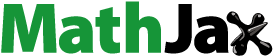
Abstract
The co-precipitation route was used for the preparation of Nd-substituted NiFe2O4. The proposed general formula of prepared ferrite was NdxNiFe2-xO4. Here, “x” the moles of Nd3+ were 0.0, 0.0125, 0.025 and 0.05 in various compositions. The prepared nanocrystallites were characterized using X-rays diffraction (XRD), Fourier transform infrared spectroscopy (FTIR), vibrating sample magnetometer (VSM) and impedance analyzer. XRD patterns of all prepared samples were analyzed to confirm the phase of the materials and determine the lattice constant, crystallite size and X-rays density. The lattice parameter value varied between 8.3501 and 8.3549 Å. The average crystallite size was ∼15 nm. The FTIR spectral analysis was used to investigate the M-O stretching vibrational bands. FTIR spectra showed the stretching vibrational bands of Fe-O at octahedral and tetrahedral sites. After structural and spectral investigations, the prepared nanocrystallites were subjected to room temperature magnetic measurements by vibrating sample magnetometry (VSM) technique. The magnetic parameters were investigated from the VSM data. The magnetic parameters suggested the possible utilization of these nanocrystalline ferrites for various advanced technological applications like switching devices and high-frequency devices. The dielectric parameters were also investigated using an impedance analyzer.
1. Introduction
Nanostructural and magnetic features of ferrites play an essential role in the technology industry due to their soft nature and sensitivity to sintering temperature. Nanocrystalline materials, including ferrites having magnetic nature, can absorb microwaves due to strong microwave absorption capability [Citation1,Citation2]. Ferrites have attracted the significant attention of materials scientists because of their versatile applications. For example, the ferrites have been reported in catalysis, ferrofluids, therapeutic visualization, sensors, magnetic data bank and anode material in batteries [Citation3–6]. These materials with high electrical resistivity and low eddy current losses are essential for high-frequency devices fabrication [Citation7,Citation8]. Such nanosized magnetic materials possess unique physical, magnetic and electrical properties due to the high surface volume ratio of grains. These ferrites are being used in high-frequency transformers cores and radiofrequency coils [Citation9]. Nickel ferrites are extensively used in the electronic industry because they have large permeability and high electrical resistivity in the high-frequency region. Nickel ferrites have an inverse structure with the replacement of Ni2+ metal cation at the octahedral sites. The properties of the materials at room temperature are highly dependent on the synthesis procedure, sintering temperature, atmosphere and nature of substituted metal cations [Citation10]. The calculated spin moment of Fe2+/3+ ions at A-site is 3.36 μB and the average moment at B-site is 2.45 μB, which showed that the net spin moment is oriented toward A-site direction. The introduction of rare earth metal cations into NiFe2O4 nanocrystallites has interesting conclusions about structural, electrical and magnetic properties. If the 3d-4f spin coupling is changed, it will alter the magnetic properties. Thus, the magnetic properties in inverse spinel ferrites are being controlled by Re–Fe interactions [Citation11].
Akhtar et al. studied the substitution of various Re3+ metal cations on spinal NMZ nano ferrites using a sol–gel synthesis procedure. A single-phase cubic structure was observed in all samples with no impurity. Pr3+-substituted NMZ nano-ferrites were observed more porous than other samples, while Gd-substituted NMZ nano-ferrites have higher saturation magnetization and absorptivity compared with other samples [Citation12]. Akhter et al. prepared Er3+-substituted nickel–zinc ferrites using the wet chemical procedure and studied the structural, spectral, electrical and magnetic parameters in detail. The cubic phase structure was observed. The appearance of the second phase showed an effect on dielectric and saturation magnetization properties. The decrease in saturation magnetization was due to the second phase [Citation13]. Baig et al. studied the structural and electrical properties of La3+ Substituted MnFe2O4 ferrite nanocrystallites. They used a low-cost effective reverse micelles microemulsion procedure. The lattice constant decreased with an increase in Re3+ metal cation substitution [Citation14].
Various synthesis methods were applied to prepare the nanocrystallites like co-precipitation [Citation13], micro-emulsion [Citation14] and sol–gel [Citation15,Citation16]. However, we preferred the co-precipitation method for the synthesis of nanocrystalline spinel ferrites (NdxNiFe2-xO4). The co-precipitation method is one of the most common facile routes for ferrites synthesis [Citation17]. The properties of ferrites are greatly affected by the dispersion of cations in the crystalline structure [Citation18]. The degree of substitution changes the properties of prepared materials for several applications like magnetic resonance visualization (MRI), transformer applications, telecommunications, multilayer chip inductors, and catalytic activities [Citation19]. Here, in this article, we have fabricated the Nd3+-substituted NiFe2O4 nanocrystallites spinel ferrites via the co-precipitation method. The primary motivation was to improve the structure of materials and to enhance magnetic and dielectric behaviour.
2. Experimental detail
2.1. Chemicals used
Following chemicals were used for the synthesis of unsubstituted and Nd-substituted nanocrystalline nickel spinel ferrites. (i) Ferric nitrate nonahydrate, (Fe(NO3)3·9H2O), Sigma Aldrich 98%; (ii) neodyum nitrate (Nd(NO3)3·6H2O), Sigma Aldrich 99.9%; (iii) nickel chloride (NiCl2·6H2O), BDH Analar 98%; (iv) aqueous ammonia (NH3 (aq.)), Riedel-de-Hein 35% and (v) distilled water. All these chemicals were used as they received. No purification was done of these chemicals.
2.2. Synthesis procedure
The nanocrystallites of unsubstituted spinel nickel ferrite (NiFe2O4) and Nd3+-substituted nickel ferrites (“NiNdxFe2-xO4” ferrite were prepared using a simple co-precipitation method [Citation15,Citation20,Citation21]. Aqueous solutions of metal salts like Ni2+, Fe3+ and Nd3+ were freshly prepared as per concentration and volumes requirements. The concentrations of these metal ions were kept as Ni2+ (0.1 M), Fe3+ (0.2 M) and Nd3+ (0.2 M). The required stoichiometric amount of each salt was weighed and then poured into distilled water to prepare these solutions. The stirring was carried out with slight heating to ensure the complete solubility of metal salts in the distilled water for preparation of the homogeneous solution. Four separate beakers were taken, washed and dried. Into these four beakers, the required volumes of each salt solution (Ni2+, Fe3+ and Nd3+) was taken. These four beakers containing various reaction mixtures were kept on magnetic stirring. The pH for all solutions was increased to 10. The main purpose of increased pH was formation of corresponding metal hydroxides in the form of precipitates. The pH of all reaction mixture was increased using a 3 M freshly prepared aqueous ammonia (NH4OH). After, when pH has been reached at about 10, the reaction mixtures were stirred for 7 h at room temperature. All reaction mixtures of four various compositions of substituted and unsubstituted nickel spinel ferrites were placed on a bench overnight as static. The main purpose of placing the reaction mixtures as static was to settle maximum metal hydroxide precipitates. Next day, the all four reaction mixture precipitates were washed with distilled water. The washing was continued till the pH reached 7. The drying of all these precipitates with different compositions was carried out in the drying oven at about 80–100°C. All dried precipitates were ground with Agate Pestle Mortar. The finely powdered precipitates were annealed in a muffle furnace. The temperature of the furnace was kept at 550°C. The annealing was continued for 3 h. Schematically, the entire synthetic scheme is illustrated in Figure [Citation22].
2.3. Characterization techniques
The prepared unsubstituted and Nd3+ substituted nickel spinel ferrites were characterized by various advanced techniques. These techniques are (i) powder X-ray diffraction analysis, (ii) Fourier transform infra-red spectroscopy, (iii) vibrating sample magnetometry and (iv) impedance analyzer. The specifications of the instruments of these techniques are (i) X-ray diffractometer (Bruker D8 advance) (XRD), (ii) Tensor-27 spectrometer (FTIR), (iii) Impedance meter (RF model E4991A Agilent) (dielectric properties studies) and (iv) Lakeshore-74071 Vibrating Sample Magnetometer (magnetic properties studies). All these four techniques based characterizations were carried out at room temperature.
3. Results and discussion
3.1. X-ray diffraction analysis
The structural characterization of prepared unsubstituted and Nd3+-substituted spinel nickel ferrites was carried out by powder XRD. The room temperature XRD patterns were recorded in twotheta range 20–70°. All these patterns are shown in Figure (a). The major diffraction planes observed at hkl (111), (220), (311), (400), (422), (511) and (440). These planes were appeared at twotheta 30, 35, 43, 56, 58 and 64°. The patterns were matched with ICDD # 003-0875. This confirmed the development of face-centred cubic structure [Citation23]. The introduction of RE3+ metal cation exhibit excellent electrical and magnetic properties; however, these cations showed limited solubility with the appearance of unidentified secondary phase (NdFeO3), which is a common property of rare earth elements. As the concentration of Nd3+ increased, a higher ionic radius of ion creates a hindrance for entering into crystal lattice structure that produced distortion and strain in the structure. This effect on the lattice constant [Citation24]. Lattice constant increased with an increase in the concentration of substituent Nd3+ cations. However, the substituted nickel ferrite compositions with x = 0.05, lattice constant value decreased due to the appearance of secondary phase [Citation25,Citation26]. Various other structural and physical parameters were calculated from XRD data. All these parameters are presented in Table . The cell constant values were determined by Equation (1) [Citation25].
(1)
(1) Scherer’s formula
was applied for the calculation of crystallite sizes nanocrystallites. Obtained crystallite sizes were estimated from 14.21 to15.84 nm and presented in Table and Figure . As the concentration of RE3+ ions increased, the crystallite size increased. The X-ray density values of NiNdxFe2-xO4 nanocrystallites were determined by Equation (2).
(2)
(2) In this equation, M is molar mass, V is cell volume and NA stands for the Avogadro number. It has been observed that as the Nd3+ contents were increased, the X-rays density values were also increased. During the detailed analysis of XRD results, two main observations were observed. First, as the lattice constant increased, the crystallite size was also increased. Second, there is a slight increase in the lattice constant that showed the replacement of Fe3+ metal cation with Nd3+ metal cation on the octahedral sites. However, a slight decrease in the lattice constant was observed for compositions with x = 0.05. This decrease may be due to improper replacement of Nd3+ that also produced secondary phase. The appearance of the secondary phase in the sample was due to the competition between metal cations at the octahedral sites [Citation22,Citation27]. The substituent RE3+ ions have a markedly higher ionic radius than iron; therefore, it is evident that all RE3+ ions were placed at octahedral sites that increased the lattice constant and produced distortion in the structure [Citation28,Citation29].
Table 1. Various XRD parameters of Nd-substituted NiFe2O4 nanocrystallites.
3.2. Fourier transforms infrared spectroscopy
Fourier transforms infrared (FTIR) analysis was performed in the wavenumber range 400–1000 cm−1. The obtained room temperature FTIR spectra are shown Figure . The two prominent bands were suggested by Waldron in 1955 for metal oxides (spinel ferrites) within 400–700 cm−1. The high-frequency band (υ1) within 500–600 cm−1 confirmed the stretching vibration between M-O at the tetrahedral sites. The low-frequency band (υ2) confirmed the movement of the Fe3+-O2− band group at the octahedral sites between 500 and 400 cm−1 [Citation30–34]. The obtained spectral band values are also presented in Table . The increase in band stretching at both sites was observed due to an increase in the concentration of Nd3+ metal cations into the lattice structure [Citation35]. The absorption band between 400 and 700 cm−1 represents the intrinsic stretching of M-O ions between two sites. Intrinsic stretching in this range is the characteristic of all AB2O4 systems of normal and inverse spinel metal oxides. The absorption bands in the expected range between two sites resulted that substituted metal cations may be replaced with Fe3+ metal cations on the octahedral sites. The broadness of bands showed the increased concentration of Nd3+ metal cations [Citation13]. The different bond lengths of A-sites and B-sites were responsible for different band positions of M-O. The narrow range variation of band positions may represent the effect of the secondary phase that affected the bond length of the Fe-O complex at both sites [Citation36–39]. Bond lengths and force constants can be calculated from the following equations.
(3)
(3)
(4)
(4) where υ1 and υ2 are frequency bands and M is the molecular weight of spacemen [Citation40].
Table 2. FTIR modes (υ1 and υ2) and force constants (Ft and Fo) for Nd-substituted NiFe2O4 nanocrystallites.
3.3. Magnetic properties studies
Magnetic properties of unsubstituted and Nd3+-substituted nickel spinel nanocrystallites were determined at room temperature. These properties were studied by a vibrating sample magnetometer (VSM). The obtained M-H (magnetic-hysteresis) loops for all compositions of NiNdxFe2-xO4 nanocrystallites are shown in Figure . Shirsath et al. studied the structural and magnetic properties of In3+-substituted NiFe2O4 in detail. They reported that as the concentration of In3+ increased, the Ms value increased. This showed that substitution of In3+ metal cation on B-sites might have shifted the Ni2+ ions to A-sites. This shifting the metal cations from one site to other site may be responsible for increased magnetization [Citation41]. Another report regarding the effect of rare earth cations substitution on magnetic properties of nickel ferrites has been reported by Tahira et al. They studied the effect of Er3+ cations. They observed the mixed behaviour of saturation magnetization. First, the Ms value increased and then decreased due to increase in the concentration of Er3+ metal cation that increased the secondary phase formation. This formation of the secondary phase may produce a distorted structure. Magnetic moment (nB) was determined using Equation (5). This equation is based on the saturated magnetization as well as on the molecular weight of nanocrystallites [Citation42].
(5)
(5) From the obtained VSM data, various magnetic parameters were extracted and determined. These parameters included (i) saturation magnetization (Ms), (ii) retentivity (Mr), (iii) coercivity (Hc), (iv) squareness ratio (R), and (v) momentary magnetic fields (nB). The calculated values for all these components are presented in Table [Citation43]. Neel sublattice model of ferromagnetic material was used to study the magnetic properties and cation distribution between A and B sublattices. Among all models, the A-B superexchange model was more effective than A-A and B-B models [Citation34]. The magnetic moment was calculated using the difference of magnetic moments between A and b sublattice. M = MB–MA. The 3d coupling between negative Fe–Fe interaction raised the high magnetic structure of inverse spinel. The interaction of 3d-4f coupling and 5d-4f indirect coupling can easily break with the corruption of RE3+ metal cations. The decreased in saturation magnetization was because of the shorter ionic radius of Fe3+ ions compared with Nd3+ ions that increased the distance and caused the weakening of interaction. This decreased the magnetic moment and also decreased the saturation magnetization [Citation44,Citation45]. When Nd3+ cations were incorporated in the structure, the Ms values were decreased. This decrease was due to the occupation of a large ionic radius of Nd3+ metal cations on the octahedral sites. S-type M-H loops were observed that confirmed the soft magnetic behaviour of the prepared nanocrystalline ferrites. Further, the crystallite size less than 42 nm estimated the soft nature of the materials [Citation46,Citation47]. The addition of Nd3+ ions into the B sites created cationic stress among various cations. Within the solid Nd3 + lattice and different terrestrial terrain, because of the orbital screen impact electron in the 4f sub-shell, there will be a magnetic force due to its high lattice structure but exhibit very strong magnetic connections with the surrounding areas. It is due to the protection of electrons in 4f from the crystal field of electrons of 5d and 6s [Citation48]. The well-recognised shell model was also used to explain this decrease in a magnetic field. This version explained in more detail the existence of spins under nanocrystallites due to their small size, which keeps them discounted on magnetization. Many of the various factors further contributed to magnetic properties, such as lattice structure imperfections, ions settlement, preferred lattice residual location, surface impact, commercial interactions and surface morphology [Citation22,Citation23]. Such types of low magnetic materials can be used in high-frequency devices [Citation49].
Figure 5. Magnetic-Hysteresis curves of Nd-substituted NiFe2O4 nanocrystallites at room temperature.
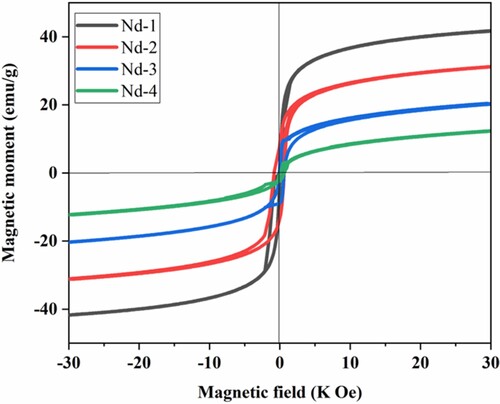
Table 3. Hysteresis parameters values derived from room temperature hysteresis loops for Nd-substituted NiFe2O4 nanocrystallites.
One more important parameter, anisotropy constant (K), can be calculated by the Stoner–Wohlfarth model using its Equation (6):
(6)
(6) Magnetic anisotropy depends on Hc and Ms values. The obtained K values for all compositions were found in the range 0.864 × 103 erg/g to 1.481 × 103 erg/g. These values are the level of ferromagnetic nano-component anisotropy [Citation50]. The strength of the magnetic material is to be rearranged in the nearest magnetic field after removing the magnetic field, and we can assess the values of Mr and square size (r = Mr/Ms) [Citation23]. By removing the magnetic field, the ability of magnetic material was checked by remnant magnetization and squareness ratio [Citation51]. Low R values suggested the formation of a single domain structure [Citation46].
3.4. Dielectric properties
The dielectric behaviour of materials was studied at room temperature with a frequency range of 1–3 MHz. The performance of the material illustrates the progress of electric dipole moments. This was due to the interchange of electrons between A and B within its structure. The real and imaginary parts (ε′ and ε′′) of all samples were calculated using the following equations and shown in Figure [Citation52]:
(7)
(7)
(8)
(8)
(9)
(9)
(10)
(10)
Figure 6. (a) Real part of dielectric permittivity, (b) imaginary part of dielectric permittivity, and (c) tan loss vs frequency (GHz) of NiNdxFe2-xO4 nanocrystallites.
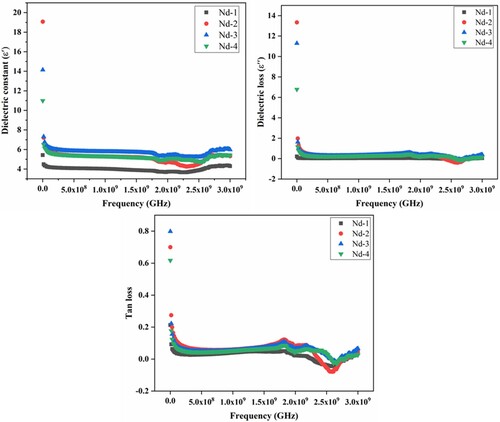
In these equations, the “C” represents the capacitance of parallel plate capacitor, “d” is the thickness, “A” shows the area of the surface of pellet, and ε0 presents space dielectric constant [Citation53]. There are four basic types of polarization mechanism present in dielectric materials. These are (i) ionic, (ii) dipolar, (iii) electronic, and (iv) space charge polarization. Among these four mechanisms, the dipolar polarization was exhibited and dominated in low-frequency region, and atomic, electronic polarization may exhibit in the higher frequency range [Citation28].
It was observed that with an increase in frequency, the dielectric constant decreased rapidly and then slowly decreased at a higher frequency level. Above 2 MHz frequency, dielectric constant was independent of frequency. This independence of frequency was due to the failure of electric dipoles with the applied field. Koop’s theory was useful to explain the decrease of dielectric constant with frequency [Citation54]. The structure of ferrites was composed of a large number of conducting grain boundaries visible at lower frequency regions. However, this showed poor conducting grain boundaries at higher frequency regions. This variation of conduction behaviour between low and high frequencies was explained by charge polarization phenomena [Citation55].
Figure (a) showed the real part (έ) measured from the 1 MHz to 3 GHz frequency range. It was observed that with an increase in Nd contents, the έ values increased. This increase in έ may be explained by polarization phenomena by strong preference movement of Fe3+ ions to A-sites. The Neel model is best to explain the hopping of electrons between Fe3+ and Fe2+ ions. An increase in dielectric polarization for electrons in the direction of the externally applied field is due to an increase in Fe ion concentration at B-sites, which increased the real part of permittivity έ [Citation56].
In spinel ferrites, the hopping of electron conductivity in iron cations occurred in the octahedral site. An increase in Fe2+ ions concentration increased the electron conductivity. The chemisorption of oxygen on the surface also oxidizes and reduces the Fe ions, which increased the Fe2+ concentration on B-sites and push back to Fe3+ ions to A-sites and replace the Ni ions. The electron transfer model was effectively used to elucidate this reason [Citation57]. The dielectric loss in spinel ferrites is shown in Figure (b) related to lag in polarization. This lag in polarization was studied with applied ac field that arises may be due to impurities, grain boundaries of the lattice structure. The dielectric loss was due to the transfer of a large amount of electrical energy into oscillating ions [Citation54]. The tangent loss factor of NiFe2-xNdxO4 nanocrystallites is the inverse relation to frequency (see Figure (c)).
4. Conclusion
The co-precipitation method was used to synthesize the NdxNiFe2-xO4 nanocrystallites. The co-precipitated precipitates of all compositions were annealed at 550°C for 3 h under air. The XRD structural analysis confirmed the cubic structure of spinel ferrites. The structure was further analyzed by FTIR that confirmed the stretching vibrational bands within 400–600 cm−1, which is the basic characteristic of spinel ferrites. Ms was found to decrease with an increased Nd3+ metal cations concentration. The dielectric parameters were affected by an increase in Nd3+ concentration. The observed magnetic and dielectric parameters of Nd3+-substituted nickel spinel ferrites showed that these materials may be used for fabrication of magnetic data storage devices and the devices that are required to operate at high frequencies.
Acknowledgements
The authors sincerely appreciate the King Saud University, Riyadh (Saudi Arabia) for sponsoring this work through researchers supporting project No. RSP-2021/163.
Disclosure statement
No potential conflict of interest was reported by the author(s).
References
- Liu X, Zhang T, Zhang L. Microwave-induced catalytic application of magnetically separable strontium ferrite in the degradation of organic dyes: insight into the catalytic mechanism. Sep Purif Technol. 2018;195:192–198.
- Munir S, Rasheed A, Zulfiqar S, et al. Synthesis, characterization and photocatalytic parameters investigation of a new CuFe2O4/Bi2O3 nanocomposite. Ceram Int. 2020;46:29182–29190.
- Tehrani-Bagha A, Gharagozlou M, Emami F. Catalytic wet peroxide oxidation of a reactive dye by magnetic copper ferrite nanoparticles. J Env Chem Eng. 2016;4:1530–1536.
- Fu Y, Chen Q, He M, et al. Copper ferrite-graphene hybrid: a multifunctional heteroarchitecture for photocatalysis and energy storage. Ind Eng Chem Res. 2012;51:11700–11709.
- Gomes J, Sousa M, Da Silva G, et al. Cation distribution in copper ferrite nanoparticles of ferrofluids: A synchrotron XRD and EXAFS investigation. J Magn Magn Mater. 2006;300:e213–e216.
- Nakhjavan B, Tahir MN, Panthöfer M, et al. Synthesis, characterization and functionalization of nearly mono-disperse copper ferrite CuxFe3−xO4 nanoparticles. J Mater Chem. 2011;21:6909–6915.
- Kannapiran N, Muthusamy A, Chitra P. Effect of MnCuFe2O4 content on magnetic and dielectric properties of poly (O-phenylenediamine)/MnCuFe2O4 nanocomposites. J Magn Magn Mater. 2016;401:345–353.
- Rao ADP, Ramesh B, Rao PRM, et al. Magnetic and microstructural properties of Sn/Nb substituted Mn–Zn ferrites. J Alloys Compd. 1999;282:268–273.
- Lakshmi M, Vijaya Kumar K, Thyagarajan K. An investigation of structural and magnetic properties of Cr–Zn ferrite nanoparticles prepared by a sol–gel process. J Nanostruct Chem. 2015;5:365–373.
- Bharathi KK, Markandeyulu G, Ramana CV. Structural, magnetic, electrical, and magnetoelectric properties of Sm- and Ho-substituted nickel ferrites. J Phys Chem C. 2011;115:554–560.
- Anwar A, Zulfiqar S, Yousuf MA, et al. Impact of rare earth Dy+3 cations on the various parameters of nanocrystalline nickel spinel ferrite. J Mater Res Technol. 2020;9:5313–5325.
- Akhtar MN, Yousaf M, Lu Y, et al. Highly efficient absorber with enhanced magnetoelectric properties based on Y, Gd, and Pr doped NMZ nanoferrites. J Magn Magn Mater. 2021;537:168232.
- Akhter T, Yousuf MA, Asghar M, et al. Structural, spectral, electrical and magnetic parameters evaluation of Er3+-substituted Ni08Zn02Fe2O4 nanoparticles synthesized by wet chemical route. Phys B. 2021;602:412574.
- Baig MM, Zulfiqar S, Yousuf MA, et al. Structural and photocatalytic properties of new rare earth La3+ substituted MnFe2O4 ferrite nanoparticles. Ceram Int. 2020;46(14):23208–23217.
- Baig MM, Yousuf MA, Agboola PO, et al. Optimization of different wet chemical routes and phase evolution studies of MnFe2O4 nanoparticles. Ceram Int. 2019;45:12682–12690.
- Ahmad M, Grössinger R, Kriegisch M, et al. Characterization of Sr-substituted W-type hexagonal ferrites synthesized by sol–gel autocombustion method. J Magn Magn Mater. 2013;332:137–145.
- Hou H, Xu G, Tan S, et al. A facile sol-gel strategy for the scalable synthesis of CuFe2O4 nanoparticles with enhanced infrared radiation property: influence of the synthesis conditions. Infrared Phys Technol. 2017;85:261–265.
- Aziz HS, Rasheed S, Khan RA, et al. Evaluation of electrical, dielectric and magnetic characteristics of Al–La doped nickel spinel ferrites. RSC Adv. 2016;6:6589–6597.
- Jauhar S, Kaur J, Goyal A, et al. Tuning the properties of cobalt ferrite: a road towards diverse applications. RSC Adv. 2016;6:97694–97719.
- Ejaz M, Mahmood A, Khan MA, et al. Influence of Yb3+ on the structural, dielectric and magnetic properties of Mg0.7Co0.3Fe2O4 nanocrystallites synthesized via co-precipitation route. J Magn Magn Mater. 2016;404:257–264.
- Ishaque M, Azhar Khan M, Ali I, et al. Study on the electromagnetic behavior evaluation of Y3+ doped cobalt nanocrystals synthesized via co-precipitation route. J Magn Magn Mater. 2014;372:68–73.
- Anwar A, Yousuf MA, Zulfiqar S, et al. The impact of highly paramagnetic Gd3+ cations on structural, spectral, magnetic and dielectric properties of spinel nickel ferrite nanoparticles. J Saudi Chem Soc. 2021;25:101306.
- Heiba ZK, Bakr Mohamed M, Arda L, et al. Cation distribution correlated with magnetic properties of nanocrystalline gadolinium substituted nickel ferrite. J Magn Magn Mater. 2015;391:195–202.
- Kumar H, Singh JP, Srivastava RC, et al. FTIR and electrical Study of dysprosium doped cobalt ferrite nanoparticles. J Nanosci. 2014;2014:862415.
- Yousuf MA, Baig MM, Al-Khalli NF, et al. The impact of yttrium cations (Y3+) on structural, spectral and dielectric properties of spinel manganese ferrite nanoparticles. Ceram Int. 2019;45:10936–10942.
- Pant RP, Arora M, Kaur B, et al. Finite size effect on Gd3+ doped CoGdxFe2−xO4 (0.0≤x≤0.5) particles. J Magn Magn Mater. 2010;322:3688–3691.
- Gadkari A, Shinde T, Vasambekar P. Structural analysis of Y3+-doped Mg–Cd ferrites prepared by oxalate co-precipitation method. Mater Chem Phys. 2009;114:505–510.
- Baig MM, Yousuf MA, Warsi MF, et al. Surfactant assisted synthesis of rare earth Dy3+ substituted MnFe2O4 nanoparticles. Ceram Int. 2019;45:18014–18022.
- Kurmude DV, Barkule RS, Raut AV, et al. X-ray diffraction and cation distribution studies in zinc-substituted nickel ferrite nanoparticles. J Supercond Novel Magn. 2014;27:547–553.
- Gunjakar JL, More AM, Gurav KV, et al. Chemical synthesis of spinel nickel ferrite (NiFe2O4) nano-sheets. Appl Surf Sci. 2008;254:5844–5848.
- Gadkari A, Shinde T, Vasambekar P. Influence of rare-earth ions on structural and magnetic properties of CdFe2O4 ferrites. Rare Met. 2010;29:168–173.
- Gilani ZA, Shifa MS, ul Huda Khan Asghar HMN, et al. New LiCo0.5PrxFe2−xO4 nanoferrites: prepared via low cost technique for high density storage application. Ceram Int. 2018;44:1881–1885.
- Ali R, Mahmood A, Khan MA, et al. Impacts of Ni–Co substitution on the structural, magnetic and dielectric properties of magnesium nano-ferrites fabricated by micro-emulsion method. J Alloys Compd. 2014;584:363–368.
- Ali R, Khan MA, Manzoor A, et al. Investigation of structural and magnetic properties of Zr-Co doped nickel ferrite nanomaterials. J Magn Magn Mater. 2017;429:142–147.
- Shaikh PA, Kambale RC, Rao AV, et al. Structural, magnetic and electrical properties of Co–Ni–Mn ferrites synthesized by co-precipitation method. J Alloys Compd. 2010;492:590–596.
- Prasad SAV, Deepty M, Ramesh PN, et al. Synthesis of MFe2O4 (M = Mg2+, Zn2+, Mn2+) spinel ferrites and their structural, elastic and electron magnetic resonance properties. Ceram Int. 2018;44:10517–10524.
- Josyulu OS, Sobhanadri J. The far-infrared spectra of some mixed cobalt zinc and magnesium zinc ferrite. Phys Stat Sol. 1981;65:479–483.
- Waldron RD. Infrared spectra of ferrites. Phys Rev. 1955;99(6):1727–1735.
- Asif Yousuf M, Mahmood Baig M, Shakir I, et al. The investigation of structural and magnetic properties of MnFe2-xWxO4 nanoparticles. Results Phys. 2020;19:103365(1)–103365(7).
- Manzoor A, Khan MA, Khan MY, et al. Tuning magnetic and high frequency dielectric behavior in Li-Zn ferrites by Ho doping. Ceram Int. 2018;44:6321–6329.
- Shirsath SE, Toksha BG, Jadhav KM. Structural and magnetic properties of In3+ substituted NiFe2O4. Mater Chem Phys. 2009;117:163–168.
- Shaikh PA, Kambale RC, Rao AV, et al. Effect of Ni doping on structural and magnetic properties of Co1–xNixFe1.9Mn0.1O4. J Magn Magn Mater. 2010;322:718–726.
- Junaid M, Khan MA, Iqbal F, et al. Structural, spectral, dielectric and magnetic properties of Tb–Dy doped Li-Ni nano-ferrites synthesized via micro-emulsion route. J Magn Magn Mater. 2016;419:338–344.
- Ansari MMN, Khan S, Ahmad N. Effect of R3+ (R = Pr, Nd, Eu and Gd) substitution on the structural, electrical, magnetic and optical properties of Mn-ferrite nanoparticles. J Magn Magn Mater. 2018;465:81–87.
- Joshi S, Kumar M, Chhoker S, et al. Structural, magnetic, dielectric and optical properties of nickel ferrite nanoparticles synthesized by co-precipitation method. J Mol Struct. 2014;1076:55–62.
- Yousuf MA, Jabeen S, Shahi MN, et al. Magnetic and electrical properties of yttrium substituted manganese ferrite nanoparticles prepared via micro-emulsion route. Results Phys. 2020;16:102973.
- Sharma US, Sharma RN, Shah R. Physical and magnetic properties of manganese ferrite nanoparticles. Int J Eng Res Appl. 2014;4(8):14–17.
- Karimi Z, Mohammadifar Y, Shokrollahi H, et al. Magnetic and structural properties of nano sized Dy-doped cobalt ferrite synthesized by co-precipitation. J Magn Magn Mater. 2014;361:150–156.
- Kambale RC, Song KM, Koo YS, et al. Low temperature synthesis of nanocrystalline Dy3+ doped cobalt ferrite: structural and magnetic properties. J Appl Phys. 2011;110:053910.
- Murugesan C, Chandrasekaran G. Impact of Gd3+ substitution on the structural, magnetic and electrical properties of cobalt ferrite nanoparticles. RSC Adv. 2015;5:73714–73725.
- Joshi S, Kumar M, Pandey H, et al. Structural, magnetic and dielectric properties of Gd3+ substituted NiFe2O4 nanoparticles. J Alloys Compd. 2018;768:287–297.
- Yousuf MA, Baig MM, Waseem M, et al. Low cost micro-emulsion route synthesis of Cr-substituted MnFe2O4 nanoparticles. Ceram Int. 2019;45:22316–22323.
- Anwar A, Yousuf MA, Tahir B, et al. New Er3+-substituted NiFe2O4 nanoparticles and their nano-heterostructures with graphene for visible light driven photo-catalysis and other potential applications. Curr Nanosci. 2019;15:1–12.
- Yadav RS, Kuřitka I, Vilcakova J, et al. Impact of grain size and structural changes on magnetic, dielectric, electrical, impedance and modulus spectroscopic characteristics of CoFe2O4 nanoparticles synthesized by honey mediated sol-gel. Adv Nat Sci: Nanosci Nanotechnol. 2017;8:045002.
- Kakade SG, Ma Y-R, Devan RS, et al. Dielectric, complex impedance, and electrical transport properties of erbium (Er3+) Ion-substituted nanocrystalline, cobalt-rich ferrite (Co1.1Fe1.9–xErxO4). J Phys Chem C. 2016;120:5682–5693.
- Zhong H, Wu Zhang H, Tao Zhou H, et al. Effects of WO3 substitution on electromagnetic properties of NiCuZn ferrite. J Magn Magn Mater. 2006;300:445–450.
- Reddy PV, Rao TS. Dielectric behaviour of mixed Mn-Mg ferrites at low frequencies. J Less Common Metals. 1985;105:63–68.