ABSTRACT
Introduction
Development of new antimicrobials with ever ‘better’ bacterial killing has long been considered the appropriate response to the growing threat of antimicrobial-resistant infections. However, the time-period between the introduction of a new antibiotic and the appearance of resistance amongst bacterial pathogens is getting shorter and shorter. This suggests that alternative pathways than making ever ‘better’ antimicrobials should be taken.
Areas covered
This review aims to answer the questions (1) whether we have means to circumvent existing antibiotic-resistance mechanisms, (2) whether we can revert existing antibiotic-resistance, (3) how we can prevent the development of antimicrobial-resistance against novel infection-control strategies, including nano-antimicrobials.
Expert opinion
Relying on relieving antibiotic-pressure and natural outcompeting of antimicrobial-resistant bacteria seems an uncertain way out of the antibiotic-crisis facing us. Novel, non-antibiotic, nanotechnology-based infection control-strategies are promising. At the same time, rapid development of new resistance mechanisms once novel strategies is taken into global clinical use, may not be ruled out and must be closely monitored. This suggests focusing research and development on designing suitable combinations of existing antibiotics with new nano-antimicrobials in a way that induction of new antimicrobial-resistance mechanisms is avoided. The latter suggestion, however, requires a change of focus in research and development.
1. Introduction
The excessive use of antibiotics drives the evolution of antibiotic-resistant bacteria [Citation1] and forms an increasing threat to our ability to treat bacterial infection [Citation2,Citation3]. Antimicrobial-resistant bacterial infections increase morbidity and mortality, inflict high costs to the healthcare system, cause major economic losses [Citation4–Citation6] and are predicted to become the number one cause of death by the year 2050 () [Citation7–Citation9].
Figure 1. Predictions for the year 2050 on the number of deaths caused by various diseases, including antimicrobial-resistant diseases. (From: review on antimicrobial resistance. Tackling drug-resistant infections globally: final report and recommendations. Available online: https://amr-review.org/sites/default/files/160518_Final%20paper_with%20cover.pdf (accessed on 27 December 2019)) (with permission of Wellcome collection, Attribution 4.0 International (CC BY 4.0)).
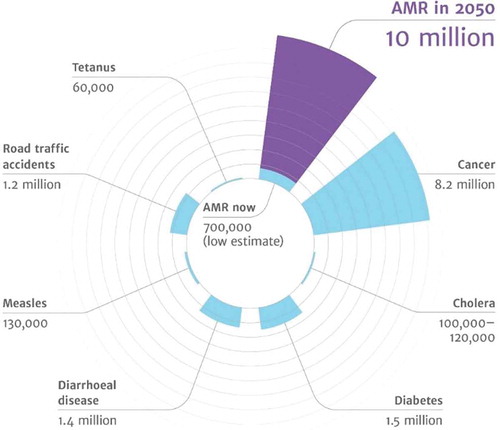
Whereas the development of new, ever ‘better’ antimicrobials has long been considered the right pathway to go in order to prevent this sour prediction from becoming true, development of novel antibiotics, their regulatory approval, and downward clinical translation proceed at a slow pace [Citation10]. Industry is gradually losing interest in the development of novel antibiotics focusing on the development of drugs for other diseases with a rapid return of investment [Citation11], since their active lifetime before the first resistant bacterial strains appear, becomes shorter and shorter [Citation12,Citation13] and return of investment is becoming more and more doubtful [Citation14].
With respect to novel infection-control strategies, expectation from nanotechnological innovations is high. Therefore, this review first briefly summarizes novel nanotechnology-based infection-control strategies and subsequently focuses on mechanisms of antimicrobial resistance in order to answer the questions: (1) do we have means to circumvent existing antibiotic-resistance mechanisms, (2) can we revert existing antibiotic-resistance, (3) how can we prevent the development of antimicrobial resistance, particularly against novel infection-control strategies.
2. Emerging new infection-control strategies
Currently, the focus in the development of new infection-control strategies is on novel, nanotechnology-based strategies [Citation15]. Nanotechnology is producing an ongoing, high number of highly diverse, novel nano-antimicrobials [Citation16] that are arguably providing already more new solutions than needed. Many novel, nanotechnological infection-control strategies are based on earlier-introduced tumor-control strategies [Citation17]. Unfortunately, with a predominantly academic motivation, there are few attempts made to bring these novel infection-control strategies to clinical translation [Citation10,Citation18]. Different nanotechnology-based antimicrobials are summarized in . The common feature of nano-antimicrobials is their small size and surface-adaptability that allow them to penetrate into an infectious biofilm. Many currently employed antimicrobials have difficulty penetrating infectious biofilms due to the extracellular matrix, self-produced by different bacterial strains. The extracellular matrix protects biofilm inhabitants against the host immune system and antimicrobial attack [Citation19,Citation20]. For penetration across the entire thickness of infectious biofilms (usually ranging up to 50 µm [Citation21]), antimicrobials should be able to be transported through water-filled channels existing in biofilms [Citation22–Citation24] without adsorption to the channel walls [Citation25]. Also, nano-antimicrobials should resist reticuloendothelial rejection during transport through the blood circulation [Citation26,Citation27]. This limits their effective size to between 100 and 200 nm [Citation28]. All nano-antimicrobials listed in can be prepared to meet this criterion, with carbon dots being the smallest type of nano-antimicrobials [Citation29,Citation30].
Figure 2. Overview of novel, nanotechnology-based antimicrobials, distinguishing organic and inorganic materials, together with their perceived advantages with respect to infection-control.
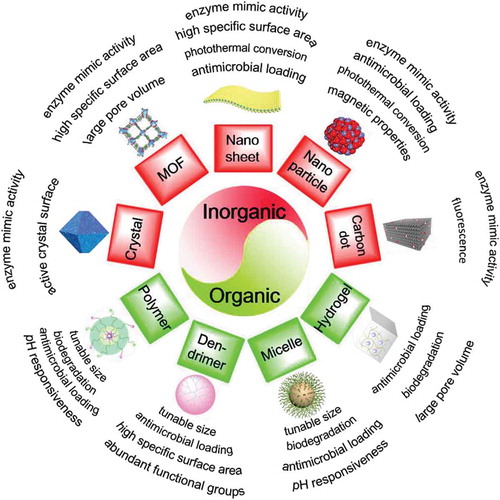
Nano-antimicrobials can be separated into two classes, based on whether composed of inorganic or organic components. Some inorganic nano-antimicrobials possess enzyme-mimic activity, like peroxidase- or deoxyribonuclease-like activity. Peroxidase-activity of inorganic nanoparticles, including carbon dots and metals included in nanocrystals, allows conversion of H2O2 into reactive-oxygen species (ROS). ROS is lethal to most bacterial strains and species [Citation31–Citation33], but as a drawback many ROS species are highly unstable and short-lived (usually in the micro- to milli-seconds range) [Citation34]. Other reactive-nitrogen species generated by nanoparticles such as nitric oxide (NO) are more stable than ROS [Citation35], killing bacteria through inhibition of DNA replication or blocking their respiration [Citation36], and hindering adhesion [Citation37]. Deoxyribonuclease-like activity can cause hydrolysis of extracellular DNA (eDNA) [Citation38], an essential component of the extracellular matrix that keeps a biofilm together [Citation39]. Disruption of the biofilm matrix may make a biofilm more amenable to antimicrobial treatment [Citation40] and bacterial dispersal [Citation41] into the bloodstream, after which planktonic, infectious bacteria are more prone to killing by antibiotics or host immune cells [Citation42].
Inorganic nano-antimicrobials introduce two new features in infection-control, being their composition-dependent photothermal and magnetic properties. Photothermal nanoparticles can be locally light-activated to produce high amounts of heat and have been studied mostly for tumor control [Citation43,Citation44], but heat can kill both mammalian cells and bacteria. Infectious bacteria can be killed using photothermal nanoparticles, presumably irrespective of Gram-character, strain, or species (see ) for an example involving Staphylococcus epidermidis) [Citation45–Citation47]. Upon application of photothermal nanoparticles in the human body to kill infectious bacteria, heat can dissipate to surrounding tissues to cause collateral damage [Citation48]. Therefore, adequate targeting of photothermal nanoparticles to a micrometer-sized, infectious site is important, but this is usually much more difficult than targeting to a centimeter-sized tumor upon which their initial clinical use was based [Citation43,Citation44]. A similar difficulty is associated with the use of antimicrobial magnetic nanoparticles [Citation49]. Whereas targeting of anti-tumor magnetic nanoparticles to a tumor site is possible by a variety of methods [Citation50,Citation51], exact targeting to a micrometer-sized, infectious biofilm and distribution over the entire depth of a biofilm is far from trivial [Citation49], opposite to the ideal distribution ()) that is suggested to be achieved effortlessly in many articles on the use of magnetic nanoparticles for infection-control [Citation52,Citation53]. In reality, over- or under-targeting of magnetic nano-particles toward the top or bottom of an infectious biofilm readily occurs, unless magnetic field strength and application time are well tuned to the dimensions of the biofilm [Citation49]. As an alternative to the exact targeting of magnetic nanoparticles, magnetic iron-oxide nanoparticles have been magnetically pulled through infectious biofilms using a simple external magnetic field to dig artificial channels that increase antibiotic penetration and bacterial killing [Citation22,Citation49,Citation54].
Figure 3. Examples of the advantages of nano-antimicrobials in combating infectious biofilms. (a) Fluorescence images showing the effect of a gold-nanorod coating on a glass surface in bacterial killing of an S. epidermidis biofilm by photothermal heat conversion. Biofilms on the gold-nanorod coated surface show almost complete bacterial killing (red-fluorescent bacteria) upon NIR-irradiation. Green-fluorescent bacteria are alive [Citation47] (with the permission of Elsevier Ltd.). (b) The idealized deep penetration and homogeneous distribution of magnetically targeted nanoparticles into infectious biofilms often assumed rather than experimentally demonstrated and not trivial to achieve [Citation49] (with permission of ACS Publications). (c) Penetration and accumulation of pH-responsive, Nile-red-loaded mixed-shell polymeric micelles in a staphylococcal biofilm at pH (5.0) [Citation64] (with permission of ACS Publications). (d) ROS production by graphene carbon dots (GQDs) upon sunlight irradiation (quantified by DCFH-DA staining) and E. coli survival (inset) [Citation30] (with permission from Taylor & Francis).
![Figure 3. Examples of the advantages of nano-antimicrobials in combating infectious biofilms. (a) Fluorescence images showing the effect of a gold-nanorod coating on a glass surface in bacterial killing of an S. epidermidis biofilm by photothermal heat conversion. Biofilms on the gold-nanorod coated surface show almost complete bacterial killing (red-fluorescent bacteria) upon NIR-irradiation. Green-fluorescent bacteria are alive [Citation47] (with the permission of Elsevier Ltd.). (b) The idealized deep penetration and homogeneous distribution of magnetically targeted nanoparticles into infectious biofilms often assumed rather than experimentally demonstrated and not trivial to achieve [Citation49] (with permission of ACS Publications). (c) Penetration and accumulation of pH-responsive, Nile-red-loaded mixed-shell polymeric micelles in a staphylococcal biofilm at pH (5.0) [Citation64] (with permission of ACS Publications). (d) ROS production by graphene carbon dots (GQDs) upon sunlight irradiation (quantified by DCFH-DA staining) and E. coli survival (inset) [Citation30] (with permission from Taylor & Francis).](/cms/asset/768bf56b-99d4-4279-9b0a-3435a7387911/iedd_a_1779697_f0003_oc.jpg)
Several nano-antimicrobials, due to their enormous specific surface area, can release high numbers of anti-bacterial metal ions, such as Ag ions [Citation55]. Large specific surface areas, such as of graphene oxide sheets also yield the possibility to bind high concentrations of antibiotics to serve as a delivery vehicle and promote the killing of bacteria [Citation56]. However, the specific structure of many inorganic nano-antimicrobials can also do irreparable and lethal physical damage to infectious bacteria on its own. The knife-like edges of two-dimensional nanomaterials can insert into bacterial membranes to extract phospholipids and therewith cause lethal cell wall damage [Citation57]. Finally, the open pore structure of metal-organic frameworks (MOFs) offers many possibilities for antimicrobial-loading and subsequent release of gases or nanozymes.
Organic nano-antimicrobials offer similar size advantages as inorganic nano-antimicrobials, while their size is often tunable [Citation58]. In addition, organic nano-antimicrobials are often biodegradable. Chitosan, for instance, is a biodegradable and nontoxic natural organic polymeric material [Citation59] and its cationic groups can interact with negatively charged bacteria to change the permeability of the bacterial cell membrane, bind to intracellular DNA, and inhibit mRNA transcription and protein synthesis [Citation60]. Aloe vera, as a natural herbal antibacterial agent, can effectively inhibit the growth of Staphylococcus aureus and Escherichia coli [Citation61]. The biodegradable polymer composite film-coating formed by Aloe vera and polyvinyl alcohol has the potential ability to prevent surgical site infection and promote wound healing [Citation62].
Organic nano-antimicrobials are relatively easy to modify as they can possess a large variety of functional groups that can be used to alter their interaction with their environment, i.e. blood, saliva, tissue cells, or biofilm bacteria and their protective matrix. pH-responsive antimicrobial-loaded micelles composed of a hydrophobic polycaprolactone (PCL) core and a hydrophilic poly(ethylene glycol) (PEG) corona can circumvent clearance by the reticuloendothelial system and be stealthily transported through the blood circulation [Citation63] to deliver an antimicrobial cargo in the acidic environment of a biofilm. pH-responsiveness typically inverts the charge of micellar nanocarriers from being negatively charged at physiological pH to being positively charged at pH 5.0, as usually prevailing in infectious biofilms [Citation64]. This allows antimicrobial-loaded micelles to penetrate and accumulate in biofilms (). Depending on micellar properties, antimicrobial release can be triggered by bacterial enzymes to kill bacteria over the depth of a biofilm [Citation64].
Zwitterionic polymeric nanoparticles possess an equimolar number of anionic and cationic groups along the same or different polymer chain [Citation65] and therewith also can alter their charge properties under physiological conditions and inside an acidic, infectious biofilm in a pH-responsive manner [Citation66,Citation67].
Carbon dots can be made from a variety of sources by simple carbonization [Citation68–Citation70] and possess antibacterial and antifungal activity [Citation29]. E. coli could be killed by ROS, produced by graphene quantum dots irradiated by sunlight () [Citation30]. Compared with carbon dots, dendrimers possess a precise molecular structure providing a large number of functional groups that can be used for conjugation of antimicrobials [Citation46,Citation71–Citation74].
3. Mechanisms of antibiotic resistance
A proper summary of antimicrobial-resistance should distinguish between the intrinsic antimicrobial-resistance that individual bacteria may possess during planktonic growth and the recalcitrance to antimicrobial penetration offered to bacteria in their protective biofilm-mode of growth, common to the majority of bacterial infections [Citation75]. The biofilm-mode of growth and the barrier it poses toward the penetration of antimicrobials and their problematic killing efficacy across the depth of a biofilm were known long before the word ‘biofilm’ was coined. Antoni van Leeuwenhoek described over three hundred years ago that vinegar, as an ancient antimicrobial, killed only bacteria that resided at the outside of a bacterial layer attached to a surface, i.e. what we now call ‘biofilm’ [Citation76]. Therewith, biofilm formation can be classified as a main mechanism of antibiotic-resistance together with other, intrinsic mechanisms of antibiotic-resistance, including enzymatic inactivation of antimicrobials, target modification, immunity, and bypass, efflux ()).
Figure 4. Mechanisms of antibiotic-resistance and antibiotic targets. (a) Mechanisms of antimicrobial resistance, including biofilm formation and intrinsic mechanisms of antibiotic-resistance-based or natural resistance or resistance acquired through gene mutation of transfer. (B) Bacterial targets of different antibiotics and resistance mechanisms applied by bacterial pathogens [Citation77] (with permission from BioMed Central).
![Figure 4. Mechanisms of antibiotic-resistance and antibiotic targets. (a) Mechanisms of antimicrobial resistance, including biofilm formation and intrinsic mechanisms of antibiotic-resistance-based or natural resistance or resistance acquired through gene mutation of transfer. (B) Bacterial targets of different antibiotics and resistance mechanisms applied by bacterial pathogens [Citation77] (with permission from BioMed Central).](/cms/asset/46d34503-2dcc-407f-9314-4d4fcb46f152/iedd_a_1779697_f0004_oc.jpg)
Clinically, antibiotics form the most applied class of antimicrobials. Antibiotic targets depend on the type of antibiotic and the bacterial strain involved and can range from the cell wall, DNA/RNA synthesis, folate synthesis, the cell membrane, and intracellular protein synthesis ()) [Citation77]. Accordingly, mechanisms of antibiotic resistance applied by bacterial pathogens also differ (see also )), depending on the antibiotic and genetic abilities of the organism. Importantly, bacteria can acquire resistance mechanisms by gene mutation under the influence of environmental antibiotic pressure or gene transfer, particularly in a biofilm.
3.1. Biofilm formation
Biofilms are complex, surface-adhering, and surface-adapted microbial communities within a self-composed extracellular polymeric matrix [Citation78,Citation79], comprised of polysaccharides, proteins, lipids, and extracellular DNA. Matrix production starts upon sensing of the adhesion forces arising from a substratum surface [Citation80] and can differ on surfaces from which different adhesion forces arise. Biofilms of some strains, adhering strongly to hydrophobic silicone rubber, produced an extensive EPS matrix and therewith became less susceptible to gentamicin. However, these strains only weakly adhered to hydrophilic, polymer-brush coated silicone rubber, leaving the bacteria in the impression of being in a planktonic state [Citation81] and accordingly had a similar susceptibility to gentamicin as planktonic bacteria. In a biofilm, depth-dependent gradients exist, like in nutrient availability, pH, and oxygen concentration toward the bottom of a biofilm [Citation82,Citation83], that cause a low metabolic activity, associated with a higher tolerance to antibiotics [Citation82]. These low metabolically active or metabolically inactive bacteria in a biofilm-mode of growth are in a so-called ‘dormant’ state. Dormant bacteria are tolerant to antibiotics, but not resistant, have not genetically changed, but are phenotypic variants.
Persisters can comprise 1% of a biofilm-population [Citation84] but due to their antibiotic-tolerance often related to chronic infections as they can start re-growing when antibiotic pressure is relieved. Antibiotic-resistant bacteria have genetically changed to block antibiotic activity and grow in the presence of antibiotics. Typically, a biofilm houses subpopulations of bacteria that are susceptible and resistant to an antibiotic, a phenomenon called hetero-resistance [Citation85,Citation86]. Accordingly, the crowdedness and close proximity between bacteria in a biofilm-mode of growth create a hot-spot for horizontal gene transfer occurring within and between bacterial species and resulting genetic changes [Citation87]. Horizontal gene transfer requires a membrane-associated DNA transport system as a part of natural, bacterial evolution [Citation88]. Antibiotic-resistant mutants therefore generally appear more frequent in bacteria in a biofilm-mode of growth than during planktonic growth and accordingly inhibitory (MIC) and bactericidal concentrations (MBC), against biofilm bacteria, are much higher than that of planktonic bacteria [Citation89,Citation90].
3.2. Enzymatic inactivation of antimicrobials
Many bacterial strains have the ability to secrete enzymes that protect them against antibiotics or other antimicrobials. Typically, these protective enzymes will add an acetyl or phosphate group to a specific site on the antibiotic to inactivate it [Citation91,Citation92]. Enzymes not only inactivate an antibiotic by blocking a specific molecular site but also by inducing structural changes to an antibiotic molecule [Citation93]. β-lactamase for instance destroyed the core of β-lactam antibiotics to inactivate its active sites (serine residues or Zn2+) [Citation94]. Structural changes and associated inactivation of active sites can prevent antibiotic molecules from binding to a target protein through steric hindrance, therewith causing antibiotic-resistance. Particularly aminoglycosides are rich in hydroxyl and amide groups, that are prone to structural changes by modifying enzymes. Currently, thousands of enzymes have been identified inactivating different classes of antibiotics, including β-lactams, aminoglycosides, macrolides, and rifampicins [Citation77] ()). Synthetic anhydrotetracycline analogues, for instance, are under consideration [Citation95] as a pro-active inhibitor of enzymatic tetracycline degradation in E. coli expressing tetracycline destructase enzymes ()).
Figure 5. (a) In vitro inhibition by different synthetic anhydrotetracycline (aTC) analogues of tetracycline destructase degradation as a function of inhibitor concentration, measured using an optical absorbance kinetic assay and expressed as a velocity parameter [Citation95]. (with permission from Springer). (b) Normalized nsaS expression as a part of the nisin efflux system in S. aureus SH1000 in a planktonic state and adhering to a hydrophobic polyethylene surface [Citation111,Citation115].
![Figure 5. (a) In vitro inhibition by different synthetic anhydrotetracycline (aTC) analogues of tetracycline destructase degradation as a function of inhibitor concentration, measured using an optical absorbance kinetic assay and expressed as a velocity parameter [Citation95]. (with permission from Springer). (b) Normalized nsaS expression as a part of the nisin efflux system in S. aureus SH1000 in a planktonic state and adhering to a hydrophobic polyethylene surface [Citation111,Citation115].](/cms/asset/b45f8010-7a4a-4cc9-bd6e-47d792ad0dbb/iedd_a_1779697_f0005_oc.jpg)
3.3. Target modification
Different antibiotics have different intra- or extra-cellular components as a target to inflict lethal damage to a bacterium ()), including genetic material, proteins, the cytoplasmic membrane, and other cell wall components [Citation96,Citation97]. Antibiotics bind to their targets with high affinity in order to negatively affect the normal functioning of the target. However, upon acquiring antibiotic resistance, target sites can be modified to lower the affinity of an antibiotic for the target site [Citation77,Citation89,Citation98,Citation99]. Resistance in Streptomyces sp. strain AM-2504 has been described to be due to amino acid substitutions in its ribosomes, yielding a loss of affinity for the antibiotic dityromycin, therewith protecting organism [Citation100].
3.4. Immunity and bypass
Our understanding of a nucleic acid-based ‘immunity’ system in bacteria, possibly governed by the CRISPR spacer content and the Cas enzymatic machinery, is still in its infancy [Citation101,Citation102]. Yet, it seems realistic that bacteria can acquire immunity to bypass antibiotics through the production of proteins that bind to antibiotics or their targets to prevent the interaction of an antibiotic with its target site [Citation99,Citation102]. CRISPR/Cas9 or its variants can be used to target bacterial antibiotic-resistance to enhance their susceptibility to current antibiotics. For instance, mutated penicillin-binding protein 2a causes methicillin-resistance in Staphylococcus aureus (MRSA) [Citation103]. A CRISPR/dCas9 plasmid system with multiple target sites can be used to replace the CRISPR-Cas9 system to target mecA gene sites in MRSA, reducing mecA gene expression and making MRSA regain susceptibility to lactam antibiotics [Citation104]. In contrast, the CRISPR/Cas machinery can also be used to impede the transfer of specific nucleic acid sequences into a bacterium which might avoid, via genetic-engineering, the dissemination of antibiotic resistance [Citation105]. However, CRISPR-based immunity in bacteria is mostly associated with resistance against viruses [Citation106].
3.5. Efflux and decreased uptake
Whereas bacterial cell wall membranes themselves present already effective barriers toward antibiotic uptake [Citation107], bacterial membranes can also possess proteinaceous structures to pump out antibiotics that have entered a cell. Accordingly, efflux pumps have been recognized as a major mechanism applied by bacterial strains to prevent killing by antibiotics [Citation108]. Depending on the family of efflux pumps they belong to [Citation109–Citation113], efflux pumps can be activated by chemical or mechanical stresses. In the presence of nisin, the intra-membrane located nisin sensor NsaS in S. aureus donates phosphate groups to an intra-cellularly located response-regulator NsaR to modulate efflux of the antibiotic [Citation114]. Efflux was demonstrated to be more efficient when the intra-membrane sensor received not only a chemical stimulus but also a mechanical one due to adhesion to a surface ()) [Citation115], as in a biofilm.
4. Circumventing existing antibiotic-resistance mechanisms
Circumventing antibiotic-resistance mechanisms may allow us to make longer use of existing antibiotics. Proper encapsulation of existing antibiotics in smart nanocarriers has already addressed the poor penetrability of infectious biofilms by antibiotics, as a mechanism controlling antimicrobial resistance ()) [Citation64,Citation116,Citation117]. Triclosan loaded into smart micellar nanocarriers also led to the killing of multiple-drug-resistant S. aureus [Citation118]. In general, bacteria seem to have more difficulty dealing with attacks by multi-antimicrobials at the same time than by single-antimicrobial attacks [Citation119]. Hence, we here briefly summarize the advantages of combining different antimicrobials with an antibiotic to combat antibiotic-resistant bacteria, including the use of nano-antimicrobials.
4.1. Antibiotic-antibiotic combinations
Infection treatment is considered easier when applying multiple antibiotics at the same time. Combination of antibiotics with different working mechanisms is preferable to increase efficacy and circumvent bacterial resistance, because it is difficult for a bacterium to block different working mechanisms at the same time [Citation120]. More importantly, multiple antibiotic treatment is considered less likely to induce antibiotic-resistance, particularly when the antibiotics have different target sites [Citation121], as this would require multiple mutations in the same bacterium [Citation122]. However, for synergy between two antibiotics to develop, they have to reach an infectious bacterium at the same time [Citation123,Citation124]. A bone cement, used for the fixation of orthopedic joint arthroplasties, for instance, loaded with a combination of gentamicin and fusidic acid was effective against a higher number of clinical isolates, including gentamicin-resistant strains, than gentamicin or clindamycin. Moreover, this combination performed better than the combination of gentamicin and clindamycin, likely because both are amino-glycosides [Citation125]. An evaluation of single-, dual-, and triple-antibiotic combinations comprising 14 different antibiotics (clindamycin, ciprofloxacin, tobramycin, streptomycin, cefoxitin, nitrofurantoin, ampicillin, erythromycin, gentamicin, chloramphenicol, vancomycin, fusidic acid, doxycycline, and trimethoprim) yielded a synergistic increase in efficacy of 17% of triple-antibiotic combinations compared to a 5% increase of dual-antibiotic combinations, as evaluated against two E. coli and an S. epidermidis strain [Citation124].
4.2. Combination of antimicrobial peptides and antibiotics
Often antibiotic-resistant bacteria show sensitivity to antimicrobial peptides [Citation126,Citation127]. Antimicrobial peptides are composed of a cationic group with a hydrophobic tail and possess the ability to perforate bacterial cell membranes to enhance antibiotic entry and killing. Sub-inhibitory concentrations of the antimicrobial polypeptide colistin for instance, clearly demonstrated cell wall perforation in Pseudomonas aeruginosa, E. coli, and Acinetobacter baumanii, yielding a synergistic effect with chloramphenicol, tetracycline, linezolid, and vancomycin against strains resistant to at least one of these antibiotics [Citation128].
4.3. Combination of synthetic antimicrobials and antibiotics
Synthetic antimicrobials comprise a wide variety of compounds, ranging from synthetic antimicrobial peptides, quaternary ammonium compounds to well-known clinically used antimicrobials, such as Triclosan and chlorhexidine [Citation129,Citation130]. Synthetic antimicrobial peptides and quaternary ammonium compounds generally function the same way as antimicrobial peptides and can pave the way for antibiotic entry in a bacterium by perforating the bacterial cell membrane [Citation131]. Amongst a screening of 6,080 compounds for possible synergistic action with tobramycin against P. aeruginosa, Triclosan as an FDA-approved antimicrobial, combined with tobramycin yielded a 2 log-unit reduction in viable bacteria in a biofilm-mode of growth, while neither Triclosan nor tobramycin any significant antimicrobial efficacy against 6/7 cystic fibrosis (CF) clinical isolates, including a tobramycin-resistant strain [Citation132]. However, the application of Triclosan in a wide range of consumer products has been described to induce a dormancy through its inhibition of fatty acid synthesis, yielding antibiotic tolerance, as an unexpected side effect [Citation133].
4.4. Combination of herbal antimicrobials and antibiotics
Several herbal antimicrobials [Citation134,Citation135] have demonstrated antimicrobial activities against multi-drug resistant bacterial strains. Combination of herbal antimicrobials with existing antibiotics lowered the minimum inhibitory concentrations of antibiotics against bacteria [Citation136]. Thymol and carvacrol reduced the resistance of Salmonella typhimurium against ampicillin, tetracycline, penicillin, bacitracin, erythromycin, and novobiocin and resistance of Streptococcus pyogenes to erythromycin. For E. coli thymol and cinnamaldehyde had a reducing effect on the resistance against ampicillin, tetracycline, penicillin, erythromycin, and novobiocin. Carvacrol, thymol, and cinnamaldehyde reduced the resistance of S. aureus against ampicillin, penicillin, and bacitracin [Citation128,Citation136]. These synergistic activities have been ascribed to the ability of herbal antimicrobials to perforate bacterial cell membranes and interfere with enzymatic inactivation of antibiotics [Citation137,Citation138].
4.5. Combination of nano-antimicrobials and antibiotics
There are numerous examples of nanoparticles that circumvent antimicrobial resistance in different bacterial strains and species, including nitric oxide-releasing nanoparticles [Citation139] or hydrogels [Citation140], chitosan [Citation59] and metal [Citation141] (most notably silver) [Citation142] containing nanoparticles, combining different modes of action to eradicate antimicrobial-resistant infections [Citation59]. Vancomycin encapsulated in chitosan nanoparticles killed vancomycin-resistant S. aureus, gold nanoparticles conjugated with vancomycin killed vancomycin-resistant enterococci and E. coli and in combination with ciprofloxacin demonstrated improved killing of vancomycin-resistant enterococci [Citation143].
4.6. Enzyme-inhibitors
Bacterial resistance to β-lactams, the most commonly used class of antibiotics, is predominantly caused by the production of bacterial enzymes, termed β-lactamases [Citation144,Citation145]. Enzymes secreted by bacteria to deactivate antibiotics can be inactivated on their turn, by enzyme-inhibitors [Citation91,Citation94]. Therewith, pro-active co-delivery of antibiotics and enzyme-inhibitors constitutes a powerful pathway to circumvent bacterial resistance to β-lactams. β-lactam antibiotics and β-lactamase inhibitors can be co-delivered for instance, by encapsulation in a metal-organic framework and have been shown to enable the killing of MRSA strains [Citation146].
5. Preventing the development of antimicrobial-resistance
Arguably, the development of novel antimicrobials that do not induce resistance may be equally or even more important than the development of ever ‘better’ antimicrobials. For many forms of antimicrobial resistance to develop, it is required that resistant mutants are generated, but as a next step, a population must be selectively enriched by the resistant sub-population [Citation147]. Methods to study induction of antimicrobial-resistance are relatively scarce and time-consuming but considering their potential future importance will be briefly summarized below, together with infection-control strategies currently known not to induce infection.
5.1. Methods to study induction of antimicrobial resistance
Antimicrobial resistance is usually assessed through the measurement of minimal inhibitory concentrations (MIC). For the phenomenological study of the induction of antimicrobial resistance, bacteria can be transferred from a growing culture into a medium supplemented with a sub-MIC concentration of an antimicrobial for successive, serial-passaging of an aliquot into fresh antimicrobial-supplemented medium. For each serial-passage, the MIC is determined. In some protocols, after a selected number of serial passages, antimicrobial concentrations are doubled repeatedly up to half the MIC. Short-term induction involves less than 20 serial-passages, while in some protocols long-term induction involves up to100 serial-passages. Essentially, once a resistant strain has been obtained, stability of resistance should be tested by culturing the resistant strain in the absence of the antimicrobial for a minimum of 5–10 serial passages [Citation148].
In a more mechanistic approach, the number of mutations conveying resistance to bacteria is determined by enumeration of the number of bacteria that acquire resistance against a sub-MIC concentration of an antimicrobial per viable organism [Citation149]. This is generally referred to as the mutation frequency but bears as a disadvantage that it neglects whether bacteria acquire resistance after short- or long-term exposure to an antimicrobial. For a given exposure time, bacterial survival in the presence of antibiotics starts to decline above MIC ()), attributed to the inhibition of wild-type bacterial growth [Citation150]. The low level-metabolic activity of bacteria between MIC and MBC allows mutations to occur and cause antibiotic resistance. Once an antibiotic concentration equal to MBC is reached within a certain time-window, no CFUs can be retrieved. The so-called mutant prevention concentration (MPC) is therefore slightly lower than MBC, alluding full eradication of all bacteria present. Mutant resistant strains can be selected between MIC and MPC, i.e. the Mutant Selection Window (MSW, see , )) [Citation150,Citation151].
Figure 6. The concepts of mutant selection window (MSW) and mutant prevention concentration (MPC), as introduced by [Citation151]. (a) CFUs retrieved from a bacterial culture after antimicrobial exposure as a function of antibiotic concentration (Green and red indicate bacteria prior to and after mutation, respectively). (b) Serum concentration of an antibiotic as a function of time after administration, with MIC and MPC, indicated [adapted from reference 150] (with permission from Oxford university press).
![Figure 6. The concepts of mutant selection window (MSW) and mutant prevention concentration (MPC), as introduced by [Citation151]. (a) CFUs retrieved from a bacterial culture after antimicrobial exposure as a function of antibiotic concentration (Green and red indicate bacteria prior to and after mutation, respectively). (b) Serum concentration of an antibiotic as a function of time after administration, with MIC and MPC, indicated [adapted from reference 150] (with permission from Oxford university press).](/cms/asset/5e80017d-693d-4c3e-9115-ca95015f3d1f/iedd_a_1779697_f0006_oc.jpg)
If bacteria acquire resistance early in a mutant selection window ()), they will have a larger progeny than when resistance is acquired later. To account for this, assuming that acquisition of resistance is linear in time, mutation rate has been introduced, i.e. the number of bacteria acquiring resistance per viable organism per unit time after antimicrobial exposure [Citation149,Citation152].
5.2. Nano-antimicrobials not inducing resistance: do they exist?
Nano-antimicrobials frequently act on a non-antibiotic basis, requiring new bacterial mechanisms to evade killing. Bacteria have acquired already quite a number of mechanisms to resist antimicrobial action and it is considered likely that with the arrival of novel nano-antimicrobials, new mechanisms of antimicrobial resistance will undoubtedly arise over time as well.
Generation of heat by certain classes of novel nano-antimicrobials is presumed to kill bacteria indiscriminate of strain or species, but heat can cause collateral tissue damage which puts a limit to the amount of heat that can be generated in vivo. Heat-resistant bacteria exist already in various natural environments like hot-water springs [Citation153] and industrial applications [Citation154], like dairy processing units. Whether potential large-scale clinical use of photothermal nanoparticles will also convey heat-resistance to human clinical pathogens, is an open question. Equally, although bacteria possess little or no resistance against ROS, several bacterial strains and species are known to possess oxidative stress regulating genes that are crucial for their survival during exposure to endogenous or exogenous ROS exposure [Citation155]. E. coli and P. aeruginosa strains have been described to develop resistance to Ag nanoparticles after repeated exposure through the production of a flagellum protein causing aggregation of Ag nanoparticles and therewith their inactivation [Citation156]. This resistance mechanism could not be overcome by additional stabilization of the nanoparticles using surfactants or polymers.
These early warnings of potential new bacterial resistance mechanisms, warrants careful monitoring of the potential development of ROS-resistant or other novel nano-antimicrobial-resistant bacterial strains, might these become large scale used in the clinic. To prevent new bacterial resistance mechanisms against nano-antimicrobials to develop, it might be advisable to start their clinical use in combination with existing antibiotics. Serial-passaging of S. aureus in the presence of quaternary ammonium modified gold nanoclusters (QA-AuNCs), did not yield an increase in MIC up to 30 days of serial-passaging ()), whereas serial-passaging in the presence of oxacillin yielded a more than 30-fold increase in MIC [Citation157]. Similar observations were made for the exposure of P. aeruginosa to antimicrobial peptide coated Ag nanoparticles and ceftriaxone ()). Combining novel nano-antimicrobials with existing antibiotics may thus provide a promising pathway to face the upcoming crisis in the control of antimicrobial-resistant infections, not only because it will allow to continue clinical antibiotic treatment of infection using available antibiotics but also because it provides a clear pathway to make longer use of potential new antibiotics under development.
Figure 7. Development of resistance against antibiotics upon bacterial exposure to nano-antimicrobials as compared with exposure to existing antibiotics. (a). Development resistance toward S. aureus exposed to quaternary ammonium modified gold nanoclusters (QA AuNCs) as compared with the development of resistance against oxacillin [Citation157]. (with permission from Wiley-VCH Verlag GmbH & Co). (b). Development of resistance toward P. aeruginosa exposed to poly(ε-caprolactone)-b-antimicrobial peptide coated Ag nanoparticles (AgNPs@PCL-b-AMPs) as compared with the development of resistance against ceftriaxone [Citation158] (with permission from ACS Publications).
![Figure 7. Development of resistance against antibiotics upon bacterial exposure to nano-antimicrobials as compared with exposure to existing antibiotics. (a). Development resistance toward S. aureus exposed to quaternary ammonium modified gold nanoclusters (QA AuNCs) as compared with the development of resistance against oxacillin [Citation157]. (with permission from Wiley-VCH Verlag GmbH & Co). (b). Development of resistance toward P. aeruginosa exposed to poly(ε-caprolactone)-b-antimicrobial peptide coated Ag nanoparticles (AgNPs@PCL-b-AMPs) as compared with the development of resistance against ceftriaxone [Citation158] (with permission from ACS Publications).](/cms/asset/6735a7b9-43da-41c0-9659-c4a2dd366079/iedd_a_1779697_f0007_oc.jpg)
6. Reverting existing antibiotic-resistance
It is sometimes argued that the threat of antimicrobial-resistant infections becoming the number one cause of death [Citation7] in 2050 will disappear once we have effectively reduced the antibiotic-pressure present world-wide. Antimicrobial resistance will simply go away the same way as it came by selective pressure [Citation159,Citation160]. Bacteria that have acquired resistance through mutations in their DNA or horizontal gene transfer need to cope with the newly acquired resistance at the expense of considerable energy consumption, that makes it difficult to compete with naturally susceptible bacteria [Citation160]. Moreover, hetero-resistance is very common in many bacterial populations and facilitates the reversal of antibiotic-resistance [Citation159]. Based on this, it has been proposed to always use the antibiotic that requires most energy for a bacterium to deal with [Citation160,Citation161], while others suggest that drugs should be developed that stop horizontal gene transfer in infectious biofilms [Citation162].
7. Expert opinion
Rampant overuse of antibiotics has led to the development of bacterial strains and species that are resistant to all known antibiotics. The prospects of novel antibiotics being developed and brought to the clinical application are low, because of the high costs involved. Moreover, the ever-shorter time-period until the first resistance strains arrive after the clinical introduction of a new antibiotic reduces the chances upon return of investment, constituting a hurdle for needed industrial participation in the development process. Relying on natural outcompeting of antimicrobial-resistant bacteria after effectively reducing the antibiotic-pressure existing worldwide, seems like a difficult and impossible pathway, not only because of the scientific debate concerning its reality but also because it requires global, political consensus.
Therefore, it is advisable to focus research efforts not solely on making ever ‘better’ (read: ‘stronger’) antimicrobials, but much more on designing suitable combinations of existing antibiotics with new nano-antimicrobials in a way that induction of new antimicrobial-resistance mechanisms is avoided.
A final brief comment on novel antimicrobial strategies involves the pre-clinical, animal evaluation that is required before human clinical trials can be initiated. Pre-clinical animal evaluation of novel infection-control strategies will remain a conditio sine qua non despite the availability of advanced in vitro models and although it is generally acknowledged that animal studies bear little relation to human clinical outcomes [Citation163,Citation164]. For a wide variety of reasons, this may be especially true for the evaluation of novel infection-control strategies in animals. Work by Moriarty et al. [Citation163] has pointed to the importance of bacterial inoculum preparation, pathogen selection, quantification methodology, large inoculum doses, overuse of sub-cutaneous infection sites, use of healthy animal and low-aiming efficacy targets. Another important reason may be, that we are often not allowed to inflict experimental infections to animals that make them suffer. Opposite to cancer where a tumor can grow for a long period of time before causing clinical symptoms, patients suffering from infection can feel sick within days after the onset of infection and are in urgent need of medical treatment. The mild, often no-symptomatic, and self-curing experimental infections inflicted in animals are therewith fully at odds with the serious, antimicrobial-resistant infections we are supposed to find a cure for in our research [Citation165]. Therefore, it has recently been proposed that animal infection-models should use the prevention of death and recurrence of infection as primary efficacy targets to be addressed by novel strategies and not, e.g., bioluminescence or CFU enumeration. In many cases, application of the latter methods only serves to facilitate the publishing of academic papers but bear no clear translational aim. Advanced animal infection models that better mimic the characteristics of serious human infectious diseases may increase the predictive value of animal studies for the human situation and better facilitate clinical translation of novel antimicrobial-strategies [Citation165].
Article highlights
Nano-antimicrobials have entirely different mechanisms of action than current antimicrobials, including antibiotics.
Combination of novel nano-antimicrobials with an existing antibiotic is a good way to eradicate antimicrobial-resistant infections.
Development of novel antimicrobials is futile with the speed at which bacteria develop new resistance-mechanisms.
Development of infection-control strategies that do not induce resistance in bacterial pathogens should be prioritized.
Advanced animal models for evaluation of infection-control strategies are required to speed up clinical use of novel antimicrobials.
This box summarizes the key points contained in the article.
Declaration of interest
HJ Busscher is also director of a consulting company, SASA BV (GN Schutterlaan 4, 9797 PC Thesinge, The Netherlands). The authors have no other relevant affiliations or financial involvement with any organization or entity with a financial interest in or financial conflict with the subject matter or materials discussed in the manuscript apart from those disclosed.
Reviewer disclosures
Peer reviewers on this manuscript have no relevant financial or other relationships to disclose.
Additional information
Funding
References
- Andersson DI, Hughes D. Persistence of antibiotic resistance in bacterial populations. FEMS Microbiol Rev. 2011;35(5):901–911.
- Llor C, Bjerrum L. Antimicrobial resistance: risk associated with antibiotic overuse and initiatives to reduce the problem. Ther Adv Drug Saf. 2014;5:229–241.
- Högberg LD, Heddini A, Cars O. The global need for effective antibiotics: challenges and recent advances. Trends Pharmacol Sci. 2010;31(11):509–515.
- Klein E, Smith DL, Laxminarayan R. Hospitalizations and deaths caused by methicillin-resistant Staphylococcus aureus, United States, 1999-2005. Emerg Infect Dis. 2007;13(12):1840–1846.
- Hampton T. Report reveals scope of US antibiotic resistance threat. JAMA. 2013;310(16):1661–1663.
- Hofer U. The cost of antimicrobial resistance. Nat Rev Microbiol. 2019;17:3.
- Humphreys G, Fleck F. United Nations meeting on antimicrobial resistance. WHO B World Health Organ. 2016;94(9):638–639.
- Cassini A, Hogberg LD, Plachouras D, et al. Attributable deaths and disability-adjusted life-years caused by infections with antibiotic-resistant bacteria in the EU and the European Economic Area in 2015: a population-level modelling analysis. Lancet Infect Dis. 2019;19:56–66.
- Interagency Coordination Group on Antimicrobial Resistance. No time to wait: securing the future from drug-resistant infections - report to the secretary-general of the united nations Apr 2019. Cited 2020 May 19. https://www.who.int/antimicrobial-resistance/interagency-coordination-group/en
- Rossignoli A, Clavenna A, Bonati M. Antibiotic prescription and prevalence rate in the outpatient paediatric population: analysis of surveys published during 2000–2005. Eur J Clin Pharmacol. 2007;63(12):1099–1106.
- Luzzatto L, Hyry HI, Schieppati A, et al. Outrageous prices of orphan drugs: a call for collaboration. Lancet. 2018;392:791–794.
- Walsh C. Molecular mechanisms that confer antibacterial drug resistance. Nature. 2000;406(6797):775–781.
- Dolgin E. Sequencing of superbugs seen as key to combating their spread. Nat Med. 2010;16(10):1054.
- Dik J-WH, Vemer P, Friedrich AW, et al. Financial evaluations of antibiotic stewardship programs—a systematic review. Front Microbiol. 2015;6:317.
- Parisi OI, Scrivano L, Sinicropi MS, et al. Polymeric nanoparticle constructs as devices for antibacterial therapy. Curr Opin Pharmacol. 2017;36:72–77.
- Picca RA, Paladini F, Sportelli MC, et al. Combined approach for the development of efficient and safe nanoantimicrobials: the case of nanosilver-modified polyurethane foams. ACS Biomater Sci Eng. 2017;3(7):1417–1425.
- Zhao Z, Wang W, Li C, et al. Reactive oxygen species–activatable liposomes regulating hypoxic tumor microenvironment for synergistic photo/chemodynamic therapies. Adv Funct Mater. 2019;29(44):1905013–1905023.
- Liu C, Guo J, Yan X, et al. Antimicrobial nanomaterials against biofilms: an alternative strategy. Environ Rev. 2017;25:223–244.
- Busscher HJ, Van der Mei HC, Subbiahdoss G, et al. Biomaterial-associated infection: locating the finish line in the race for the surface. Sci Transl Med. 2012;4(153):153rv10.
- Flemming H-C, Wingender J, Szewzyk U, et al. Biofilms: an emergent form of bacterial life. Nat Rev Microbiol. 2016;14(9):563–575.
- Høiby N, Bjarnsholt T, Givskov M, et al. Antibiotic resistance of bacterial biofilms. Int J Antimicrob Agents. 2010;35(4):322–332.
- Quan K, Zhang Z, Chen H, et al. Artificial channels in an infectious biofilm created by magnetic nanoparticles enhanced bacterial killing by antibiotics. Small. 2019;15(39):1902313–1902318.
- de Beer D, Stoodley P, Lewandowski Z. Measurement of local diffusion coefficients in biofilms by microinjection and confocal microscopy. Biotechnol Bioeng. 1997;53(2):151–158.
- Stewart PS. Mini-review: convection around biofilms. Biofouling. 2012;28:187–198.
- Houry A, Gohar M, Deschamps J, et al. Bacterial swimmers that infiltrate and take over the biofilm matrix. Proc Natl Acad Sci USA. 2012;109(32):13088–13093.
- Wang Y, Wang Z, Xu C, et al. A disassembling strategy overcomes the EPR effect and renal clearance dilemma of the multifunctional theranostic nanoparticles for cancer therapy. Biomaterials. 2019;197:284–293.
- Romberg B, Hennink WE, Storm G. Sheddable coatings for long-circulating nanoparticles. Pharm Res. 2008;25(1):55–71.
- Liu Y, Shi L, Su L, et al. Nanotechnology-based antimicrobials and delivery systems for biofilm-infection control. Chem Soc Rev. 2019;48(2):428–446.
- Li H, Huang J, Song Y, et al. Degradable carbon dots with broad-spectrum antibacterial activity. ACS Appl Mater Inter. 2018;10(32):26936–26946.
- Zhao F, Gu W, Zhou J, et al. Solar-excited graphene quantum dots for bacterial inactivation via generation of reactive oxygen species. J Environ Sci Heal C. 2019;37(2):67–80.
- Cai T, Fang G, Tian X, et al. Optimization of antibacterial efficacy of noble-metal-based core–shell nanostructures and effect of natural organic matter. ACS Nano. 2019;13(11):12694–12702.
- Ge C, Wu R, Chong Y, et al. Synthesis of Pt hollow nanodendrites with enhanced peroxidase-like activity against bacterial infections: implication for wound healing. Adv Funct Mater. 2018;28(28):1801484–1801494.
- Applerot G, Lipovsky A, Dror R, et al. Enhanced antibacterial activity of nanocrystalline ZnO due to increased ROS‐mediated cell injury. Adv Funct Mater. 2009;19(6):842–852.
- Gechev TS, Van Breusegem F, Stone JM, et al. Reactive oxygen species as signals that modulate plant stress responses and programmed cell death. Bioessays. 2006;28(11):1091–1101.
- Thomas DD. Breathing new life into nitric oxide signaling: A brief overview of the interplay between oxygen and nitric oxide. Redox Biol. 2015;5:225–233.
- Schapiro JM, Libby SJ, Fang FC. Inhibition of bacterial DNA replication by zinc mobilization during nitrosative stress. Proc Natl Acad Sci USA. 2003;100:8496–8501.
- Mihu MR, Cabral V, Pattabhi R, et al. Sustained nitric oxide-releasing nanoparticles interfere with methicillin-resistant Staphylococcus aureus adhesion and biofilm formation in a rat central venous catheter model. Antimicrob Agents Chemother. 2017;61(1):e02020–16.
- Liu Z, Wang F, Ren J, et al. A series of MOF/Ce-based nanozymes with dual enzyme-like activity disrupting biofilms and hindering recolonization of bacteria. Biomaterials. 2019;208:21–31.
- Flemming H-C, Wingender J. The biofilm matrix. Nat Rev Microbiol. 2010;8(9):623–633.
- Weldrick PJ, Hardman MJ, Paunov VN. Enhanced clearing of wound-related pathogenic bacterial biofilms using protease-functionalized antibiotic nanocarriers. ACS Appl Mater Inter. 2019;11(47):43902–43919.
- Smith AW. Biofilms and antibiotic therapy: is there a role for combating bacterial resistance by the use of novel drug delivery systems? Adv Drug Deliv Rev. 2005;57(10):1539–1550.
- Gogokhia L, Buhrke K, Bell R, et al. Expansion of bacteriophages is linked to aggravated intestinal inflammation and colitis. Cell Host Microbe. 2019;25(2):285–299.
- Park J-H, von Maltzahn G, Ong LL, et al. Cooperative nanoparticles for tumor detection and photothermally triggered drug delivery. Adv Mater. 2010;22(8):880–885.
- Yan H, Teh C, Sreejith S, et al. Functional mesoporous silica nanoparticles for photothermal-controlled drug delivery in vivo. Angew Chem Int Edit. 2012;51(33):8373–8377.
- Huang J, Zhou J, Zhuang J, et al. Strong near-infrared absorbing and biocompatible CuS nanoparticles for rapid and efficient photothermal ablation of gram-positive and-negative bacteria. ACS Appl Mater Inter. 2017;9(42):36606–36614.
- Yu S, Li G, Liu R, et al. Dendritic Fe3O4@Poly(dopamine)@PAMAM nanocomposite as controllable NO-releasing material: a synergistic photothermal and NO antibacterial study. Adv Funct Mater. 2018;28(20):1707440–1707453.
- Pihl M, Bruzell E, Andersson M. Bacterial biofilm elimination using gold nanorod localised surface plasmon resonance generated heat. Mater Sci Eng C. 2017;80:54–58.
- Ghosh S, Sahoo N, Sajanlal P, et al. Anomalous subsurface thermal behavior in tissue mimics upon near infrared irradiation mediated photothermal therapy. J Biomed Nanotechnol. 2014;10(3):405–414.
- Quan K, Zhang Z, Ren Y, et al. Homogeneous distribution of magnetic, antimicrobial-carrying nanoparticles through an infectious biofilm enhances biofilm-killing efficacy. ACS Biomater Sci Eng. 2019;6(1):205–212.
- Espinosa A, Di Corato R, Kolosnjaj-Tabi J, et al. Duality of iron oxide nanoparticles in cancer therapy: amplification of heating efficiency by magnetic hyperthermia and photothermal bimodal treatment. ACS Nano. 2016;10(2):2436–2446.
- Chen C, Wang S, Li L, et al. Bacterial magnetic nanoparticles for photothermal therapy of cancer under the guidance of MRI. Biomaterials. 2016;104:352–360.
- Subbiahdoss G, Sharifi S, Grijpma DW, et al. Magnetic targeting of surface-modified superparamagnetic iron oxide nanoparticles yields antibacterial efficacy against biofilms of gentamicin-resistant staphylococci. Acta Biomater. 2012;8(6):2047–2055.
- Geilich BM, Gelfat I, Sridhar S, et al. Superparamagnetic iron oxide-encapsulating polymersome nanocarriers for biofilm eradication. Biomaterials. 2017;119:78–85.
- Wang X, Wu J, Li P, et al. Microenvironment-responsive magnetic nanocomposites based on silver nanoparticles/gentamicin for enhanced biofilm disruption by magnetic field. ACS Appl Mater Inter. 2018;10(41):34905–34915.
- Franci G, Falanga A, Galdiero S, et al. Silver nanoparticles as potential antibacterial agents. Molecules. 2015;20(5):8856–8874.
- Singh V, Kumar V, Kashyap S, et al. Graphene oxide synergistically enhances antibiotic efficacy in vancomycin-resistant Staphylococcus aureus. ACS Appl Bio Mater. 2019;2(3):1148–1157.
- Tu Y, Lv M, Xiu P, et al. Destructive extraction of phospholipids from Escherichia coli membranes by graphene nanosheets. Nat Nanotechnol. 2013;8(8):594–601.
- Huo S, Jiang Y, Gupta A, et al. Fully zwitterionic nanoparticle antimicrobial agents through tuning of core size and ligand structure. ACS Nano. 2016;10(9):8732–8737.
- Pelgrift RY, Friedman AJ. Nanotechnology as a therapeutic tool to combat microbial resistance. Adv Drug Deliv Rev. 2013;65(13):1803–1815.
- Blecher K, Nasir A, Friedman A. The growing role of nanotechnology in combating infectious disease. Virulence. 2011;2(5):395–401.
- Maan AA, Nazir A, Khan MKI, et al. The therapeutic properties and applications of Aloe vera: a review. J Herbs Med. 2018;12:1–10.
- Ghafoor B, Ali MN, Ansari U, et al. New biofunctional loading of natural antimicrobial agent in biodegradable polymeric films for biomedical applications. Int J Biomater. 2016;2016:1–9.
- Su Y, Zhao L, Meng F, et al. Triclosan loaded polyurethane micelles with pH and lipase sensitive properties for antibacterial applications and treatment of biofilms. Mater Sci Eng C. 2018;93:921–930.
- Liu Y, Busscher HJ, Zhao B, et al. Surface-adaptive, antimicrobially loaded,micellar nanocarriers with enhanced penetration and killing efficiency in staphylococcal biofilms. ACS Nano. 2016;10(4):4779–4789.
- Mi L, Jiang S. Integrated antimicrobial and nonfouling zwitterionic polymers. Angew Chem Int Edit. 2014;53(7):1746–1754.
- Hu D, Li H, Wang B, et al. Surface-adaptive gold nanoparticles with effective adherence and enhanced photothermal ablation of methicillin-resistant Staphylococcus aureus biofilm. ACS Nano. 2017;11(9):9330–9339.
- Xu HV, Zheng XT, Wang C, et al. Bioinspired antimicrobial nanodots with amphiphilic and zwitterionic-like characteristics for combating multidrug-resistant bacteria and biofilm removal. ACS Appl Nano Mater. 2018;1(5):2062–2068.
- Liu X, Pang J, Xu F, et al. Simple approach to synthesize amino-functionalized carbon dots by carbonization of chitosan. Sci Rep. 2016;6(1):31100–31107.
- Dong Y, Shao J, Chen C, et al. Blue luminescent graphene quantum dots and graphene oxide prepared by tuning the carbonization degree of citric acid. Carbon. 2012;50(12):4738–4743.
- Bagheri Z, Ehtesabi H, Rahmandoust M, et al. New insight into the concept of carbonization degree in synthesis of carbon dots to achieve facile smartphone based sensing platform. Sci Rep. 2017;7(1):11013–11023.
- Choi SK, Myc A, Silpe JE, et al. Dendrimer-based multivalent vancomycin nanoplatform for targeting the drug-resistant bacterial surface. ACS Nano. 2013;7(1):214–228.
- Wrońska N, Felczak A, Zawadzka K, et al. Poly (propylene imine) dendrimers and amoxicillin as dual-action antibacterial agents. Molecules. 2015;20(10):19330–19342.
- Dai T, Wang C, Wang Y, et al. A nanocomposite hydrogel with potent and broad-spectrum antibacterial activity. ACS Appl Mater Inter. 2018;10(17):15163–15173.
- Lu Y, Slomberg DL, Shah A, et al. Nitric oxide-releasing amphiphilic poly (amidoamine)(PAMAM) dendrimers as antibacterial agents. Biomacromolecules. 2013;14(10):3589–3598.
- Fux CA, Costerton JW, Stewart PS, et al. Survival strategies of infectious biofilms. Trends Microbiol. 2005;13(1):34–40.
- Van Leeuwenhoek A. Microscopical observations, about animals in the scurf of the teeth, the substance call’d worms in the nose, the cuticula consisting of scales. Philos Trans R Soc Lond. 1684;14:568–574.
- Wright GD. Q&A: antibiotic resistance: where does it come from and what can we do about it? BMC Biol. 2010;8(1):123.
- Whitchurch CB, Tolker-Nielsen T, Ragas PC, et al. Extracellular DNA required for bacterial biofilm formation. Science. 2002;295(5559):1487.
- McDougald D, Rice SA, Barraud N, et al. Should we stay or should we go: mechanisms and ecological consequences for biofilm dispersal. Nat Rev Microbiol. 2012;10(1):39–50.
- Harapanahalli AK, Chen Y, Li J, et al. Influence of adhesion force on icaA and cidA gene expression and production of matrix components in Staphylococcus aureus biofilms. Appl Environ Microbiol. 2015;81(10):3369–3378.
- Muszanska AK, Nejadnik MR, Chen Y, et al. Bacterial adhesion forces with substratum surfaces and the susceptibility of biofilms to antibiotics. Antimicrob Agents Chemother. 2012;56(9):4961–4964.
- Borriello G, Werner E, Roe F, et al. Oxygen limitation contributes to antibiotic tolerance of Pseudomonas aeruginosa in biofilms. Antimicrob Agents Chemother. 2004;48(7):2659–2664.
- Boles BR, Horswill AR. Staphylococcal biofilm disassembly. Trends Microbiol. 2011;19(9):449–455.
- Wood TK, Knabel SJ, Kwan BW. Bacterial persister cell formation and dormancy. Appl Environ Microbiol. 2013;79(23):7116–7121.
- El-Halfawy OM, Valvano MA. Antimicrobial heteroresistance: an emerging field in need of clarity. Clin Microbiol Rev. 2015;28(1):191–207.
- Nicoloff H, Hjort K, Levin BR, et al. The high prevalence of antibiotic heteroresistance in pathogenic bacteria is mainly caused by gene amplification. Nat Microbiol. 2019;4(3):504–514.
- Nesse LL, Simm R. A hotspot for emerging bacterial genotypes. Adv App Microbiol. 2018;103:223–246.
- Sun D. Pull in and push out: mechanisms of horizontal gene transfer in bacteria. Front Microbiol. 2018;9:2154–2161.
- Hengzhuang W, Wu H, Ciofu O, et al. Pharmacokinetics/pharmacodynamics of colistin and imipenem on mucoid and nonmucoid Pseudomonas aeruginosa biofilms. Antimicrob Agents Chemother. 2011;55(9):4469–4474.
- Hengzhuang W, Wu H, Ciofu O, et al. In vivo pharmacokinetics/pharmacodynamics of colistin and imipenem in Pseudomonas aeruginosa biofilm infection. Antimicrob Agents Chemother. 2012;56(5):2683–2690.
- Ramirez MS, Tolmasky ME. Aminoglycoside modifying enzymes. Drug Resist Update. 2010;13(6):151–171.
- Criswell D The “evolution” of antibiotic resistance.” Institute for creation research. Web 28. Oct 2014. 2004.
- Blair JMA, Webber MA, Baylay AJ, et al. Molecular mechanisms of antibiotic resistance. Nat Rev Microbiol. 2015;13(1):42–51.
- Ma Y-X, Wang C-Y, Li -Y-Y, et al. Considerations and caveats in combating ESKAPE pathogens against nosocomial Infections. Adv Sci. 2020;7(1):1901872–1901914.
- Markley JL, Fang L, Gasparrini AJ, et al. Semisynthetic analogues of anhydrotetracycline as inhibitors of tetracycline destructase enzymes. ACS Infect Dis. 2019;5(4):618–633.
- Li F, Collins JG, Keene FR. Ruthenium complexes as antimicrobial agents. Chem Soc Rev. 2015;44(8):2529–2542.
- Lee AS, de Lencastre H, Garau J, et al. Methicillin-resistant Staphylococcus aureus. Nat Rev Dis Primers. 2018;4:18033–18056.
- Al-Tawfiq JA, Laxminarayan R, Mendelson M. How should we respond to the emergence of plasmid-mediated colistin resistance in humans and animals? Int J Infect Dis. 2017;54:77–84.
- Enright MC, Robinson DA, Randle G, et al. The evolutionary history of methicillin-resistant Staphylococcus aureus (MRSA). Proc Natl Acad Sci USA. 2002;99(11):7687–7692.
- Fabbretti A, Çapuni R, Giuliodori AM, et al. Characterization of the self-resistance mechanism to dityromycin in the Streptomyces producer strain. mSphere. 2019;4(5):e00554–19.
- Barrangou R, Fremaux C, Deveau H, et al. CRISPR provides acquired resistance against viruses in prokaryotes. Science. 2007;315(5819):1709–1712.
- Barrangou R. The roles of CRISPR–Cas systems in adaptive immunity and beyond. Curr Opin Immunol. 2015;32:36–41.
- Fuda CC, Fisher JF, Mobashery S. Beta-lactam resistance in Staphylococcus aureus: the adaptive resistance of a plastic genome. Cell Mol Life Sci. 2005;62:2617–2633.
- Wang K, Nicholaou M. Suppression of antimicrobial resistance in MRSA using CRISPR-dCas9. Clin Lab Sci. 2017;30(4):207–213.
- Horvath P, Barrangou R. CRISPR/Cas, the immune system of bacteria and archaea. Science. 2010;327(5962):167–170.
- Weissman JL, Laljani RM, Fagan WF, et al. Visualization and prediction of CRISPR incidence in microbial trait-space to identify drivers of antiviral immune strategy. ISME. 2019;13(10):2589–2602.
- Koebnik R, Locher KP, Van Gelder P. Structure and function of bacterial outer membrane proteins: barrels in a nutshell. Mol Microbiol. 2000;37(2):239–253.
- Masuda N, Sakagawa E, Ohya S, et al. Substrate specificities of MexAB-OprM, MexCD-OprJ, and MexXY-oprM efflux pumps in Pseudomonas aeruginosa. Antimicrob Agents Chemother. 2000;44(12):3322–3327.
- Ferrer-Espada R, Shahrour H, Pitts B, et al. A permeability-increasing drug synergizes with bacterial efflux pump inhibitors and restores susceptibility to antibiotics in multi-drug resistant Pseudomonas aeruginosa strains. Sci Rep. 2019;9(1):3452–3463.
- Piddock LJV. Multidrug-resistance efflux pumps? Not just for resistance. Nat Rev Microbiol. 2006;4(8):629–636.
- Putman M, van Veen HW, Konings WN. Molecular properties of bacterial multidrug transporters. Microbiol Mol Biol Rev. 2000;64(4):672–693.
- Poole K. Efflux pumps as antimicrobial resistance mechanisms. Ann Med. 2007;39(3):162–176.
- Blanco P, Hernando-Amado S, Reales-Calderon JA, et al. Bacterial multidrug efflux pumps: much more than antibiotic resistance determinants. Microorganisms. 2016;4(1):14–27.
- Blake KL, Randall CP, Neill AJ. In vitro studies indicate a high resistance potential for the lantibiotic nisin in Staphylococcus aureus and define a genetic basis for nisin resistance. Antimicrob Agents Chemother. 2011;55(5):2362–2368.
- Carniello V, Harapanahalli AK, Busscher HJ, et al. Adhesion force sensing and activation of a membrane-bound sensor to activate nisin efflux pumps in Staphylococcus aureus under mechanical and chemical stresses. J Colloid Interf Sci. 2018;512:14–20.
- Abed N, Couvreur P. Nanocarriers for antibiotics: A promising solution to treat intracellular bacterial infections. Int J Antimicrob Agents. 2014;43(6):485–496.
- Ahmad Nor Y, Niu Y, Karmakar S, et al. Shaping nanoparticles with hydrophilic compositions and hydrophobic properties as nanocarriers for antibiotic delivery. ACS Central Sci. 2015;1(6):328–334.
- Liu Y, Ren Y, Li Y, et al. Nanocarriers with conjugated antimicrobials to eradicate pathogenic biofilms evaluated in murine in vivo and human ex vivo infection models. Acta Biomater. 2018;79:331–343.
- Ejim L, Farha MA, Falconer SB, et al. Combinations of antibiotics and nonantibiotic drugs enhance antimicrobial efficacy. Nat Chem Biol. 2011;7(6):348–350.
- Lazzarini L, Lipsky BA, Mader JT. Antibiotic treatment of osteomyelitis: what have we learned from 30 years of clinical trials? Int J Infect Dis. 2005;9(3):127–138.
- Brooks BD, Brooks AE. Therapeutic strategies to combat antibiotic resistance. Adv Drug Deliv Rev. 2014;78:14–27.
- Friedman AJ, Phan J, Schairer DO, et al. Antimicrobial and anti-Inflammatory activity of chitosan–alginate nanoparticles: A targeted therapy for cutaneous pathogens. J Invest Dermatol. 2013;133(5):1231–1239.
- Allen TM, Cullis PR. Liposomal drug delivery systems: from concept to clinical applications. Adv Drug Deliv Rev. 2013;65(1):36–48.
- Beppler C, Tekin E, White C, et al. When more is less: emergent suppressive interactions in three-drug combinations. BMC Microbiol. 2017;17(1):107–115.
- Neut D, Hendriks JGE, van Horn JR, et al. Antimicrobial efficacy of gentamicin-loaded acrylic bone cements with fusidic acid or clindamycin added. J Orthop Res. 2006;24(2):291–299.
- Dey R, De K, Mukherjee R, et al. Small antibacterial molecules highly active against drug-resistant Staphylococcus aureus. MedChemComm. 2019;10(11):1907–1915.
- Mwangi J, Yin Y, Wang G, et al. The antimicrobial peptide ZY4 combats multidrug-resistant Pseudomonas aeruginosa and Acinetobacter baumannii infection. Proc Natl Acad Sci USA. 2019;116(52):26516–26522.
- Armengol E, Domenech O, Fusté E, et al. Efficacy of combinations of colistin with other antimicrobials involves membrane fluidity and efflux machinery. Infect Drug Resist. 2019;12:2031–2038.
- Wu D-Q, Zhu J, Han H, et al. Synthesis and characterization of arginine-NIPAAm hybrid hydrogel as wound dressing: in vitro and in vivo study. Acta Biomater. 2018;65:305–316.
- Onesti M, Carella S, Scuderi N. Effectiveness of antimicrobial-coated sutures for the prevention of surgical site infection: a review of the literature. Eur Rev Med Pharmacol Sci. 2018;22:5729–5739.
- Ruden S, Rieder A, Schwartz T, et al. Synergy pattern of short cationic antimicrobial peptides against multidrug-resistant Pseudomonas aeruginosa. Front Microbiol. 2019;10:2740.
- Maiden MM, Hunt AMA, Zachos MP, et al. Triclosan is an aminoglycoside adjuvant for eradication of Pseudomonas aeruginosa biofilms. Antimicrob Agents Chemother. 2018;62(6):e00146–18.
- Westfall C, Flores-Mireles AL, Robinson JI, et al. The widely used antimicrobial triclosan induces high levels of antibiotic tolerance in vitro and reduces antibiotic efficacy up to 100-fold in vivo. Antimicrob Agents Chemother. 2019;63(5):e02312–18.
- Khan R, Islam B, Akram M, et al. Antimicrobial activity of five herbal extracts against multi drug resistant (MDR) strains of bacteria and fungus of clinical origin. Molecules. 2009;14(2):586–597.
- Solórzano-Santos F, Miranda-Novales MG. Essential oils from aromatic herbs as antimicrobial agents. Curr Opin Biotech. 2012;23(2):136–141.
- Palaniappan K, Holley RA. Use of natural antimicrobials to increase antibiotic susceptibility of drug resistant bacteria. Int J Food Microbiol. 2010;140(2):164–168.
- Aburjai T, Darwish RM, Al-Khalil S, et al. Screening of antibiotic resistant inhibitors from local plant materials against two different strains of Pseudomonas aeruginosa. J Ethnopharmacol. 2001;76(1):39–44.
- Hemaiswarya S, Kruthiventi AK, Doble M. Synergism between natural products and antibiotics against infectious diseases. Phytomedicine. 2008;15(8):639–652.
- Carpenter AW, Slomberg DL, Rao KS, et al. Influence of scaffold size on bactericidal activity of nitric oxide-releasing silica nanoparticles. ACS Nano. 2011;5(9):7235–7244.
- Nye R, Robinia K, Peterson P, et al. Efficacy of a nitric oxide dressing in decreasing bacterial counts on human skin. J Wound Care. 2018;27(Sup7):S19–S25.
- Dizaj SM, Lotfipur F, Barzegar-Jalali M, et al. Antimicrobial activity of the metals and metal oxide nanoparticles. Mater Sci Eng C. 2014;44(1):278–284.
- Le Ouay B, Stellacci F. Antibacterial activity of silver nanoparticles: A surface science insight. Nano Today. 2015;10(3):339–354.
- Huh AJ, Kwon YJ. “Nanoantibiotics”: A new paradigm for treating infectious diseases using nanomaterials in the antibiotics resistant era. J Control Release. 2011;156(2):128–145.
- Egorov A, Rubtsova M, Grigorenko V, et al. The role of the Ω-loop in regulation of the catalytic activity of TEM-type β-lactamases. Biomolecules. 2019;9(12):854–869.
- Tooke CL, Hinchliffe P, Bragginton EC, et al. β-Lactamases and β-lactamase inhibitors in the 21st century. J Mol Biol. 2019;431(18):3472–3500.
- Duan F, Feng X, Jin Y, et al. Metal–carbenicillin framework-based nanoantibiotics with enhanced penetration and highly efficient inhibition of MRSA. Biomaterials. 2017;144:155–165.
- Zhao X, Drlica K. Restricting the selection of antibiotic-resistant mutants: a general strategy derived from fluoroquinolone studies. Clin Infect Dis. 2001;33(Supplement_3):S147–S156.
- Hong J, Hu J, Ke F. Experimental induction of bacterial resistance to the antimicrobial peptide tachyplesin I and investigation of the resistance mechanisms. Antimicrob Agents Chemother. 2016;60(10):6067–6075.
- Pope CF, Sullivan DM, McHugh TD, et al. A practical guide to measuring mutation rates in antibiotic resistance. Antimicrob Agents Chemother. 2008;52(4):1209–1214.
- Drlica K, Zhao X. Mutant selection window hypothesis updated. Clin Infect Dis. 2007;44(5):681–688.
- Dong Y, Zhao X, Domagala J, et al. Effect of fluoroquinolone concentration on selection of resistant mutants of Mycobacterium bovis BCG and Staphylococcus aureus. Antimicrob Agents Chemother. 1999;43(7):1756–1758.
- Cirz RT, Romesberg FE. Induction and inhibition of ciprofloxacin resistance-conferring mutations in hypermutator bacteria. Antimicrob Agents Chemother. 2006;50(1):220–225.
- Sen SK, Mohapatra SK, Satpathy S, et al. Characterization of hot water spring source isolated clones of bacteria and their industrial applicability. Int J Chem Res. 2010;2(1):01–07.
- Gleeson D, O’Connell A, Jordan K. Review of potential sources and control of thermoduric bacteria in bulk-tank milk. Irish J Agr Food Res. 2013;52(2):217–227.
- Vatansever F, de Melo WC, Avci P, et al. Antimicrobial strategies centered around reactive oxygen species–bactericidal antibiotics, photodynamic therapy, and beyond. FEMS Microbiol Rev. 2013;37(6):955–989.
- Panáček A, Kvítek L, Smékalová M, et al. Bacterial resistance to silver nanoparticles and how to overcome it. Nat Nanotech. 2018;13(1):65–71.
- Xie Y, Liu Y, Yang J, et al. Gold nanoclusters for targeting methicillin-resistant Staphylococcus aureus in vivo. Angew Chem Int Edit. 2018;57(15):3958–3962.
- Zhen J-B, Kang P-W, Zhao M-H, et al. Silver nanoparticle conjugated star PCL-b-AMPs copolymer as nanocomposite exhibits efficient antibacterial properties. Bioconjugate Chem. 2020;31(1):51–63.
- Andersson DI, Nicoloff H, Hjort K. Mechanisms and clinical relevance of bacterial heteroresistance. Nat Rev Microbiol. 2019;17:479–496.
- Silva RF, Mendonça SC, Carvalho LM, et al. Pervasive sign epistasis between conjugative plasmids and drug-resistance chromosomal mutations. PLoS Gent. 2011;7(7):e1002181.
- Purcell A. Superbugs may be here to stay. NewScientist. 2011 August;2824:6.
- Getino M, Sanabria-Ríos DJ, Fernández-López R, et al. Synthetic fatty acids prevent plasmid-mediated horizontal gene transfer. mBio. 2015;6(5):e01032–15.
- Moriarty T, Grainger D, Richards R. Challenges in linking preclinical anti-microbial research strategies with clinical outcomes for device-associated infections. Eur Cell Mater. 2014;28:112–128.
- Campoccia D, Montanaro L, Moriarty T, et al. The selection of appropriate bacterial strains in preclinical evaluation of infection-resistant biomaterials. Int J Artif Organs. 2008;31(9):841–847.
- Busscher HJ, Woudstra W, van Kooten TG, et al. Accepting higher morbidity in exchange for sacrificing fewer animals in studies developing novel infection-control strategies. Biomaterials. 2020;232:119737–119744.