scales was longer than that on the surface without scales, which could effectively prolong the freezing time of water drops and the appearance of frost branches on the frozen droplets. This could also be seen from the frost observation test results. Additionally, the air stored in the structure prevented the droplet from completely inflating the non-smooth morphology on the scale surface. This would also help to delay the formation of frost on the scale surface of Catocala electa Borkhauson.
5. Conclusions
The study investigated the influence of the scales of the Catocala electa Borkhauson on the frosting process. The experiments showed that the frost on the wing surface before and after the scale removal had undergone a similar formation process. It could be summarized as the formation of water droplets- the increase in distribution and volume-rolling, merging, and freezing of water droplets- the appearance of frost crystals-frost layer growth. However, the scales on the wing surface could affect the attachment shape and distribution number of the droplets, and the freezing of water droplets and the appearance of frost crystals were delayed. And the hydrophobicity of the wing surface would be reduced by removing the scales. The microscopic observation test revealed many criss-cross structures on the scale surface, which formed the micro-nano space for air storage on the scale surface, and the water droplets were in composite contact on the surface. The special micro-nano structure could delay the freezing of water on the scale surface. Additionally, the scales on the wing surface of Catocala electa Borkhauson were superhydrophobic, and the water droplets were easier to roll and fall off, so the contact time between the droplets and the scale surface was reduced. The freezing time of the droplets was also delayed. Combining with previous studies on the composition of the wing scale of Catocala electa Borkhauson, the present stud could help provide a reference for designing a coupled functional surface in the field of anti-icing/frosting.
Author contributions
The first author, Ren, Cong, and Jin, carried out the Catocala electa Borkhauson lab work, participated in data analysis, conceived of the study, designed the study; the first author, Chen, Xu, and Jin participated in the design of the study and drafted the manuscript, carried out the statistical analyses and collected field data; Chen Cong, Jin, and Choy critically revised the manuscript, coordinated the study and helped draft the manuscript. All authors gave final approval for publication and agreed to be held accountable for the work performed therein.
Disclosure statement
No potential conflict of interest was reported by the author(s).
Additional information
Funding
References
- Da Silva DL, Melo C, Hermes CJL. Effect of frost morphology on the thermal-hydraulic performance of fan-supplied tube-fin evaporators. Appl Therm Eng. 2017;111:1060–1068.
- Joppolo CM, Molinaroli L, De Antonellis S, et al. Experimental analysis of frost formation with the presence of an electric field on fin and tube evaporator. Int J Refrig. 2012;35(2):468–474.
- Pu L, Liu R, Huang H, et al. Experimental study of cyclic frosting and defrosting on microchannel heat exchangers with different coatings. Energy Build. 2020;226:Article ID 110382.
- Song MJ, Deng SM, Dang CB, et al. Review on improvement for air source heat pump units during frosting and defrosting. Appl Energy. 2018;211:1150–1170.
- Jeong CH, Lee SH. Condensation frosting characteristics of SAM-coated nanostructured superhydrophobic surface. Int. J. Air-Cond. Refrig. 2018;26(1):Article ID 1850008.
- Lynch FT, Khodadoust A. Effects of ice accretions on aircraft aerodynamics. Prog Aerosp Sci. 2001;37(8):669–767.
- Hu WJ, Song MJ, Jiang YQ, et al. A modeling study on the heat storage and release characteristics of a phase change material based double-spiral coiled heat exchanger in an air source heat pump for defrosting. Appl Energy. 2019;236:877–892.
- Song MJ, Fan C, Mao N, et al. An experimental study on time-based start defrosting control strategy optimization for an air source heat pump unit with frost evenly distributed and melted frost locally drained. Energy Build. 2018;178:36–37.
- Yoon Y, Jeong H, Lee KS. Adaptive defrost methods for improving defrosting efficiency of household refrigerator. Energy Convers Manage. 2018;157:511–516.
- Zhu JH, Sun YY, Wang W, et al. A novel temperature-humidity-time defrosting control method based on a frosting map for air-source heat pumps. Int J Refrig. 2015;54:45–54.
- Latthe SS, Sutrar RS, Bhosale AK, et al. Recent developments in air-trapped superhydrophobic and liquid-infused slippery surfaces for anti-icing application. Prog Org Coat. 2019;137:Article ID 105373.
- Fakorede O, Feger Z, Ibrahim H, et al. Ice protection systems for wind turbines in cold climate: characteristics, comparisons and analysis. Renew Sust Energ Rev. 2016;65:662–675.
- Amer M, Wang CC. Review of defrosting methods. Renew Sust Energ Rev. 2017;73:53–74.
- Sonobe N, Fukiba K, Sato S, et al. Method for defrosting heat exchangers using an air-particle jet. Int J Refrig. 2015;60:261–269.
- Sheng W, Liu PP, Dang CB, et al. Review of restraint frost method on cold surface. Renew Sust Energ Rev. 2017;79:806–813.
- Wang F, Liang CH, Zhang XS. Research of anti-frosting technology in refrigeration and air conditioning fields: A review. Renew Sust Energ Rev. 2018;81:707–722.
- Hayashi Y, Aoki A, Yuhana H. Study of frost properties correlation with frost formation types. J Heat Transfer. 1977;6(3):79–94.
- Tao YX, Besant RW, Rezkallah KS. A mathematical model for predicting the densification and growth of frost on a flat plate. Int J Heat Mass Transf. 1993;36(2):353–363.
- Na B, Webb RL. A fundamental understanding of factors affecting frost nucleation. Int J Heat Mass Transf. 2003;46(20):3797–3808.
- Piucco RO, Hermes CJL, Melo C, et al. A study of frost nucleation on flat surfaces. Exp Therm Fluid Sci. 2008;32(8):1710–1715.
- Armengol JM, Salinas CT, Salinas CT, et al. Modeling of frost formation over parallel cold plates considering a two-dimensional growth rate. Int J Therm Sci. 2016;104:245–256.
- Gong JY, Hou JQ, Sun JJ, et al. A numerical investigation of frost growth on cold surfaces based on the lattice boltzmann method. Energies. 2018;11(8):Article ID 2077.
- Hermes CJL. An analytical solution to the problem of frost growth and densification on flat surfaces. Int J Heat Mass Transf. 2012;55(23-24):7346–7351.
- Sommers AD, Napora AC, Truster NL, et al. A semi-empirical correlation for predicting the frost density on hydrophilic and hydrophobic substrates. Int J Refrig. 2017;74:313–323.
- Wang W, Guo QC, Lu WP, et al. A generalized simple model for predicting frost growth on cold flat plate. Int J Refrig. 2012;35(2):475–486.
- Wu XM, Ma Q, Chu FQ, et al. Phase change mass transfer model for frost growth and densification. Int J Heat Mass Transf. 2016;96:11–19.
- Chen YP, Lu PF, Shen CS, et al. Experimental study on frost formation on a cold surface in low atmospheric pressure. Appl Therm Eng. 2015;90:86–93.
- Gong JY, Sun JJ, Li GJ. An experimental study of the effect of air quality on frosting on cold flat surface. Int Commun Heat Mass Transfer. 2017;82:139–144.
- Li LY, Liu ZL, Li YX, et al. Frost deposition on a horizontal cryogenic surface in free convection. Int J Heat Mass Transf. 2017;113:166–175.
- Tahavvor AR, Yaghoubi M. Experimental and numerical study of frost formation by natural convection over a cold horizontal circular cylinder. Int J Refrig. 2010;33(7):1444–1458.
- Esmeryan KD, Gyoshev SD, Castano CE, et al. Anti-frosting and defrosting performance of chemically modified super-nonwettable carbon soot coatings. J Phys D: Appl Phys. 2021;54(1):Article ID 015303.
- Laturkar SV, Mahanwar PA. Superhydrophobic coatings using nanomaterials for anti-frost applications - review. Nanosyst-Phys Chem M. 2016;7(4):650–656.
- Esmeryan KD, Castano CE, Gyoshev SD, et al. On the dynamics of contact line freezing of water droplets on superhydrophobic carbon soot coatings. Curr Appl Phys. 2021;31:74–86.
- Kulinich SA, Farhadi S, Nose K, et al. Superhydrophobic surfaces: are they really ice-repellent? Langmuir. 2011;27(1):25–29.
- Ozkan DB, Ozil E, Inan C. Experimental investigation of the defrosting process on domestic refrigerator finned tube evaporators. Heat Transfer Eng. 2012;33(6):548–557.
- Varanasi KK, Deng T, Smith JD, et al. Frost formation and ice adhesion on superhydrophobic surfaces. Appl Phys Lett. 2010;97(23):Article ID 234102.
- Wexler JS, Grosskopf A, Chow M, et al. Robust liquid-infused surfaces through patterned wettability. Soft Matter. 2015;11(25):5023–5029.
- Zheng SL, Cheng L, Fu Q, et al. Development of stable superhydrophobic coatings on aluminum surface for corrosion–resistant, self–cleaning, and anti–icing applications. Mater Des. 2016;93:261–270.
- Zheng HK, Chang SN, Zhao YY. Anti-icing & icephobic mechanism and applications of superhydrophobic/ultra slippery surface. Prog Chem. 2017;29(1):102–118.
- Yuan ZQ, Chen H, Tang JX, et al. A novel preparation of polystyrene film with a superhydrophobic surface using a template method. J Phys D: Appl Phys. 2007;40(11):3485–3489.
- Wang ST, Liu KS, Yao X, et al. Bioinspired surfaces with superwettability: new insight on theory, design, and applications. Chem Rev 2015;115(16):8230–8293.
- Zang DM, Zhu RW, Zhang W, et al. Corrosion-resistant superhydrophobic coatings on mg alloy surfaces inspired by lotus seedpod. Adv. Funct. Mater. 2017;27(8):Article ID 1605446.
- Gao XF, Yan X, Yao X, et al. The dry-style antifogging properties of mosquito compound eyes and artificial analogues prepared by soft lithography. Adv Mater. 2007;19(17):2213–2217.
- Lee SG, Lim HS, Lee DY, et al. Tunable anisotropic wettability of rice leaf-like wavy surfaces. Adv Funct Mater. 2013;23(5):547–553.
- Liu CC, Ju J, Zheng YM, et al. Asymmetric ratchet effect for directional transport of fog drops on static and dynamic butterfly wings. Acs Nano. 2014;8(2):1321–1329.
- Li Q, Guo ZG. Fundamentals of icing and common strategies for designing biomimetic anti-icing surfaces. J Mater Chem A. 2018;6(28):13549–13581.
- Chen SC. Pictorial handbook of rare and precious insects in China. Beijing: China Forestry Publishing Press, China, 1998; pp. 196–198.
- Institute of Zoology, Chinese Academy of Sciences. Iconocraphia heterocerorum sinicorum. Beijing: Science Press, China, 1982; Pp. 237–387.
- Chen TK, Cong Q, Qi YC, et al. Hydrophobic durability characteristics of butterfly wing surface after freezing cycles towards the design of nature inspired anti-icing surfaces. Plos One. 2018;13(1):Article ID: 0188775.
- Steve H. The wing of a butterfly. Global Cosmetic Industry. 2002;170(8):32–35.
- Schroder-Turk GE, Wickham S, Averdunk H, et al. The chiral structure of porous chitin within the wing-scales of callophrys rubi. J Struct Biol 2011;174(2):290–295.
- Wang XJ, Cong Q, Zhang JJ, et al. Multivariate coupling mechanism of NOCTUIDAE moth wings’ surface superhydrophobicity. Chin Sci Bull. 2009;54(4):569–575.