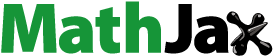
Abstract
In this paper, wet-chemically synthesized hierarchical NiO microspheres and their exfoliated (ExC)-based nanocomposites (NiO/ExC) as electrodes for supercapacitor applications have been reported. X-ray diffraction (XRD), Fourier transform infrared (FTIR) spectroscopy and Scanning electron microscopic (SEM) techniques were used to characterize the as-synthesized products. The study of PXRD revealed that NiO exhibits a cubic phase. Morphological analysis showed a hierarchical micro-spherical structure of mesoporous NiO particles. The as-synthesized hierarchically porous NiO manifested a specific capacitance of 331 F/g with 50% capacitance retention. But, exfoliated carbon (ExC)-based nanocomposite (0.07 g NiO/0.003 g ExC) showed a particular capacitance of 815 F/g at the scan rate of 5 mV/s, with 79% retention in capacitance. High electrical conductivity and larger surface area arising from synergistic effects between NiO and exfoliated carbon resulted in a tremendous electrochemical response of NiO/ExC nanocomposites. The inclusion of the ExC further improves electrical impedance spectroscopic results by facilitating the charge transfer.
GRAPHICAL ABSTRACT
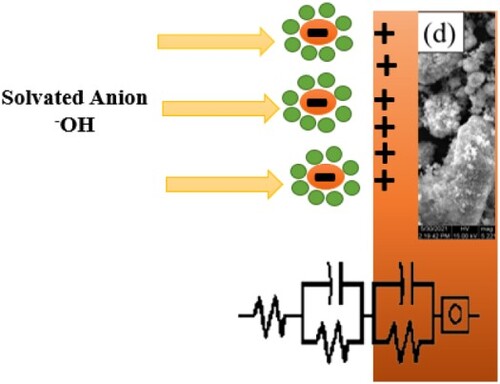
1. Introduction
The use of energy is rapidly increasing, and sources of energy are insufficient to meet energy demands. In the present age, fossil fuels are the source on which we depend mainly on energy requirements. Because of the decrease in the availability of fossil fuels and hazardous environmental impact, the demand for energy storage sources to fulfil the needs of energy is increasing intensely [Citation1]. To maintain the balance between energy demand and consumption, researchers are striving to design efficient and cost-effective energy storage devices that are environment-friendly. Generally, for energy storage and consumption purpose, solar cells, capacitors, supercapacitors, lithium-ion batteries, and fuel cells are used [Citation2]. Although lithium-ion batteries have many drawbacks, they are the most commonly utilized energy storage and consumption devices. On the other side, supercapacitors are considered very efficient due to their tuneable energy density, excellent periodic efficacy, high power density and longer life cycle [Citation3]. The supercapacitors are also termed electrochemical capacitors. These have higher power density than lithium-ion batteries and have better energy density than traditional capacitors [Citation4].
Supercapacitors are mainly categorized based on their charging capacities, named hybrid capacitors, pseudocapacitors (PSCs) and double-layer capacitors (EDLCs). The PSCs proceed with a redox reaction across the electrolyte and electrode, where EDLCs store charges across the electrical double layer by using an electrostatic approach [Citation5]. At the same time, both electrostatic and redox reaction takes place in hybrid supercapacitors [Citation6]. Among the three categories of supercapacitors, hybrid supercapacitors are capacitors with both high capacitance and a high energy storage capacity. They’ve received a lot of attention because of their proclivity for merging the properties of their constituents (EDLC and pseudocapacitors) [Citation7]. The possibilities for such combinations are vast, with a particular interest in those produced by conducting and electroactive components and aimed at energy storage [Citation8]. Many metal oxides, such as tin oxide, ruthenium oxide, ferrous oxide, manganese oxide, and cobalt oxide, have been researched to evaluate the electrochemical performance of the supercapacitors [Citation9]. Recently, Nickel oxide (NiO) has gained massive attention due to its unique optical, mechanical, electrochemical, and electronic properties [Citation10]. The use of NiO as an electrode, electrochromic device and catalyst is increasing day by day [Citation11]
The bandgap for the stable NiO p-type semiconductor ranges from 3.6 to 4.0 eV [Citation12]. Its properties include large spin optical stability, various manufacturing possibilities, excellent durability, electrochemical stability, and anodic electro-chromism. NiO can be combined with other components such as metal oxides, graphene, and CNT to increase the conductivity of the overall design as NiO tend to have low conductivity practically [Citation13].
NiO has been synthesized in several morphological structures employing various sysnthesizing techniques, such as nanotubes, nanoballs, nanosheets, nanoflakes, nanoflowers, nanospheres, nanorods and nanowires. The applications of semiconductor metal oxide rely on synthesis and properties such as size, morphology and structure. To manufacture NiO, several methods have been established, such as sol–gel synthesis [Citation14], wet chemical synthesis [Citation15], thermal decomposition method [Citation16], microwave method [Citation17] and hydrothermal method [Citation18]. Alhough these methods are easy to synthesizing nanocrystalline NiO,there are manyother methods, such as the solvothermal method [Citation19], template method [Citation20], electrode-deposition method [Citation21], reduction method [Citation22], and anodic arc plasma method [Citation23]. Many researchers have reported several morphological architectures of NiO used in supercapacitors. Liu and Anderson, synthesized porous NiO with a specific capacitance of 50–64 F/g in 1996 [Citation24]. Wang et al. used the immersion technique for the formation of wave-like NiO flakes to carry out the cyclic voltammetry and discharge process at different scan rates. Estimated r628 and 586 F/g capacitance were obtained at the scanning rates of 1 and 2 mV/s, respectively. Capacitance values achieved by NiO synthesized by microwave and reflux method were 598, 576, 541 F/g 388, 313 and 247 F/g, respectively, at the same scan rate mentioned above. NiO shows specific capacitance with a longer discharge time of 420, 399, 372, and 337 F/g at scan rates of 0.5, 1.0, 2.0, and 4.0 mV/s, respectively. Xing et al. [Citation25] observed the properties of mesoporous nano ball NiO using precursors NiCl2.6H2O and sodium dodecyl sulphate as a template and adopted a template method for its synthesis. The samples 250, 300 and 350°C achieved capacitances of 124, 106 and 68 Fg−1, respectively. Zhang et al. [Citation26] prepared nanocrystalline NiO by applying the microwave method and solid-state decomposition to achieve a capacitance of 97 and 186 F/g. Xu et al. [Citation26] utilized a thin nanosheet to synthesize NiO nanotube, whose resultant capacitance was 960 F/g. Ding et al. prepared NiO nanosheet hollow spheres with a capacitance of 415 F/g [Citation27].
Researchers have shown that using highly conductive substrates such asd exfoliated porous carbon (ExC) [Citation28], r-GO [Citation29], CNTs [Citation30] and exfoliated graphene (EG) [Citation31], the ability of the electrode material can be enhanced. Due to its porous framework, large surface area and unique structure of its exfoliated layers, ExC shows great capacitance compared to other materials. The specific capacitance of the NiO nanotubes can be increased by increasing the surface area. Jin et al. synthesized nitrogen-doped porous exfoliated carbon nanosheets in the basal plane, and phosphorus was present at lattice edges. The resultant optimal supercapacitor depicted a quite high specific capacitance of 265 F/g at 0.5 Ag−1. By preparing nanohybrids with an appropriate concentration of exfoliated carbon (ExC) [Citation32], it is possible to enhance the poor electrical conductivity. Soned et al. analyzed the electrochemical behaviour of synthesized exfoliated carbon fibres in H2SO4 electrolyte. The estimated specific capacitance of this sample was 450 F/g. Wang et al. estimated the capacitance in 130–165 F/g by synthesizing partially exfoliated carbon nanotubes. The results show that its resultant capacitance was greater than simple nanotubes. Toyoda et al. studied the capacitance using fibres of exfoliated carbon as electrode material for electric double-layer capacitance in 1 mol/dm3 H2SO4 as an electrolyte, where capacitance can be increased using concentrated sulphuric acid [Citation33]. Hence, by changing the morphology of the electrode with the highest degree of porosity and then mixing it with most conducting exfoliated carbon, the specific capacitance of the NiO electrode can be improved.
The main aim of the research was achieved by preparing porous NiO and their hybrids with ExC hierarchically by the sonication method. The resultant product achieved was analyzed by all required physicochemical techniques. Admirably, exfoliated carbon-based nanohybrid exihibited the highest specific capacity of 815 F/g at a scan rate of 5 mV/s. Because the exceptional performance of the exfoliated carbon-based nanocomposites made them prospective applicants for applications in high-performance supercapacitors.
2. Experimental section
2.1. Chemical reagents
Hierarchical porous NiO and its nanocomposites were synthesized utilizing the following precursor materials: Nickel chloride (NiCl2.6H2O); potassium hydroxide (KOH); sulphuric acid (H2SO4, 98%); phosphoric acid (H3PO4, 70%); graphite powder (C); ammonia solution (NH3.H2O) bought from Sigma Aldrich. All reactant materials used were of analytical grade.
2.2. Synthesis of hierarchically porous NiO
In this synthesis procedure, prepared 0.4 M nickel chloride hexahydrate (6 mL) and 0.48 M potassium hydroxide (2 mL) were mixed in a beaker under consistent stirring with the subsequent addition of 30 mL of deionized water and 1.8 mL of ammonia. In a water bath, the consequent homogenized dark blue solution was kept and incubated at 68–70°C for 24 h. After the incubation period, via centrifugation, the obtained black precipitates were washed until pH = 7 was achieved. Afterwards, the resultant product was dried at 80°C overnight, then ground and annealed for two hours at 500°C [Citation34].
2.3. Synthesis of exfoliated carbon (ExC)
Exfoliated carbon was synthesized by soaking 10 g of graphite flakes in 150 mL concentrated sulphuric acid (H2SO4, 98%) and 50 mL phosphoric acid (H3PO4, 70%) overnight. The graphite was then washed with deionized water several times until the pH reached 7. It was dried at 60–80°C in a vacuum oven overnight. The dried product was placed in a domestic oven (1000 W) for 2-3 min and ground to fine powder [Citation28].
2.4. Synthesis of NiO/ExC nanocomposites
Nanocomposites of nickel oxide and ExC were synthesized by the ultrasonication method. 0.09 g NiO, 0.08 g NiO and 0.07 g NiO were dispersed in 50 mL ethanol in separate beakers and sonicated for 2 h. Similarly, 0.001, 0.002 and 0.003 g of exfoliated carbon were dispersed in 50 mL ethanol in different beakers and sonicated for 2 h. Following sonication, 0.001 g exfoliated carbon solution was added to 0.09 g NiO suspension, while 0.002 and 0.003 g exfoliated carbon solution was applied to 0.08 g NiO and 0.07 g NiO suspensions, respectively, and sonicated for two hours. After sonication, the dark-coloured suspension was dried overnight in a vacuum oven at 80°C, and the material was finely ground.
2.5. Materials’ characterization
The morphology of the prepared materials was analyzed using a scanning electron microscope (SEM). X-ray diffraction patterns were taken by an X-ray diffraction instrument. All electrochemical measurements were done using GAMRY potentiostat 5000 E (Figure ).
2.6. Working electrode preparation
Nickel foam was used to prepare electrodes. A thin layer of the materials slurry prepared using ethanol and nafion binder was deposited on almost 1 cm2 of nickel foam. Nearly 0.001 g of the material was deposited on each electrode found by pre- and post-weight determination after drying the electrode. Similarly, electrodes of all the materials were prepared and dried [Citation35].
2.7. Electrochemical characterization
A system withthree electrodes was used for electrochemical measurements. The platinum (Pt) wire served as a counter electrode, and Ag/AgCl served as a reference electrode in this system. The frequency for EIS measurements was from 10000 Hz to 0.1 Hz. The potential window was 0–0.6 V for CV measurements.
3. Results and discussion
3.1. Powder XRD analysis
The X-ray diffraction patterns of hierarchically porous nickel oxide, exfoliated carbon and their nanocomposites are shown in Figure . The diffraction peaks of NiO were observed by X-ray diffraction (6100) instrument from Shimadzu with Cu Kα radiation at 40 kV and 50 mA at 37°, 42°, 62°, 75° and 77° 2 theta and were indexed as “(1 1 1)”, “(2 0 0)”, “(2 2 0)”, “(3 1 1)” and “(2 2 2)” diffraction planes, respectively which were crystallized in the cubic system. The correspondent X-ray diffraction pattern agreeing with JCPDS (card number 01-071-1179) confirms the formation of hierarchically porous NiO. There was no additional peak in the XRD pattern that ensures the high quality of the synthesized material. Unit Cell software was used to calculate XRD parameters. Unit cell length along the x-axis, y-axis and z-axis was 4.24 A°. The volume of a unit cell was 76.2 A°3. The crystallite size was calculated by the Scherer formula, as given in equation 1 [Citation36].
(1)
(1) In this equation, β is full-width half maxima value, λ is the X-rays wavelength (1.5406 nm), k denotes the shape factor (equals 0.9), and θ is the Bragg’s angle used to represent the diffraction angle for diffraction planes. The crystallite size calculated for ExC was 8.87 nm. While the ExC showed strong peaks at 2 theta = 26°, 45°, 54° and 77° at diffraction planes of “(0 0 2)”, “(1 0 1)”, “(0 0 4)” and “(1 1 0)”, respectively that ensures the hexagonal structure of ExC (JCPDS 01-075-1621). The typical and prominent peaks of the composites indicate the presence of components in composites, as shown in Figure . The pure ExC has the highest intensity peak “(0 0 2)”, while innanohybrids, it was suppressed; the reason could be that nanohybrids have a low weight percentage of ExC. The crystallite size of the ExC is 20.18 nm, unit cell length along the X-axis and Z-axis was 2.46 A° and 6.79 A°, respectively, while the unit cell volume calculated was 35.81 (A°)3. The specific surface area was calculated from XRD data by following Dp = 6000/S × ρ. In this formula, the Dp is the crystallite size of the material, S is the specific surface area and ρ is the density of the material [Citation37,Citation38]. The calculated specific surface area was 437 m2/g. This higher value of specific was only responsible for the mesoporous nature of the material.
3.2. SEM analysis
The morphological aspects were investigated by the SEM analysis. Synthesized NiO micro-spheres of SEM images are shown in Figure which shows that NiO was spherical . The walls of microspheres consist of nanosheets composed of small grain-size nanoparticles. Hierarchical NiO structure includes small grain-size particles, mesoporous nature and microporous nanosheets, which was also concluded from the specific surface area calculated from XRD results. SEM images of composites of NiO and ExC in Figure (a–c) show that NiO spheres were successfully suspended on carbon sheets.
3.3. FTIR analysis
FTIR spectroscopy was carried out to examine the functional groups present in the synthesized products; all the measurements were carried out by FTIR 6700 Nicolet TM Fourier transform infrared spectrometer, and the results are shown in Figure . The peaks observed at 3491 cm−1 were of O-H stretching. Peaks observed in the region of 1524 cm−1 depict bending vibrations by water molecules. The absorption band centred at 789 cm−1 is attributed to Ni-O stretching vibration [Citation39] that depicts the synthesis of NiO. While the IR peak at 3107 cm−1 is due to CH2 vibration that could be related to the presence of amorphous carbon in the sample [Citation40], while in the region of 1600–1800 cm−1, peaks indicate C=C stretching and peaks at 2346 were assigned to CO2 that came from the atmosphere. The FTIR results were following the results of XRD.
3.4. Electrochemical behaviour
The electrochemical evaluation of hierarchical NiO and its ExC-based nanocomposites was carried out by the cyclic voltammetry (CV) technique utilizing a 1 M KOH solution. The CV was attained at various scan rates such as 100, 80, 50, 30, 20, 10, and 5 mV/s, and specific capacity was calculated from eq2. The potential window was 0–0.6 V. CV profile is shown in Figure (a–d), CV curves demonstrate the observed oxidation and reduction peaks that corresponding to redox reactions. These peaks show that through faradic reaction, material is storing charge.
(2)
(2) The area enclosed by NiO was smaller, while the area enclosed by its composites with ExC was greater. The composite with greater concentration enclosed greater area than other composites. This area enclosed proposes that NiO material was storing charge poorly [Citation41] while composites were attributed due to the addition of ExC. The synergistic effects of faradic and non-faradic reactions are responsible for the enclosed larger area. The nanocomposites exhibited near quasi rectangular-shaped CV curves. This broadened CV loop revealed that the material followed faradic and non-faradic reactions to store the charge [Citation42]. The inclusion of exfoliated carbon increased capacitance while decreasing the internal resistance of the nanocomposites and increasing the surface area [Citation43]. The faradic redox reactions had good cyclic stability at the electrode surface, confirmed by the increased current reciprocation and shifting the redox peaks towards maximum potential.
Figure 5. CV profile of (a) hierarchically porous NiO, (b) 0.09 g NiO/0.001 g ExC nanocomposite (c), 0.08 g NiO/0.002 g ExC nanocomposite (d), 0.07 g NiO/0.003 g ExC nanocomposite (e), hierarchically porous NiO and (f) 0.007 g NiO/0.003 g ExC at 1st and 2000th cycles, (g) scan rate and specific capacity and (h) capacitance percentage retention of NiO and ExC-based nanocomposite.
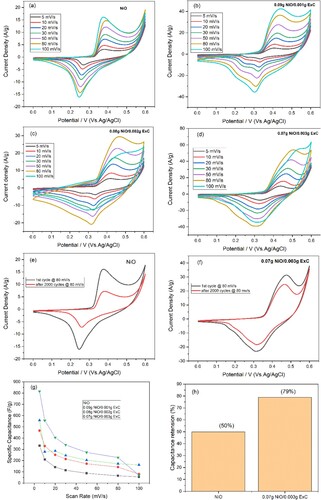
The effect of scan rate on capacitance is shown in Figure (g and h). Due to the increase in scan rate, capacitance decreases. At a higher scan rate in the charge-storage mechanism, only the surface of the electrode is involved due to insubstantial time. In contrast, comparatively large charges store at a lower scan rate, as ions have enough time to diffuse deeply into electrode material [Citation44]. To check the cyclic stability of hierarchically NiO microspheres and their ExC comprised composites, CV was done at 80 mV/s for 2000 cycles, as shown in Figure (e and f). In porous NiO, there is an observable decrease in the CV loop area that proposes that because of loss of mass from electrode and volume expansion, capacitance decreased [Citation45]. Compared to NiO, composites of NiO and ExC depicted better results even after 2000 cycles. It can be concluded from the Figure that capacitance retention in the case of NiO microspheres was 50%, while capacitance retention of 0.07 g NiO/0.003 g ExC was 79% after 2000 cycles.
Features considered responsible for excellent electrochemical properties were more conductive nature and high surface area of ExC, better diffusion of ions and hierarchically porous NiO. Nanocomposites were allowing better capacitance due to the synergistic effects of their process, and strong relations between components were the reasons for cyclic stability. ExC provided volume expansion to aid the redox reactions at the electrode–electrolyte interface [Citation46].
The galvanostatic charge discharge (GCD) measurements of 0.007 g NiO/0.003 g ExC were also carried out at 1, 3, 5, 7, 9 A/g current density, as shown in Figure . The results showed hybrid behaviour for charging electrode material. The specific capacitance and percent retention were calculated from these results and tabulated in Table . The GCD profiles of 0.007 g NiO/0.003 g ExC at different current density values are not linear and showed diffused plateau regions. These regions corresponded with the CV profiles of the material. Moreover, the GCD profile also indicates the dominance of reversible faradic reaction for charge storage mechanism in 0.007 g NiO/0.003 g ExC electrode. The material showed high specific capacity (795 F/g) at lower current density, and is reduced to 655.5 F/g at higher current density (9 A/g). It was a higher probability for electrode–electrolyte interaction taking place at lower current density and vice versa [Citation47,Citation48].
Table 1. Galvanostatic charge-discharge measurement results for 0.007 g NiO/0.003 g ExC.
Figure shows the Nyquist plot of hierarchically porous NiO and its ExC composed nanocomposite. The higher frequency region at the x-intercept and vertical line at the lower frequency region show the solvent resistance and Warburg resistance, respectively. Compared to hierarchically porous NiO, the ExC-based composite exhibits reduced solution resistance, as shown in Figure . The Warburg resistance shows the allocation of ions of electrolyte into the porous electrode. Diffusion resistance (Warburg resistance) decreases as the slope of the vertical line increases, implying that electrolyte ions will take a smaller route for appropriate diffusion. The slant line in the low-frequency region doesn’t indicate the pure capacitive behaviour. It suggests that NiO/ExC gets closer to the capacitive behaviour than NiO. The semicircle in the low-frequency region was not observed, which might be due to the ohmic connection of material with Ni foam [Citation49]. The equivalent circuit of EIS data was fitted, and the corresponding circuit diagram has been shown in Figure . The circuit components are in series as R(CR)(CR)O.
In Table , the specific capacitance of different NiO-based electrode materials is shown, where the synthesized material 0.07 g NiO/0.003 g ExC showed the specific capacity of 815 F/g, which is greater than other NiO-based electrodes. This electrode showed a more remarkable electrochemical behaviour because it contained a more significant percentage of ExC than other composites. This comparison indicates that NiO/ExC composite is a promising electrode material. Table .
Table 2. Summary of performance of NiO-based electrode materials.
4. Conclusion
Hierarchically porous NiO and its nanocomposites with ExC were successfully synthesized by the precipitation and the sonication method. All essential physio-chemical techniques characterized the synthesized materials. The electrochemical measurements, CV and EIS of the prepared materials, were performed to observe the electrochemical properties. EIS revealed that the ExC-based composite of NiO contains less resistance compared to porous NiO. Excellent electrochemical properties of ExC-based nanocomposites of NiO prove them as potential electrode material for energy storage devices.
Acknowledgements
The authors acknowledges the financial support of Taif University Researchers Supporting Project number (TURSP-2020/05), Taif University, Taif, Saudi Arabia. Authors are also thankful to the Institute of Chemistry (BJ-Campus), The Islamia University of Bahawalpur and Higher Education Commission (HEC) Pakistan.
Disclosure statement
No potential conflict of interest was reported by the author(s).
Additional information
Funding
References
- Prabunathan P, Sethuraman K, Alagar M. Mno 2-doped, polyaniline-grafted rice husk ash nanocomposites and their electrochemical capacitor applications. RSC Adv. 2014;4:47726–47734.
- Dr QP, Song Y, Lv H, et al. Ammonium-ion storage using electrodeposited manganese oxides. Angew Chem. 2020;60:5718–5722.
- Abas N, Kalair A, Khan N. Review of fossil fuels and future energy technologies. Futures. 2015;69:31–49.
- Sharma K, Arora A, Tripathi SK. Review of supercapacitors: materials and devices. J Energy Stor. 2019;21:801–825.
- Dong R, Song Y, Yang D, et al. Electrochemical in situ construction of vanadium oxide heterostructures with boosted pseudocapacitive charge storage. J Mater Chem A. 2020;8:1176–1183.
- Najib S, Erdem E. Current progress achieved in novel materials for supercapacitor electrodes: mini review. Nanoscale Adv. 2019;1:2817–2827.
- Buiel E, Development of lead-carbon hybrid battery/super capacitors, Proc. Advanced Capacitor World Summit, July 17, 19 2006.
- Lu Z, Chang Z, Zhu W, et al. Beta-phased Ni (OH) 2 nanowall film with reversible capacitance higher than theoretical Faradic capacitance. Chem Commun. 2011;47:9651–9653.
- Chavan UJ, Yadav AA. Electrochemical behavior of spray deposited mixed nickel manganese oxide thin films for supercapacitor applications. J Mater Sci Mater Electron. 2017;28:4958–4964.
- Gu T-T, Wu X-M, Dong Y-M, et al. Novel photoelectrochemical hydrogen peroxide sensor based on hemin sensitized nanoporous NiO based photocathode. J Electroanal Chem. 2015;759:27–31.
- Ferreira FF, Avendaño E. Reversible electronic charge transfer between Au nanoparticles and electrochromic NiO matrices upon electrochemical cycling. J Phys Chem C. 2007;111:16608–16612.
- Venter A, Botha JR. Optical and electrical properties of NiO for possible dielectric applications. S Afr J Sci. 2011;107:1–6.
- Jlassi M, Sta I, Hajji M, et al. Synthesis and characterization of nickel oxide thin films deposited on glass substrates using spray pyrolysis. Appl Surf Sci. 2014;308:199–205.
- Xu C, Hong K, Liu S, et al. A novel wet chemical route to NiO nanowires. J Cryst Growth. 2003;255:308–312.
- Byrappa K, Adschiri T. Hydrothermal technology for nanotechnology. Prog Cryst Growth Charact Mater. 2007;53:117–166.
- Alagiri M, Ponnusamy S, Muthamizhchelvan C. Synthesis and characterization of NiO nanoparticles by sol–gel method. J Mater Sci Mater Electron. 2012;23:728–732.
- Li J, Yan R, Xiao B, et al. Preparation of nano-NiO particles and evaluation of their catalytic activity in pyrolyzing biomass components. Energy Fuels. 2008;22:16–23.
- San X, Li M, Liu D, et al. A facile one-step hydrothermal synthesis of NiO/ZnO heterojunction microflowers for the enhanced formaldehyde sensing properties. J Alloys Compd. 2018;739:260–269.
- Qiao H, Wei Z, Yang H, et al. Preparation and characterization of NiO nanoparticles by anodic arc plasma method. J Nanomater. 2009.
- Chandra S, Kumar A, Tomar PK. Synthesis of Ni nanoparticles and their characterizations. J Saudi Chem Soc. 2014;18:437–442.
- Wu M-S, Huang Y-A, Yang C-H, et al. Electrodeposition of nanoporous nickel oxide film for electrochemical capacitors. Int J Hydrogen Energy. 2007;32:4153–4159.
- Wang Y-g, Xia Y-y. Electrochemical capacitance characterization of NiO with ordered mesoporous structure synthesized by template SBA-15. Electrochim Acta. 2006;51:3223–3227.
- Anandan b, Rajendran V. Morphological and size effects of NiO nanoparticles via solvothermal process and their optical properties. Mater Sci Semicond Process. 2011;14:43–47.
- Liu KC, Anderson MA. Porous nickel oxide/nickel films for electrochemical capacitors. J Electrochem Soc. 1996;143:124.
- Xing W, Li F, Yan Z-f, et al. Synthesis and electrochemical properties of mesoporous nickel oxide. J Power Sources. 2004;134:324–330.
- Zhang Y, Gui Y, Wu X, et al. Preparation of nanostructures NiO and their electrochemical capacitive behaviors. Int J Hydrogen Energy. 2009;34:2467–2470.
- Zhu T, Wang Z, Ding S, et al. Hierarchical nickel sulfide hollow spheres for high performance supercapacitors. RSC Adv. 2011;1:397–400.
- Jin J, Qiao X, Zhou F, et al. Interconnected phosphorus and nitrogen codoped porous exfoliated carbon nanosheets for high-rate supercapacitors. ACS Appl Mater Interfaces. 2017;9:17317–17325.
- Rafiq S, Aadil M, Warsi MF, et al. Nio nanoparticles and their nanohybrid with flat rGO sheets: As an ideal electroactive material for hybrid capacitor applications. Ceram Int. 2022.
- Zou Y, Cai C, Xiang C, et al. Simple synthesis of core-shell structure of Co–Co3O4@ carbon-nanotube-incorporated nitrogen-doped carbon for high-performance supercapacitor. Electrochim Acta. 2018;261:537–547.
- Lv H, Pan Q, Song Y, et al. A review on nano-/microstructured materials constructed by electrochemical technologies for supercapacitors. Nano Micro Letters. 2020;12:118.
- Soneda Y, Toyoda M, Tani Y, et al. Electrochemical behavior of exfoliated carbon fibers in H2SO4 electrolyte with different concentrations. J Phys Chem Solids. 2004;65:219–222.
- Toyoda M, Tani Y, Soneda Y. Exfoliated carbon fibers as an electrode for electric double layer capacitors in a 1 mol/dm3 H2SO4 electrolyte. Carbon. 2004;42:2833–2837.
- Qin Y, Zhang F, Chen Y, et al. Hierarchically porous CuO hollow spheres fabricated via a one-pot template-free method for high-performance gas sensors. J Phys Chem C. 2012;116:11994–12000.
- Aadil M, Zulfiqar S, Shahid M, et al. Binder free mesoporous Ag-doped Co3O4 nanosheets with outstanding cyclic stability and rate capability for advanced supercapacitor applications. J Alloys Compd. 2020;844:156062.
- Barzinjy AA, Hamad SM, Aydın S, et al. Green and eco-friendly synthesis of nickel oxide nanoparticles and its photocatalytic activity for methyl orange degradation. J Mater Sci Mater Electron. 2020;31:11303–11316.
- Theivasanthi T, Alagar MJ. An insight analysis of nano sized powder of jackfruit seed. 2011.
- Park JY, Lee YJ, Jun KW, et al. Chemical synthesis and characterization of highly oil dispersed MgO nanoparticles. J Ind Eng Chem. 2006;12:882–887.
- Shuihab A, Khalf S. Fabrication and characterization of nickel oxide nanoparticles/silicon NiO NPS/Si, AIP Conference Proceedings, AIP Publishing LLC, 2018, pp. 020026.
- Scheibe B, Tadyszak K, Jarek M, et al. Study on the magnetic properties of differently functionalized multilayered Ti3C2Tx MXenes and Ti-Al-C carbides. Appl Surf Sci. 2019;479:216–224.
- Sabeeh H, Zulfiqar S, Aadil M, et al. Flake-like MoS2 nano-architecture and its nanocomposite with reduced Graphene Oxide for hybrid supercapacitors applications. Ceram Int. 2020;46:21064–21072.
- Aadil M, Shaheen W, Warsi MF, et al. Superior electrochemical activity of α-Fe2O3/rGO nanocomposite for advance energy storage devices. J Alloys Compd. 2016;689:648–654.
- Aadil M, Zulfiqar S, Sabeeh H, et al. Enhanced electrochemical energy storage properties of carbon coated Co3O4 nanoparticles-reduced graphene oxide ternary nano-hybrids. Ceram Int. 2020;46:17836–17845.
- Shakir I, Almutairi Z, Shar SS, et al. Nickel hydroxide nanoparticles and their hybrids with carbon nanotubes for electrochemical energy storage applications. Results Phys. 2020;17:103117.
- Li X, Dhanabalan A, Wang C. Enhanced electrochemical performance of porous NiO–Ni nanocomposite anode for lithium ion batteries. J Power Sources. 2011;196:9625–9630.
- Wang G, Ling Y, Qian F, et al. Enhanced capacitance in partially exfoliated multi-walled carbon nanotubes. J Power Sources. 2011;196:5209–5214.
- Khan M, Warsi M, Zulfiqar S. Journal pre-proof nanostructured V2O5 and its nanohybrid with MXene as an efficient electrode material for electrochemical capacitor applications. Ceram Int. 2022;48(2):2345–2354.
- Khan M, Zulfiqar S, Shahid M, et al. Fabrication of rationally designed CNTs supported binary nanohybrid with multiple approaches to boost electrochemical performance. J Electroanal Chem. 2021;884.
- Premathilake D, Outlaw RA, Parler SG, et al. Electric double layer capacitors for ac filtering made from vertically oriented graphene nanosheets on aluminum. Carbon. 2017;111:231–237.
- Xu J, Wu L, Liu Y, et al. NiO-rGO composite for supercapacitor electrode. Surf Interfaces. 2019;18:100420.
- Bu Y, Wang S, Jin H, et al. Synthesis of porous NiO/reduced graphene oxide composites for supercapacitors. J Electrochem Soc. 2012;159:A990–A994.
- Chernysheva D, Pudova L, Popov Y, et al. Non-isothermal decomposition as efficient and simple synthesis method of NiO/C nanoparticles for asymmetric supercapacitors. Nanomaterials. 2021;11:187.
- Pang H, Ma Y, Li G, et al. Facile synthesis of porous ZnO–NiO composite micropolyhedrons and their application for high power supercapacitor electrode materials. Dalton Trans. 2012;41:13284–13291.