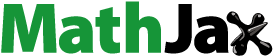
Abstract
The advancement of energy conversion and storage frameworks depends significantly on the development of reliable, effective, and affordable electrocatalysts. Steadily, there is an increasing demand of energy, fossil fuels consumption, and alarming environmental issues that have induced researchers to find alternate renewable sources. For this purpose, water splitting using various electrocatalysts have been investigated in a highly favourable virtual environment and is a highly demanding challenge to fulfil the energy crises. Here in this work, we fabricated three-dimensional (3D) nanosheet network NiO/CoSe2/NF nanocomposite (where NF represents nickel foam) material via hydrothermal treatment. All the synthesized materials like NiO/NF, CoSe2/NF, and NiO/CoSe2/NF nanohybrid are tested for the oxygen evolution process in a basic medium (1.0M KOH). Among all directly grown NiO/CoSe2 3D nanosheet has shown the lowest overpotential (263 mV), Tafel slope value (38 mVdec−1), which is remarkably improved as compared to the pristine NiO/NF and CoSe2. The resultant enhanced performance is due to improved morphological characteristics, increased surface area, and electrochemical coupling of electrons between NiO/NF and CoSe2/NF due to the synergistic effect.
1. Introduction
Due to the population explosion, the world’s energy demand has expanded significantly during the last few years [Citation1–3]. To cope with this energy demand, researchers are considering long-term and valuable energy solutions. The availability of fossil fuels is rapidly diminishing, and their use has led to a number of environmental issues like acid rain, the greenhouse effect, and photochemical smog [Citation4,Citation5]. In order to produce clean energy alternatives to non-renewable fuel, it is mandatory to discover pure and sustainable energy resources, like wind, solar, and tidal power [Citation4,Citation6]. Through the electrolysis of water, hydrogen energy generation is considered a promising technical scheme for generating clean energy [Citation4,Citation7–9]. Consequently, innovations that utilize water electrolysis are essential for creating sustainable, renewable power. Noble metals like ruthenium oxide or iridium oxide were used by advanced technologies to speed up the production of oxygen through the OER, while platinum-derived materials, which are made of precious metals, were thought to produce hydrogen through the HER [Citation4,Citation10]. Besides this, there is less availability and scarcity of these materials have pushed researchers to venture into discovering new cost-effective and efficient materials [Citation11–15].
Numerous materials have been used to inspect electrocatalysts’ performance for OER, i.e., semiconductors, conductive polymers, transition metal chalcogenides (TMCs), metal–organic framework (MOF), carbon-based materials, and oxides, etc. Nowadays, oxides of a transition metal like NiO [Citation4,Citation16], Co3O4[Citation17], CuO2[Citation17], CuO/Co3O4/rGO [Citation18], CuO/Co3O4 [Citation19], and nickel chalcogenides such as CuSbSexS2-x [Citation20], NiSe2@Fe3SO4 [Citation21] have been analysed for water splitting due to their large earth reservoirs, cost-effective and environmentally sustainable features. However, the deceptive performance of individual metal oxides is usually dissatisfied because of their unwanted adsorption energies (ΔE) of the reaction intermediates and poor conductivity [Citation22,Citation23].
There are many techniques are employed to enhance the electrochemical efficiency of metal oxides, i.e., using conductive carbon frames, introducing hetero-interfaces and doped elements [Citation24–27]. Furthermore, improved heterointerfaces among several active phases in heterostructured electrocatalyst may display intriguing electrical properties that improve electrochemical performance. Additionally, the presence of crystal defects may alter the local surface charge and, consequently, the electrical properties of catalysts, causing an increase in the activity of certain catalysts [Citation28,Citation29]. Scientists have recently attempted to construct a bifunctional electrocatalyst possessing the ability to catalyze both of the HER and OER processes [Citation30,Citation31]. Consequently, integrating various half-reaction centres into multi-component arrangement might all together increase both HER and OER in the same electrolyte media that can make a more accessible functioning system. Furthermore, it has been described that the low-spin state’s metals (e.g. Co, Mo, W) generally display diverse oxidation states, and hence their oxides provide a reversible surface oxygen ion interchange capacity, which could simplify the development of O* while performing OER. Therefore, introducing metal chalcogenides into the metal oxides system build a novel multi-substituent catalyst with various heterointerfaces might contribute to grow a highly proficient material. On the other hand, the interrelated studies addressing this subject matter were still infrequent and the fundamental mechanism is not very clear. Thus, fabricating a hybrid electrode with exceptional OER activities and stability at low cost remains a big task, particularly when demanding the huge evolution of current density in profitable commercial water-splitting tools [Citation32–34].
Here, we have reported a fabrication of a 3D nanosheet-like structure of NiO/CoSe2to design a novel nanohybrid directly grown on NF via hydrothermal method as an electrocatalyst for OER using Ni(NO3)2·6H2O, CoCl2. 6H2O and Selenium powder as precursors. In a basic medium, under oxidizing potential, chalcogenides are thermodynamically unstable as compared to hydroxides and oxides. Instability is prevented by the use of chalcogenides with oxides as an electrode material. Therefore, the synergistic interactions between two domains like NiO/NF and CoSe2/NF created abundant active interfaces zones enhancing the electrochemical performance of OER compared to its counterparts. As expected, NiO/CoSe2/NF nanosheet attained an especially synergistically enhanced process for OER in 1.0 M KOH electrolyte. NiO/CoSe2/NF nanohybrid attained lower Tafel slope (38 mVdec−1) and lower overpotential of 263 mV at 10 mA cm−2 for oxygen evolution process.
2. Experimental section
2.1. Chemicals
Nitric acid (Merck 65%), Nickel nitrate hexahydrate Ni(NO3)2.6H2O (Sigma Aldrich-99.9%), Ammonia solution (Merck-25%), ethanol (Analar 99.8%), potassium hydroxide (KOH, 85%, Riede-de-Haen), selenium powder (Se), (99.99%Sigma Aldrich), cobalt chloride hexahydrate (CoCl2.6H2O, 97%, Merck), Acetone, Normapur 99.8% Hydrazine Monohydrate (extra pure, Dae-Jung), and deionized water (DI). None of the compounds were further purified before application.
2.2. Fabrication of pristine NiO/NF
0.05 M nickel nitrate hexahydrate was diluted in 80 mL distilled water and agitated for 15 min to create nickel oxide (NiO) using a hydrothermal technique. The 0.05M ammonium mixture was then constantly stirred for 30 min on the magnetized hot plate while being added dropwise to the aforementioned mixture. The obtained solution was then put into a 100 mL thermal reactor and heated to 180 °C for 12 h before being allowed to cool at ambient temperature. Using centrifugation at a speed of 3000 rpm, the resulting mixture was separated from the solvent once the sealed autoclave had naturally cooled. Following centrifugation, the resulting mixture was repeatedly rinsed with both water and ethanol, respectively. The final step was to grind the produced material into a fine powder after it had been desiccated at 60°C for 24 h.
2.3. Fabrication of NiO/ CoSe2/NFnanohybrid
To synthesize NiO/CoSe2/NF nanohybrid, a simple, ecofriendly hydrothermal method was used to get the fine crystal and controlled morphology. In this emblematic procedure, 0.1 mM selenium powder was dissolved in an alkaline medium (10 mL of 6.0M KOH solution) under continuous stirring on the magnetic hot plate. Then after 20 min of stirring, 2 mL of N2H4 solution was inserted dropwise into the aforementioned mixture. The obtained resulting mixture was continuously stirred for 2 h at ambient temperature. After the complete dissolution of selenium powder then, 0.1 mM cobalt chloride hexahydrate was added. Afterward, the as-prepared NiO/NF (0.05 g) nanoparticles were inserted into the mixture and stirred again for 10 min on the magnetic stir plate. The final mixture was previously poured into a 50 mL autoclave and then heating for 15 h at 180°C in the oven. Following a 15-h heat treatment, the autoclave was rinsed several times with absolute C2H5OH and H2O, dried at 60°C for overnight and stored for further characterization. For comparison purposes, individual CoSe2 was also prepared with the same method. The whole process is displayed in Scheme 1.
2.5. Material characterization
Powder X-ray diffraction (PXRD) was accomplished via advance Bruker D-8 using Cu Kα radiation at a voltage of 20 kV for structure and phase determination. Scanning electron microscopic (SEM) analysis (Hitachi s-4800) was used to detect the material’s morphology. Using the nitrogen adsorption/desorption route, Calculations were made of the Brunauer–Emmett–Teller (BET) contact area and diameter and distribution of pores. Energy-dispersive X-ray spectroscopy (EDS) was used using the JSM-5190 spectrometer to determine the constituent composition.
2.6. Electrochemical evaluations
A typical three-electrode system with a potentiostat was used to conduct all of the electrochemical measurement at ambient temperature. A saturated silver/silver chloride (Ag/AgCl) electrode as the reference electrode, a Pt wire served as the counter electrode, and the synthesized compound (5 mg) was coated on nickel foam (NF) as the working electrode in an electrometer. In 1.0M KOH solution, all electrochemical processes were investigated using the CV and LSV test at 5mVs−1 with iR correction. Electrochemical impedance spectra (EIS) were obtained in the operated condition of 100 kHz to 1.0 Hz and 5 mV. All the measurements are repeated at room temperature to ensure that they were as precise as feasible. The RHE potential of the Ag/AgCl reference electrode was calculated using Equation (1).
(1)
(1)
E(vs Ag/AgCl) shows the potential evaluated using Ag/AgCl as the reference electrode, while 0.059 indicates a correction factor, pH is the electrolyte’s pH, and E is the standard thermodynamic potential (0.197).
Equation (2) was used to compute the electrochemical surface area (ECSA) using cyclic voltammetry (CV) in a non-redox zone (0.9–1.02 V vs. RHE) in 1.0 M KOH electrolye at multiple scan speeds (5, 10, 15, 20, and 25 m Vs−1). The resultant change between the anodic and cathodic current levels at 1.92 V (V vs RHE) was estimated from cyclic voltammogram curves, while Δj value was taken at a point where clear separation occurred between the CV curves at various scan rates. These data were displayed against the scan speeds and the change in current density until a straight line was obtained. The double layer capacitance (Cdl) was then produced by dividing the slope of the line by two and form the Cdl value Equation (2), ECSA was evaluated by dividing Cdl by the Cs (0.040 mF cm−2) was considered from literature for flat electrodes Equation (3) [Citation35].
(2)
(2)
(3)
(3)
In the current scenario, the overpotential (η) vs logj plot was used to evaluate the Tafel slope of prepared materials’ efficiency for the reaction kinetics. The Tafel slope was measured with Equation (4).
(4)
(4)
In Equation (4), overpotential is represented by η, the charge transfer coefficient is represented by α, the number of electrons taking part in the reaction rate is represented by n, the Faraday constant is represented by F, the current density and the exchange current density shows represented by j and j0.
3. Results and discussion
3.1. Morphological, elemental and textural analysis
SEM analysis was employed to examine the surface morphology and particle size of material created hydrothermally. (a–e) displays SEM micrographs of all the synthesized materials at various magnifications. The resultant NiO/NF nanoparticles were cubic ((a,b)), and the shape of the CoSe2wasnanopetals, as displayed in (c). (d,e) depicts the NiO/CoSe2/NF nanohybrid as consisting of vertically growing nanopetals adorned with cubes on their surfaces and resembling vertically oriented nanosheet networks. The resultant outcome strongly supports the particle size between 12 and 25 nm calculated via Imagej software and decorated homogeneously over the nanopetals surface. The nanosheet-like hierarchical porous structure may increase the reactive sites for electrolyte interaction with electrode material which may cause the improvement of active electrode material for OER in 1.0M KOH electrolyte. Furthermore, this morphology has provided a more channel for electron transformation and resulted in improved electrochemical performances.
Figure 1. SEM micrographs of (a,b) NiO icy cubes, CoSe2 nanopetals and (c-d) NiO/CoSe2vertically oriented nanosheet networks at low and high magnification.
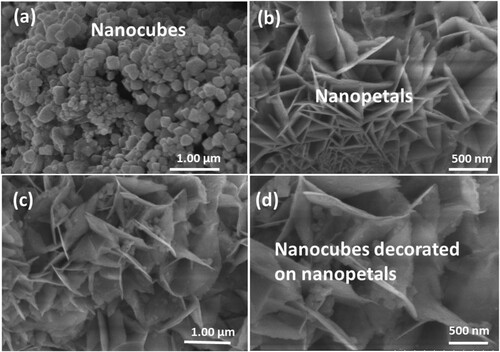
Energy dispersive spectrum (EDS) of the NiO/CoSe2/NF is shown in (a). EDS results affirmed the presence of all the corresponding elements, and their molar compositions are tabulated in calculated via Equation (4).
(4)
(4)
The Brunauer–Emmett–Teller (BET) result of NiO/CoSe2/NF is shown in (b). N2 adsorption/desorption isotherm of the hierarchical nanohybrid NiO/CoSe2/NF displays the hysteresis loop (type II) as depicted in (b). The measured BET interface area, pore size, and pore volume are 65.5 m2 g−1, 17.38 nm, and 0.28 cm3 g−1, respectively. (c) displays the elemental mapping resulting from energy-filtered SEM, displaying the Se, Ni, Co, and O elements all were homogeneously dispersed on the interfaces of nanohybrid, determine the synthesis of NiO/CoSe2/NF. (d) represents the selected area from the EDS analysis, further confirming the successful formation of NiO/CoSe2/NF nanohybrid.
Figure 2. (a) EDX spectrum, (b) BET isotherm, (c) elemental mapping, (d) selected area from EDX analysis of NiO/CoSe2vertically oriented nanosheet networks.
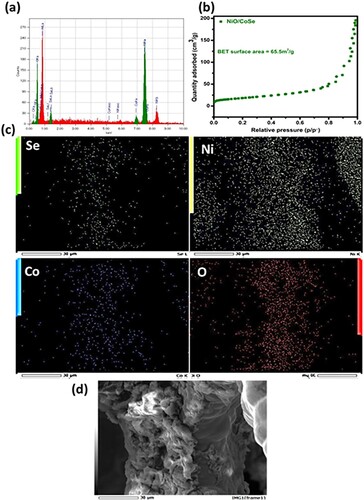
Table 1. Elemental composition resulted from EDX for NiO/CoSe2/NF.
3.2. Structural analysis
XRD technique was exploited to investigate the structure and phases of as-grown products. The traced XRD pattern of CoSe2/NF, NiO/NF, and NiO/CoSe2/NF are illustrated in . The noticeable diffraction peaks of pristine CoSe2 and NiO are consistent with standard data JCPDS card No. 00-001-1239, 00-001-1239, respectively. The diffraction pattern of NiO/CoSe2/NF nanohybrid is perfectively matched with CoSe2/NF cubic structure, and NiO/NF cubic structure validated the growth of the binary component system. The characteristics peaks of pristine CoSe2/NF were observed at 30.7°, 44.82°, and 52.14° which corresponds to a plane (200), (220), and (311), respectively, NiO/NF observed at 37.06°, 43.14°, 62.8°, 75.32°, and 79.5° which consigned to (111), (200), (220), (311), and (222) crystal planes, while in NiO/CoSe2/NF nanohybrid the peaks at 30.58°, 44.74°, and 52.1° consistent with CoSe2 and 37.26°, 43.3°, 62.89°, 75.32°, and 79.48° with NiO/NF, corresponds to planes as mentioned above. There was no additional peak observed in XRD pattern, which endorsed the successful synthesis of the material with excellent crystallinity and purity. Furthermore, the structural changes in NiO/CoSe2/NF nanohybrid due to the interaction of individual components were investigated by calculating average crystalline size, lattice constants, micro-strain, d-spacing, dislocation density, and unit cell volume, using equations discussed in the previous report [Citation36], and values are summarized in . The variation in lattice parameters in composite occurred due to the interaction of different ions, which affect each lattice’s growth. Also, the crystalline size of CoSe2/NF decreased in the composite while NiO/NF increased, which was also confirmed by the sharpness of peaks in the composite.
Table 2. Structural parameters of as-prepared materials.
3.3 Electrochemical OER studies
Electrochemical study for OER was performed via the traditional three-electrode system in basic solution at pH = 13.6. The efficiency of the fabricated electrode material was measured by ECSA, which was linearly proportional to the Cdl. The resultant Cdl values were calculated from cyclic voltammetry (CV) considering the non-redox region at various sweeping rates (5–30 mVs−1) and were displayed in (a–c). The calculated Cdl values for NiO/NF, CoSe2/NF, and NiO/CoSe2/NF electrodes were 27.24, 0.5, and 28.04 mF, respectively, as displayed in (a–c). Taking into account the previously reported findings for the flat electrodes, the Cdl values were then divided by the specific capacitance to obtain the ECSA [Citation36,Citation37]. The resultant ECSA for NiO/NF, CoSe2/NF and NiO/CoSe2/NF electrocatalyst were 681, 13.5 and 701 cm2, respectively. Larger the value of ECSA will be better the OER performance of the electrocatalyst. Hence, the improved ECSA could facilitate the higher OER activity of the electrocatalysts because of the many active zone present on the interfaces of the nanohybrid electrocatalyst.
Figure 4. (a-c) CV curves at various scanning rate, (a′-c′) double layer capacitance for NiO/NF, CoSe2/NF and NiO/CoSe2/NF, respectively.
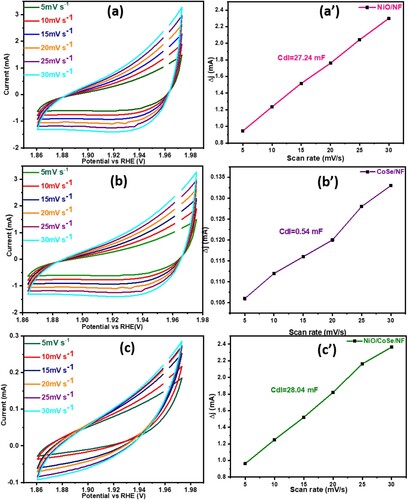
Cyclic voltammetry (CV) and Linear sweep voltammetry (LSV) curves of CoSe2/NF, NiO/ NF, and NiO/CoSe2/NF 3D nanosheet network in 1.0MKOH electrolyte were displayed in (a,b) at 0–2 V vs. RHE at a sweeping speed of 5 mVs−1. Voltammograms represent the two redox peaks revealing the pseudocapacitive behaviour for all synthesized electrodes [Citation38,Citation39]. Among all electrocatalysts, 3D nanocomposite shows higher redox reactions indicating rapid electron transfer due to higher surface area and compact nanosheet network morphology. Ni/Co represents the synthesized product’s active site and the OH. are physically bounds on the active zone and produce Ni/Co-OH in the first step as a result of the oxidation of hydroxide anions (1e-) (Ni/Co-OH). After the linked protons and electrons in Ni/Co-OH are removed, Ni/Co–O is formed. The O- Ni/Co is nucleophilically coupled with 1e- to generate the intermediate M-OH (hydroxyl anion) in contrast to this nucleophilic assault (Ni/Co -OOH). Another e- transfer coupled with a proton released oxygen and produced additional free active centres. Following that follows the direct production of metal (Ni/Co) and oxygen (O2) by combining the two Ni/Co -O species (O2). Since the amount of protons or electrons carried at each step vary, the process as described here can be used as a model for the majority of other processes. Decoupled electron flow was the most popular theory. The possible redox reactions mechanism in alkaline media for CoSe2/NF and NiO/NF were given below as in previously reported results as discussed below [Citation40,Citation41];
(5)
(5)
(6)
(6)
(7)
(7)
(8)
(8)
(9)
(9)
(10)
(10)
(11)
(11)
Figure 5. (a) CV plots at a scan rate of 5 mV s−1, (b) LSV curves, (c) tafel slope and (d) Nyquist plot for NiO/NF, CoSe2/NF and NiO/CoSe2/NF.
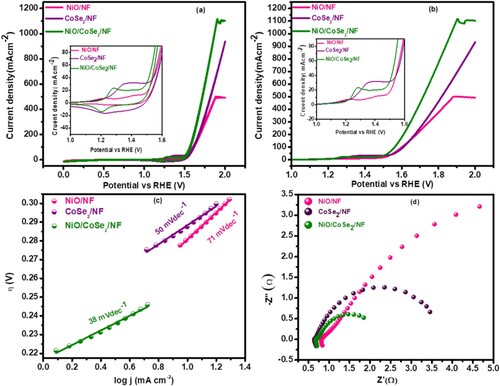
The formation of Co-OOH/NiOOH or Co/Ni-OH is a vital step in making O2, and the formation of oxides is more stable than hydroxides. From comparison , it is seen that the nanohybrid material (NiO/CoSe2) displays remarkably reduced overpotential 263 mV at 10 mAcm−2 comparatively to the counterparts such as NiO/NF (296 mV), CoSe2/NF (289 mV). The lower over the potential of the nanosheet composite, when compared with the pristine NiO and CoSe2, implies the superior OER performance in 1.0 M potassium hydroxide solution at ambient temperature. This increase in oxygen evolution was due to higher conductivity, intrinsic activity, rich in oxygen, and active site on the 3D nanosheet network morphology and synergetic effect, which allows the electronic structure to weak hydrogen adsorption and water dissociation. Furthermore, because the bonding of Selenium with different transition metals results in various electronic structures and atomic arrangements, the electrocatalytic activity could be enhanced due to the synergistic effect.
Furthermore, the Tafel study was investigated for the kinetic mechanism of OER activity and was obtained by the plot of logarithm current density vs. potential (RHE). The NiO/ CoSe2exhibits a lower Tafel plot (38mVdec−1) as compared to the CoSe2/NF (50mVdec−1) and NiO/NF (71mVdec−1). The slightest Tafel slope of the composite indicates the transfer of 4 electrons generated during OER reaction as already reported [Citation37]. The value of the Tafel slope suggests fast kinetics and rapid OER reaction, which would result from 3D nanosheet network structured morphology and good synergistic effect between CoSe2 nano pedals and NiO providing more active sites.
Table 3. Comparison of electrochemical properties for all the synthesized products.
To investigate ions’ transportation, the electrochemical impedance spectroscopy (EIS) analysis was accomplished in similar alkaline conditions using computer control potentiostat. All the synthesized samples represent the semicircle pattern in the small-frequency zone and the resultant semi-arc diameter responds to the electron transfer reaction. The shorter the semicircle, the lower the Rct and higher conductivity due to the lower Rct. Notably, at the electrolyte/electrode interface, the rate of electrons transfer was decided by the value of charge transfer resistance (Rct). Among all the materials, the synthesized nanocomposite NiO/CoSe2has a much lower charge transfer, which benefits the electrochemical reaction process. The resultant EIS spectra were then fitted to the equivalent circuit to get charge transfer resistance(Rct) and solution resistance(Rs) values summarized in .
The electrochemical results revealed that nanohybrid could be employed in the industrial field as an electrocatalyst for hydrogen production to reduce the energy crisis. Hence, the combination of CoSe2 and NiO might enhance surface property. On the other hand, the main activity of CoSe2 and NiO may be due to inactiveness and poor conductivity. In contrast, the nanohybrid may have surface-tailored features with great active sites that allow electron transfer for energy conversion appliance. Due to the above-mentioned results of the nanohybrid, we perform the durability of the nanomaterial. The durability and stability of electrocatalyst by applying the continuous CV cycles in 1.0 M potassium hydroxide electrolyte in the region of (0–2 V vs. RHE) as shown in (a). The obtained results show that the desired material is durable and acceptable due to a negligible shift in the current density for OER performance, and all electrochemical results are compared to the results that have already been published and are listed in . Hence the observed results made the material quite desirable and efficient for OER.
Table 4. Comparative study with other reported electrocatalysts.
In summary, NiO/CoSe2/NF 3-D nanosheet network was achieved by facile in hydrothermal reaction route directly grown on NF. NiO/CoSe2/NF nanocomposite, NiO/NF, and CoSe2/NF domains form plentiful interface zone with excellent chemisorption capacity for oxygen evolution reaction. Additionally, the 3-D NiO/CoSe2/NF nanosheet grown on NF is equally builta3-D interconnected integration structure, permitting the uncovered heterointerfaces. The remarkably lower overpotential of 263 mV, Tafel slope value of 38mVdec-1, and excellent durability and stability of 1500 cycles of NiO/CoSe2/NF make it a promising electrocatalyst in basic medium (1.0M KOH).
Disclosure statement
No potential conflict of interest was reported by the author(s).
Correction Statement
This article has been republished with minor changes. These changes do not impact the academic content of the article.
Additional information
Funding
References
- Gray HB. Powering the planet with solar fuel. Nat Chem. 2009;1(1):7–7.
- Peng L, Shen J, Zheng X, et al. Rationally design of monometallic NiO-Ni3S2/NF heteronanosheets as bifunctional electrocatalysts for overall water splitting. J Catal. 2019;369:345–351.
- Nisa MU, Manzoor S, Abid AG, et al. Cdse supported SnO2 nanocomposite with strongly hydrophilic surface for enhanced overall water splitting. Fuel. 2022;321:124086.
- Jiao Y, Zheng Y, Jaroniec M, et al. Design of electrocatalysts for oxygen-and hydrogen-involving energy conversion reactions. Chem Soc Rev. 2015;44(8):2060–2086.
- Zhang SL, Guan BY, Lu XF, et al. Metal atom-doped Co3O4 hierarchical nanoplates for electrocatalytic oxygen evolution. Adv Mater. 2020;32(31):2002235.
- Katubi KM, Nisa MU, Manzoor S, et al. Hydrothermally fabricated NdTe hollow shells thermally vaporized on Ni foam for water-splitting in alkaline media. Appl Phys A. 2022;128(10):1–10.
- Gu L-F, Chen JJ, Zhou T, et al. Engineering cobalt oxide by interfaces and pore architectures for enhanced electrocatalytic performance for overall water splitting. Nanoscale. 2020;12(20):11201–11208.
- Dou Y, He CT, Zhang L, et al. Approaching the activity limit of CoSe2 for oxygen evolution via Fe doping and co vacancy. Nat commun. 2020;11(1):1–9.
- Zheng S, Chen H, Tong X, et al. Integration of a photo-fenton reaction and a membrane filtration using CS/PAN@ FeOOH/g-C3N4Electrospun nanofibers: synthesis, characterization, self-cleaning performance and mechanism. Appl Catal B: Environ. 2021;281:119519.
- Subbaraman R, Tripkovic D, Chang K-C, et al. Trends in activity for the water electrolyser reactions on 3 d M (Ni, Co, Fe, Mn) hydr (oxy) oxide catalysts. Nat Mater. 2012;11(6):550–557.
- Patil SA, Rabani I, Vikraman D, et al. Template-free synthesis of one-dimensional cobalt sulfide nanorod array as an attractive architecture for overall water splitting. Int J Energy Res. 2021;45(2):2785–2796.
- Rabani I, Hussain S, Vikraman D, et al. 1D-CoSe 2 nanoarray: a designed structure for efficient hydrogen evolution and symmetric supercapacitor characteristics. Dalton Trans. 2020;49(40):14191–14200.
- Li T, Niu K, Yang M, et al. Ultrathin CoS2 shells anchored on Co3O4 nanoneedles for efficient hydrogen evolution electrocatalysis. J Power Sources. 2017;356:89–96.
- Shrestha NK, Patil SA, Cho S, et al. Cu–Fe–NH 2 based metal–organic framework nanosheets via drop-casting for highly efficient oxygen evolution catalysts durable at ultrahigh currents. J Mater Chem A. 2020;8(46):24408–24418.
- Shrestha NK, Patil SA, Han J, et al. Chemical etching induced microporous nickel backbones decorated with metallic Fe@ hydroxide nanocatalysts: an efficient and sustainable OER anode toward industrial alkaline water-splitting. J Mater Chem A. 2022;10(16):8989–9000.
- Tang C, Cheng N, Pu Z, et al. Nise nanowire film supported on nickel foam: an efficient and stable 3D bifunctional electrode for full water splitting. Angew Chem. 2015;127(32):9483–9487.
- Qayoom Mugheri, Tahira A, Aftab U, et al. Co3O4/NiO bifunctional electrocatalyst for water splitting. Electrochim Acta. 2019;306:9–17.
- Prabhu S, Sohila S, Navaneethan D, et al. Three dimensional flower-like CuO/Co3O4/r-GO heterostructure for high-performance asymmetric supercapacitors. J Alloys Compd. 2020;846:156439.
- Ju H, Liu XD, Tao CY, et al. A novel edge-rich structure of CuO/Co3O4 derived from prussian blue analogue as a high-rate and ultra-stable electrode for efficient capacitive storage. Electrochim Acta. 2021;366:137410.
- Ramasamy K, Gupta RK, Palchoudhury S, et al. Layer-Structured copper antimony chalcogenides (CuSbSe x S2–x): stable electrode materials for supercapacitors. Chem Mater. 2015;27(1):379–386.
- Manikandan R, Justin Raj C., Nagaraju G, et al. Selenium enriched hybrid metal chalcogenides with enhanced redox kinetics for high-energy density supercapacitors. Chem Eng J. 2021;414:128924.
- Zhao Z, Liu H, Gao W, et al. Surface-engineered PtNi-O nanostructure with record-high performance for electrocatalytic hydrogen evolution reaction. J Am Chem Soc. 2018;140(29):9046–9050.
- Anantharaj S, Ede SR, Sakthikumar K, et al. Recent trends and perspectives in electrochemical water splitting with an emphasis on sulfide, selenide, and phosphide catalysts of Fe, Co, and Ni: a review. ACS Catal. 2016;6(12):8069–8097.
- Peng X, Yujiao Y, Xun J, et al. Recent advance and prospectives of electrocatalysts based on transition metal selenides for efficient water splitting. Nano Energy. 2020;78:105234.
- Gao R, Zhang H, Yan D. Iron diselenide nanoplatelets: stable and efficient water-electrolysis catalysts. Nano Energy. 2017;31:90–95.
- Luo R, Qian Z, Xing L, et al. Re-looking into the active moieties of metal X-ides (X- = phosph-, sulf-, nitr-, and carb-) toward oxygen evolution reaction. Adv Func Mater. 2021;31(37):2102918.
- Zhao S, Yang Y, Tang ZJAC. Insight into structural evolution, active sites, and stability of heterogeneous electrocatalysts. Angew Chem. 2021;134:e202110186.
- Yu Z, Li Y, Martin-Diaconescu V, et al. Highly efficient and stable saline water electrolysis enabled by self-supported nickel-iron phosphosulfide nanotubes with heterointerfaces and under-coordinated metal active sites. Adv Funct Mater. 2022;32:2206138.
- Yuan H, Wang S, Ma Z, et al. Oxygen vacancies engineered self-supported B doped Co3O4 nanowires as an efficient multifunctional catalyst for electrochemical water splitting and hydrolysis of sodium borohydride. Chem Eng J. 2021;404:126474.
- Liu Y, Cheng H, Lyu M, et al. Low overpotential in vacancy-rich ultrathin CoSe2 nanosheets for water oxidation. J Am Chem Soc. 2014;136(44):15670–15675.
- Kong D, Wang H, Cha JJ., et al. Synthesis of MoS2 and MoSe2 films with vertically aligned layers. Nano Lett. 2013;13(3):1341–1347.
- Long X, Li G, Wang Z, et al. Metallic iron–nickel sulfide ultrathin nanosheets as a highly active electrocatalyst for hydrogen evolution reaction in acidic media. J Am Chem Soc. 2015;137(37):11900–11903.
- Wu R, Zhang J, Shi Y, et al. Metallic WO2–carbon mesoporous nanowires as highly efficient electrocatalysts for hydrogen evolution reaction. J Am Chem Soc. 2015;137(22):6983–6986.
- Lukowski MA, Daniel AS, Meng F, et al. Enhanced hydrogen evolution catalysis from chemically exfoliated metallic MoS2 nanosheets. J Am Chem Soc. 2013;135(28):10274–10277.
- Nazar N, Manzoor S, ur Rehman Y, et al. Metal-organic framework derived CeO2/C nanorod arrays directly grown on nickel foam as a highly efficient electrocatalyst for OER. Fuel. 2022;307:121823.
- Manzoor S, Ashiq MF, Usman M, et al. Development of excellent and novel flowery zirconia/cadmium sulfide nanohybrid electrode: For high performance electrochemical supercapacitor application. J Energy Storage. 2021;40:102718.
- Aftab F, Duran H, Kirchhoff K, et al. A facile synthesis of FeCo nanoparticles encapsulated in hierarchical N-doped carbon nanotube/nanofiber hybrids for overall water splitting. Welcome to ICONN-2019. 2020: 35.
- Yue Z, Zhu W, Li Y, et al. Surface engineering of a nickel oxide–nickel hybrid nanoarray as a versatile catalyst for both superior water and urea oxidation. Inorg Chem. 2018;57(8):4693–4698.
- Jin Y, Huang S, Yue X, et al. Mo-and Fe-modified Ni (OH) 2/NiOOH nanosheets as highly active and stable electrocatalysts for oxygen evolution reaction. ACS Catal. 2018;8(3):2359–2363.
- Dinh KN, Gomes VG. Hybrid Ni/NiO composite with N-doped activated carbon from waste cauliflower leaves: A sustainable bifunctional electrocatalyst for efficient water splitting. Carbon. 2020;157:515–524.
- Prabakaran K, Lokanathan M, Kakade B. Three dimensional flower like cobalt sulfide (CoS)/functionalized MWCNT composite catalyst for efficient oxygen evolution reactions. Appl Surf Sci. 2019;466:830–836.
- Liang D, et al. In-situ doping of Co in nickel selenide nanoflower for robust electrocatalysis towards oxygen evolution. Int J Hydrogen Energy. 2020;45(51):27047–27055.
- Zhou Q, Li TT, Qian J, et al. Self-supported hierarchical CuO x@ Co 3 O 4 heterostructures as efficient bifunctional electrocatalysts for water splitting. J Mater Chem A. 2018;6(29):14431–14439.
- Dong Y, Yang J, Liu Y, et al. 2D Fe-doped NiO nanosheets with grain boundary defects for the advanced oxygen evolution reaction. Dalton Trans. 2020;49(19):6355–6362.
- Dai W, Lu T, Pan YJJoPS. Novel and promising electrocatalyst for oxygen evolution reaction based on MnFeCoNi high entropy alloy. J Power Sources. 2019;430:104–111.
- Zhao L, Cao Q, Wang A, et al. Iron oxide embedded titania nanowires–An active and stable electrocatalyst for oxygen evolution in acidic media. Nano Energy. 2018;45:118–126.
- Fominykh K, Chernev P, Zaharieva I, et al. Iron-doped nickel oxide nanocrystals as highly efficient electrocatalysts for alkaline water splitting. ACS Nano. 2015;9(5):5180–5188.
- Wang C, Qi L. Heterostructured inter-doped Ruthenium–Cobalt oxide hollow nanosheet arrays for highly efficient overall water splitting. Angew Chem. 2020;132(39):17372–17377.
- Yan K, Lu YJS. Direct growth of MoS2 microspheres on Ni foam as a hybrid nanocomposite efficient for oxygen evolution reaction. Small. 2016;12(22):2975–2981.
- Li W, Gao X, Xiong D, et al. Hydrothermal synthesis of monolithic Co3Se4 nanowire electrodes for oxygen evolution and overall water splitting with high efficiency and extraordinary catalytic stability. Adv Energy Mater. 2017;7(17):1602579.