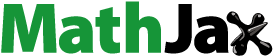
Abstract
In this work, a phenomenological model (PM) is used to simulate the magnetocaloric effect (MCE) of GdMn1-xCrxO3 (0 ≤x ≤0.4) (GMCO) samples through the modelling of experimental isofield thermo-magnetization curves. The results showed that the MCE of GMCO samples depends strongly on Cr content, achieving a conventional MCE for low Cr content (level of doping x ≤ 0.3). However, the MCE of a higher Cr content sample has an inverse MCE. The behaviour of MCE in GMCO samples indicated that GMCO compounds are interesting magnetocaloric materials and can fruitfully be functioned as cryogenic magnetic refrigerants below 40 K especially in radiation detectors for outer space research.
1. Introduction
With accelerated steps, the magnetic refrigerator (MR) has become a strong alternative to the gas refrigerator because of its more efficient cooling performance, less weight, more mechanical stability, less damage to the environment, and more energy savings [Citation1–6]. MR is an essential requirement in aerospace applications, medical devices, space applications, and food cooling [Citation7–14]. MR relies on the idea of applying the magnetocaloric effect (MCE) to magnetocaloric (MC) materials in the range of temperatures close to the temperature of the magnetic phase transition [Citation15–20]. The MCE is a phenomenon in which magnetic entropy change (ΔSM) of MC materials occurs when they are subjected to a change in an external magnetic field (Hexe) [Citation21–28]. In order for the development and improvement of MR to happen, the researchers studied different types of magnetic materials such as magnetic alloys, manganites and others [Citation29–36]. In a traditional MCE, the cooling action in MC material happens as a reaction of adiabatic demagnetization process that is performed by a sudden eliminating of Hexe [Citation37]. Quite the reverse, MC materials can be cooled via adiabatic magnetization, which is done by increasing Hexe on diamagnetic materials. This effect is termed an “ inverse MCE” [Citation38]. This inverse MCE appears clearly in antiferromagnetic (AFM) materials over the temperature range of the AFM transition.
There is a strong interest in studying rare earth manganites owing to unusual magnetic ordering such as AFM and spin reorientation as results of unusual spin arrangements [Citation39–41]. Manganites of the RMnO3 type usually contain undersized trivalent R ions, such as GdMnO3, and exhibit ferroelectricity caused by magnetic interaction competition, evoking an AFM spin ordering that results in lattice modulations [Citation42]. Tiwari et al. investigated GdMn1-xCrxO3 (GMCO) with 0 ≤x ≤0.4 prepared via sol–gel technique showing well to do sequence of magnetic transitions and recommending that GMCO compounds can be functioned in the fields of magnetic switching, MR and spintronic applications [Citation42]. From this practical point, the MCE of GMCO compounds is studied in this work. In this research, a phenomenological model (PM) is used to study the thermomagnetic properties through the work of simulated magnetization temperature curves for GMCO, concluding magnetic entropy change (ΔSM), heat capacity change (), and relative cooling power (RCP).
2. Theoretical considerations
According to PM, described in [Citation43, Citation44], the dependence of magnetization (M) on temperature (T) is given by:
(1)
(1) where
is an initial value of magnetization at a ferromagnetic (FM) or AFM-paramagnetic transition and
is a final value of this transition as shown in Figure , where
,
for FM or AFM phase, and
.
3. Results and discussion
To simulate the MCE of GMCO, PM parameters for GMCO were determined directly from experimental data (magnetization vs. temperature) as in Ref. [Citation42]. Figure shows the magnetization vs. temperature for GMCO where the experimental data from Ref. [Citation42] are symbolized by symbols, while the simulated data are symbolized by dashed lines. The PM parameters are tabulated in Table . We can realize from Figure that there is a satisfied agreement between the experimental and the theoretical ones of M(T) for GMCO samples under 0.05 T, approving PM is a good model for fitting magnetization vs. temperature. The M(T) curves of GMCO samples reveal that GMCO samples are showing a clear second order magnetic transition. of GMCO samples increases slightly with Cr content. Interestingly, when x is 0.4, a magnetization reversal is observed in GMCO, which expects the existence of an inverse MCE, as we will show later.
Figure 2. Magnetization vs. temperature for GMCO samples in Hexe of 0.05 T. The dashed curves are modelled results and symbols represent experimental data from Ref. 42.
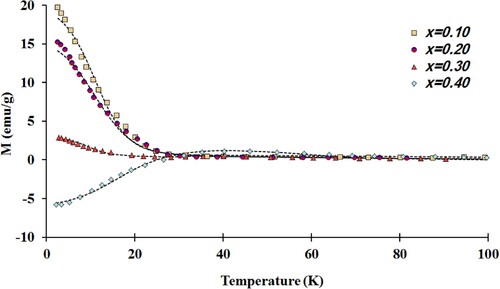
Table 1. The PM parameters for GMCO at Hexe = 0.05 T.
of GMCO samples under adiabatic magnetic field shift (ΔH) of 0.05 T is formulated by.
(2)
(2)
A maximum of (ΔSMax) can be determined when T = TMPT as follows:
(3)
(3)
Figure shows the simulated temperature dependence of ΔSM for GMCO samples under ΔH of 0.05 T, calculated by using Equation (2). Interestingly, of GMCO depends strongly on Cr content, concluding that the thermomagnetic property of GMCO is characterized as a conventional MCE for low Cr content (level of doping x ≤ 0.3). However, the thermomagnetic property of GMCO is characterized as an inverse MCE when the level of doping x is 0.4 as a result of magnetization reversal below the compensation temperature (Tcomp), at which the opposing magnetic moments are equal. But, above Tcomp, the thermomagnetic of GMCO characterizes as a conventional MCE with a very small value of
as a result of a FM transition. This magnetization reversal, which causes an inverse MCE below Tcomp when the level of doping x is 0.4, is due to negative exchange interaction between Gd and Cr ions. At below Tcomp, when Hexe is applied, the spins of Cr3+ are directed along the Hexe direction while the spins of Gd3+ are directed opposite to that of the Hexe direction. At the same time, the magnetic moment of Gd3+ is more than magnetic moment of Cr3+ ion. Therefore, the net magnetization of the highly Cr content sample is negative value. However, at above Tcomp, when Hexe is applied, magnetic moment of Cr3+ is greater than the magnetic moment of Gd3+ resulting in a net positive magnetization, causing a conventional MCE.
The effectiveness of GMCO samples as MC material can be evaluated by RCP. This parameter is accounted for by the absolute value of ΔSMax and full-width at half-maximum () of the ΔSM curve by following the formula:
(4)
(4) where
can be obtained as follows:
(5)
(5)
Table indicates that has moderate values and is ranged between 11.3 and 25.5 K for GMCO samples under ΔH of 0.05 T. Furthermore, MC properties decrease dramatically with Cr content.
Table 2. The predicted values of MC properties for GMCO under ΔH of 0.05 T.
According to the PM model [Citation43], the characterization of curves of the GMCO samples can be predicted as follows.
(6)
(6) Figure shows the temperature dependence of simulated ΔCP,H for GMCO samples under ΔH of 0.05 T. For low Cr content (level of doping x ≤ 0.3), the simulated
varies from a negative value to a positive one around
, adding a change in the total specific heat. However, the inversed characterization is observed when level of doping x is 0.4 as the result of magnetization reversal below Tcomp. But, above Tcomp, the simulated
has the same characterization of lower Cr content samples (level of doping x ≤ 0.3) as a result of the FM transition. The oscillating temperature dependence of
at temperatures is a reflection of ΔSM behaviour due to different exchange interactions between Gd and Cr ions below and above 40 K as explained before [Citation42].
To investigate further details about MCE in GMCO samples, the refrigerant capacity (RC) is well-thought-out to judge the efficiency of GMCO samples as effective MC materials in MR. RC was calculated as follows [Citation44]:
(8)
(8)
Form Table , RC decreases with Cr content for a level of doping x ≤ 0.3. However, for further Cr content, RC improves with Cr content. Table gives a comparative image of MCE parameters of GMCO samples with the corresponding ones of several MC materials in same value of ΔH and higher ones in published works. Importantly, the MCE parameters of GMCO samples are higher or as good as the corresponding MCE parameters of these published works with the same value of ΔH or larger. Interestingly, although the of ΔH in the published Gd single crystal is twenty times of the best investigated GMCO sample, |ΔSMax| of Gd single crystal is larger about 5.5 times. Moreover, GMCO samples are effective MC materials below 40 K due to the sequence of magnetic transitions below this temperature.
Table 3. The values of MC properties for GMCO and other MC materials.
Finally, GMCO samples are interesting MC materials in cryogenic MR below 40 K especially for devices that function in very low temperatures. For instance, in very low temperature radiation detectors for outer space investigation, where the gravitational effect is non-effective, it is very hard to use 4He−3He dilution refrigerators [Citation45]. Therefore, these GMCO compounds are practical MC materials in this situation to overcome the difficulty of working 4He−3He dilution refrigerators.
4. Conclusion
The simulation of MCE GMCO compounds containing different Cr content has been done via PM. The results of simulation show that this PM is a constructive model for the calculation of thermomagnetic properties for GMCO compounds. The MCE of GMCO samples depends strongly on Cr content, achieving a conventional MCE for low Cr content (level of doping x ≤ 0.3). However, the MCE of GMCO has a type of inverse MCE when the level of doping x is 0.4 below Tcomp. The opportunity to control from conventional MCE to inverse MCE via the level of Cr doping in GMCO increases the prospects for the design of MR. The behaviour of MCE in GMCO samples indicates that they are promising candidates in the cryogenic MR below 40 K.
Disclosure statement
No potential conflict of interest was reported by the author(s).
References
- Hsini M, Hcini S, Zemni S. Study of magnetic entropy change in Nd0.67Ba0.33Mn0.98Fe0.02O3 by means of theoretical models. J.Supercond. Nov. Magn. 2018;31:81.
- Wang X, Wu J, Dkhil B, et al. Enhanced electrocaloric effect near polymorphic phase boundary in lead-free potassium sodium niobate ceramics. Appl. Phys.Lett. 2017;110:063904.
- Ahmed EM, Hemeda OM, Alamri HR, et al. Magnetocaloric Effect in α'-MnB Nanoparticles. Russ J Phys Chem. 2022;96:S101–S104.
- Hamad MA, Alamri HR. α-MnO2 nanorods’ magnetocaloric effect for hydrogen liquefaction. J Supercond Nov Magn. 2022;35:515–518.
- Alzahrani B, Hsini M, Hcini S, et al. Study of the magnetocaloric effect by means of theoretical models in La0.6Ca0.2Na0.2MnO3 manganite compound. J. Low Temp. Phys. 2020;200:26–39.
- Hamad MA. Magnetocaloric effect in Sr2FeMoO6/Ag composites. Process.Appl.Ceram. 2015;9:11.
- Bai Y, Wu X, Zhao S. Oxygen vacancy modulating inverse and conventional magnetocaloric effects coexisting in double perovskite Bi2NiMnO6-δ films. Ceram Int. 2021;47:6614–6622.
- Ewas AM. Large magnetocaloric effect of La0.67Pb0.33Mn1−xCoxO3 in small magnetic field variation. Ceram. Int. 2017;43:7660.
- Ahmed E, Hemeda O, Alamri H, et al. Investigation on Magnetocaloric Effect in Sc Doped Th2NiC2 Superconductors. Phys. Metals Metallogr. 2021;122:1454–1457.
- Mechi N, Alzahrani B, Hcini S, et al. Correlation between magnetocaloric and electrical properties based on phenomenological models in La0.47Pr0.2Pb0.33MnO3perovskite. Phase Trans. 2018;91:559.
- Bourouina M, Krichene A, Boudjada NC, et al. Structural, magnetic and magnetocaloric properties of nanostructured Pr 0.5 Sr 0.5 MnO 3 manganite synthesized by mechanical alloying. Ceram. Int. 2017;43:8139.
- Abdelalim Y, Mohamed A, Hemeda O, et al. Simulation of magnetocaloric properties in La1-xBaxCoO3. Journal of Magnetism and Magnetic Materials. 560;120:169661.
- Dhahri A, Jemmali M, Dhahri E, et al. Structural characterization, magnetic, magnetocaloric properties and phenomenological model in manganite La0.75 Sr0.1Ca0.15 MnO3 compound. J. Alloy Compd. 2015;638:221.
- Ahmed E, Alamri H, Elghnam S, et al. Tuning Magnetocaloric Properties for La1 – xSrxCoO3. Phys. Solid State. 2021;63:1601–1604.
- Jeddi M, Gharsallah H, Bekri M, et al. Phenomenological modeling of magnetocaloric properties in 0.75La0.6Ca0.4MnO3/0.25La0.6Sr0.4MnO3 nanocomposite manganite. J.Low Temp.Phys. 2020;198:135.
- Sfifir I, Koubaa W, Koubaa M, et al. Phenomenological model of magnetocaloric effect in La0.7Ca0.2Ba0.1MnO3 manganite around room temperature. J.Supercond. Nov. Magn. 2017;30:911.
- Zarai E, Issaoui F, Tozri A, et al. Critical behavior near the paramagnetic to ferromagnetic phase transition temperature in Sr1.5Nd0.5MnO4 compound. J.Supercond. Nov. Magn. 2016;29:869.
- Thandapani P, Ramalinga M, Denardin JC. Magnetocaloric effect and universal curve behavior in superparamagnetic zinc ferrite nanoparticles synthesized via microwave assisted co-precipitation method. Phys. Status Solidi (a). 2018;215:1700842.
- Dung NT, Pham Y, Lam DS, et al. Critical behavior of polycrystalline Pr0.7Ca0.1Sr0.2MnO3 exhibiting the crossover of first and second order magnetic phase transitions. J. Mater. Res. Technol. 2020;9:12747.
- Zhang KS, Xue JN, Wang YX, et al. Magnetocaloric effect and corrosion resistance of La(Fe, Si)13composite plates bonded by different fraction of phenolic resin. AIP Adv. 2018;8:048104.
- Ahmed EM, Hemeda OM, Alamri HR, et al. Investigation of thermomagnetic properties in Ca3Co2O6over cryogenic temperature between 0 and 100 K. Phase Trans. 2021;94:835–841.
- Mohassel R, Amiri M, Abbas AK, et al. Pechini synthesis using propylene glycol and various acid as stabilizing agents and characterization of Gd2NiMnO6 ceramic nanostructures with good photocatalytic properties for removal of organic dyes in water. J. Mater. Res. Technol. 2020;9:1720.
- Hussain I, Khan SN, Kim EJ, et al. Nb modified structural, magnetic and magnetocaloric properties of double perovskite Ba2FeMo1−xNbxO6. Ceram. Int. 2018;44:2892.
- Hcini S, Boudard M, Dhahri A, et al. Magnetocaloric effect study by means of theoretical models and spontaneous magnetization determination in Ni0.4Mg0.3Cu0.3Fe2O4ferrite. Mater Res Express. 2019;6:066108.
- El Kossi S, Dhahri J, Hlil EK. Structural, magnetic and theoretical investigations on the magnetocaloric properties of La0.7Sr0.25K0.05MnO3perovskite. RSC Adv. 2016;6:63497.
- Barclay J, Brooks K, Cui J, et al. Propane liquefaction with an active magnetic regenerative liquefier. Cryogenics. 2019;100:69–76.
- El-Sayed AH, Hamad MA. Tailoring thermomagnetic properties in Pb(Zr0.52Ti0.48)O3–Ni(1−x)ZnxFe2O4. Phase Trans. 2019;92:517.
- Qian Y, Ma X, Si X, et al. The analysis of magnetocaloric effect and magnetic critical behavior in Mn5Ge3−xAgxcompounds. Phys Scr. 2020;95:065701.
- Hamad MA, Hemeda OM, Mohamed AM. Investigations on enhancing thermomagnetic properties in CoxZn1−xFe2O4. J.Supercond.Nov.Magn. 2020;33:2753–2757.
- Mandal K, Das SC, Dutta P, et al. Effect of non-magnetic Ag-doping on magnetic and magnetofunctional properties of MnNiGe alloy. J. Alloys Comp. 2020;822:153454.
- Singh V, Bag P, Rawat R, et al. Re-epithelialization and immune cell behaviour in an ex vivo human skin model. Sci Rep. 2020;10:1–17.
- Kokila IP, Kumar PS, Kanagaraj M, et al. Multiple magnetic phase transition and short-range ferromagnetic behavior influence on magnetocaloric effect of Sm2NiMnO6 nanoparticles. J. Nanoparticle Res. 2020;22:1–10.
- Mandal PR, Khan A, Nath TK. Antisite disorder driven magnetodielectric and magnetocaloric effect in double perovskite La2−xSrxCoMnO6(x = 0.0, 0.5, 1.0). J. Appl. Phys. 2020;128:024104.
- Barman A, Chatterjee S, Dey JK, et al. Interface-induced enhanced magnetocaloric effect in an epitaxial Co Fe 2 O 4/La 0.7 Sr 0.3 Mn O 3 heterostructure. Phys.Rev.B. 2020;102:054433.
- Fkhar L, Mounkachi O, El Maalam K, et al. Large magnetic entropy change in Pr2/3Sr1/3MnO3-CuO Composite at Room Temperature. J.Supercond.Nov.Magn. 2019;32:3579.
- Alamri H, Elghnam S, Hemeda O, et al. Hysteresis Energy Loss of Nanocrystalline CoFe2O4 Synthesized by Modified Citrate-Gel Method. Phys. Solid State. 2021;63:1332–1336.
- Liu Y, Zhang X, Xing D, et al. Magnetocaloric effect (MCE) in melt-extracted Ni–Mn–Ga–Fe Heusler microwires. J. Alloys Comp. 2014;616:184.
- Moya X, Mañosa L, Planes A, et al. Cooling and heating by adiabatic magnetization in the Ni 50 Mn 34 In 16 magnetic shape-memory alloy. Phys.Rev.B. 2007;75:184412.
- Magesh J, et al. Strong enhancement of magnetoelectric coupling in Dy3+doped HoMnO3. Appl. Phys. Lett. 2012;101:1–4.
- Kurbakov AI, Ryzhov VA, Runov VV, et al. Study of phase separation phenomena in half-doped manganites with isovalent substitution of rare-earth cations on example of Sm 0.32 Pr 0.18 Sr 0.5 MnO 3 . Phys.Rev.B. 2019;100:184424.
- Ferreira WS, Moreira JA, Almeida A, et al. Spin-phonon coupling and magnetoelectric properties: EuMnO 3 versus GdMnO 3. Phys. Rev. B. 2009;79:054303.
- Tiwari P, Kumar S, Rath C. Probing structural transformation and optical and magnetic properties in Cr doped GdMnO3: Jahn–Teller distortion, photoluminescence and magnetic switching effect. RSC Adv. 2019;9:39871.
- Hamad MA. Prediction of thermomagnetic properties of La0.67Ca0.33MnO3and La0.67Sr0.33MnO3. Phase Trans. 2012;85:106.
- Hamad MA. Theoretical work on effect of pressure on magnetocaloric properties of $${La}_{0.7}{Ca}_{0.3}{MnO}_{3}$$ La 0.7 Ca 0.3 MnO 3. Int. J. Thermophys. 2015;36:2748.
- Evangelisti M, Candini A, Affronte M, et al. Magnetocaloric effect in spin-degenerated molecular nanomagnets. Phys.Rev.B. 2009;79:104414.
- Hamad MA. Magnetocaloric effect in La1−x Cd x MnO3. J.Supercond.Nov.Magn. 2013;26:3459.
- Hemeda OM, Mostafa NY, Abd Elkader OH, et al. Electrical and morphological properties of magnetocaloric nano ZnNi ferrite. J.Magn.Magn.Mater. 2015;394:96–104.
- El-Sayed AH, Hamad MA. Nickle concentration effect on low magnetic field magnetocaloric properties for Ni2+xMn1−xGe. J.Supercond.Nov.Magn. 2019;31:1447.
- Hamad MA. Magnetocaloric effect in (001)-Oriented MnAs thin film. J.Supercond.Nov.Magn. 2014;27:263.
- Hamad MA. Magnetocaloric effect in polycrystalline Gd1−xCaxBaCo2O5.5. Materials Lett. 2012;82:181.
- Hamad MA. Magnetocaloric effect in La1.25Sr0.75MnCoO6. J.Therm.Anal.Calorim. 2014;115:523.
- Amirov AA, Makoed II, Chaudhari YA, et al. Magnetocaloric effect in BiFe1−xZnxO3 multiferroics. J.Supercond.Nov.Magn. 2018;31:3283.
- Masrour R, Jabar A, Benyoussef A, et al. Monte Carlo simulation study of magnetocaloric effect in NdMnO 3 perovskite. J.Magn.Magn.Mater. 2016;401:91–95.
- Hamad MA. Magneto-caloric effect in Ge0.95Mn0.05 Films. J.Supercond.Nov.Magn. 2013;26:449.
- Amirov AA, Makoed II, Chaudhari YA, et al. Magnetocaloric effect in BiFe1−xZnxO3 multiferroics. J.Supercond.Nov.Magn. 2018;31:3283.
- El-Sayed AH, Hamad MA. Phenomenological modeling of magnetocaloric effect for Ni58Fe26Ga28 Alloy. J.Supercond.Nov.Magn. 2018;31:1895.
- Ba DN, Zheng Y, Becerra L. Magnetocaloric effect in flexible, free-standing gadolinium thick films for energy conversion applications. Phys Rev Appl. 2021;15:064045.