ABSTRACT
Introduction
Therapeutic enzymes are currently used in the treatment of several diseases. In most cases, the benefits are limited due to poor in vivo stability, immunogenicity, and drug-induced inactivating antibodies. A partial solution to the problem is obtained by masking the therapeutic protein by chemical modifications. Unfortunately, this is not a satisfactory solution because frequent adverse events, including anaphylaxis, can arise.
Area covered
Among the delivery systems, we focused on red blood cells for the delivery of therapeutic enzymes. Erythrocytes possess a long circulation time, a reduced immunogenicity, there is no need of chemical modifications and the encapsulated enzyme remains active because it is protected by the cell membrane. Here we discuss some representative applications of the preclinical developments of the field. Some of these are currently in clinic, others are approaching the clinic and others are illustrative of the development process. The selected examples are not always the most recent, but they are the most useful for a comparative approach.
Expert opinion
The results discussed confirm the central role that red blood cells can play in the treatment of several conditions and suggest the benefit in using a natural cellular carrier in terms of pharmacokinetic, biodistribution, safety, and efficacy.
1. Introduction
Enzyme-loaded red blood cells (RBCs) were suggested by Ihler et al. [Citation1] as bioreactors for the delivery of therapeutic proteins in the circulatory system since 1973. Different techniques have been developed since then, but all of them are based on the remarkable ability of the red cell to undergo reversible changes of shape of the membrane and transient opening of pores on it. The most studied methods for obtaining protein-loaded erythrocytes are electroporation, drug-induced endocytosis, osmotic pulse, and hypotonic hemolysis. Among these, hypotonic hemolysis achieved the greatest development being the most used in preclinical and clinical studies. Indeed, under hypotonic conditions pores till to 500 Å open on the erythrocyte membrane, permitting the entrance of one or more proteins, and by restoring physiological osmotic conditions, the membranes reseal and RBCs reassume their normal biconcave shape and impermeability features. Once in circulation, resealed RBCs show an almost normal survival times as demonstrated by the half-life values both of murine [Citation2,Citation3] and human [Citation4,Citation5] loaded RBCs. Another potential developing technology is based on the manufacture of red blood cells expressing bio-therapeutic proteins to create highly selective and allogeneic cellular medicines aimed at treating a range of diseases by the hematopoietic stem cell therapy [www.rubiustx.com]. Due to the limits of this approach, the company has suspended this program.
When the enzyme is confined inside RBCs, it is protected from premature degradation, inactivation, or excretion. Furthermore, enzyme-loaded erythrocytes can act as long-circulating bioreactors able to clear undesirable cell membrane-permeating molecules from the bloodstream, thereby paving the way for numerous clinical applications and overcoming both enzyme bioavailability and immunogenicity issues. Indeed, the clinical progress in enzyme-loaded RBC technologies has been documented in a recent review [Citation6]. Moreover, the continuous development in the cloning of recombinant enzymes and in the expression and purification procedures has created the opportunity to dispose of a virtually infinite array of proteins in large amounts that could be used in the clinical setting to treat several pathological conditions.
It is noteworthy that the most advanced clinical developments are based on solid preclinical investigations, suggesting the importance of preliminary studies on animal models to collect data on the feasibility, safety, and immunogenicity before first-in-man studies and clinical trials. The encapsulation’s potential of RBCs from different mammalian species has been at first evaluated by De Loach [Citation7]; since then, many authors have documented the development and efficacy of enzyme-loaded RBCs in animal models, as reviewed in [Citation8] and in a very elegant and comprehensive recent work by Bax [Citation9]. The enzyme-loaded RBCs efficacy in animal models is also highlighted and listed in .
Table 1. Preclinical studies for the different applications of RBCs as enzyme delivery system
In this review, due to space limitation, we focus only on those preclinical studies of enzyme-loaded RBCs that have successfully lead to clinical applications (such as in Mitochondrial neurogastrointestinal encephalomyopathy disease and some cancers) and on preclinical studies which are potentially very close to a realization in humans (such as Phenylketonuria disease). Finally, we discuss as an example two promising applications that need further evaluations in animal models (alcohol and ammonia detoxification). In , a schematic representation of the preclinical applications here discussed is reported.
Figure 1. Enzyme-loaded red blood cells. Schematic representation of the preclinical applications of enzyme-loaded RBCs in: Mitochondrial neurogastrointestinal encephalomyopathy (MNGIE); Cancer; Phenylketonuria (PKU); Alcohol detoxification and Hyperammonemia. Thymidine phosphorylase (TP); deoxyuridine (dUridine); deoxyuracile (dUracile); methionine gamma-lyase (MGL); asparaginase (ASNase); arginine deiminase (ADI); phenylalanine hydroxylase (PAH), phenylalanine ammonia-lyase (PAL); acetaldehyde dehydrogenase (AcDH); alcohol oxidase (AlOx); glutamate dehydrogenase (GDH); glutamine synthetase (GS); alanine aminotransferase (AAT). Figure does not detail the existing different diffusion rate of the substrates and the different efficiency of enzymes in metabolizing them that can significantly contribute in defining the efficacy of the system
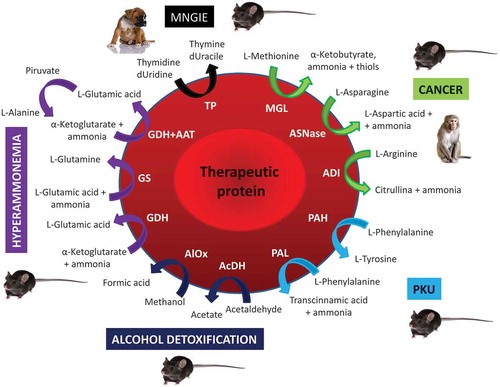
2. Mitochondrial neurogastrointestinal encephalomyopathy
Mitochondrial neurogastrointestinal encephalomyopathy (MNGIE) is a rare autosomal recessive disease resulting in a multisystem disorder, a progressive morbidity, premature death and it is caused by deficiency in thymidine phosphorylase (TP) activity (EC 2.4.2.4). From the biochemical point of view deficiency in thymidine phosphorylase activity results in elevated concentrations of thymidine and deoxyuridine, which in turn generate imbalances within the mitochondrial nucleotide pools and mitochondrial dysfunction. In 2008 [Citation25] RBC-encapsulated thymidine phosphorylase was proved to be effective in reducing plasma thymidine and deoxyuridine concentrations, providing clear biochemical evidence that the approach was successful. Clinical improvement was also demonstrated in a single patient during 27 treatment cycles without relevant toxicities [Citation26]. Based on these and other observations, both FDA and EMA provided to erythrocyte-encapsulated thymidine phosphorylase the status of Orphan Drug. Safety and efficacy were confirmed extending compassionate treatment of the first patient for additional 49 months and two additional patients [Citation27]. A Phase 2, multi-center, multiple-dose, open-label trial without a control was then designed as an enzyme-replacement therapy for MNGIE (ClinicalTrials.gov, NCT03866954). Three erythrocyte-TP dose levels were planned, with patients receiving the required dose level in order to achieve the metabolic correction. Study duration was 31 months, with 24 months of treatment. The primary objectives which have been considered were safety, tolerability, pharmacodynamic, and the efficacy of multiple doses of erythrocyte-TP [Citation28]. To start this study, a robust preclinical development was needed. Preclinical toxicity was assessed in two animal models: rodents and dogs [Citation29]. The study, under GLP, considered IV administrations of erythrocyte-TP for 4 weeks, twice per week. The doses selected were 4–6 times the expected human dose. In mice, thrombi/emboli in the lungs were recorded compared to controls. Spleen enlargement was also evident. Anti-drug antibodies were observed in all animals, as expected from the administration of a recombinant bacterial enzyme. In dogs, the observed level of clinical signs during or shortly after dosing was significant and increased with each subsequent dosing occasion. Medications were only partially effective and nonspecific antibodies were detected in all animals. Anti-drug antibodies were found only in two animals. Other toxicities were evident, including hematological abnormalities, thymus atrophy, reduced body weight vs controls, and other minor modifications. The authors conclude that the results do not envisage potential serious toxicities to prevent further clinical developments. It is noteworthy that these abnormalities were not observed in human patients under compassionate treatment with the same system. Thrombi or emboli in the lung were newer observed in treated patients, hence a possible explanation should likely be found in the use of allogenic blood obtained from the same strain of animals, instead of autologous erythrocytes. This would justify while the same phenomena were not observed in dogs treated with autologous blood. This explanation is not totally convincing, since a number of studies on the use of mice allogenic blood to deliver drugs or enzymes were never reported to be associated with thrombi formation. One possible alternative explanation is the presence of a significant amount of endotoxins in the enzyme preparation used. This has been observed also in our laboratory as a cause of thrombi in the lungs. Finally, both mice and dogs used in these studies were normal animal not showing the disease. These researches recommend attention in clinical studies and in the monitoring of antidrug antibodies. The results reported in humans under compassionate treatment indicate any or limited antibody response as in dogs, but the figure is totally different in mice. Reduction of thymus in dogs should be of further concern and likely drug related.
3. Cancer
Cancer is a serious health problem in all populations, regardless of wealth or social status. In 2018, 18.1 million people around the world had cancer and 9.6 million died from the disease. By 2040, those figures will nearly double, with the greatest increase in low- and middle-income countries, where more than two-thirds of the world’s cancers will occur (WHO REPORT ON CANCER setting priorities, investing wisely and providing care for all. 2020). The past half century has seen tremendous progress in cancer treatment, mainly through advances in systemic therapy and more recently in immunotherapy, as well as refinements in radiotherapy and surgery. Some illustrative examples of enzymatic therapy are here discussed.
3.1. Methionine gamma-lyase as cancer therapy
Growing insight into cancer metabolism is highlighting the importance of nutrient supply to tumor development and therapeutic response. Interestingly, several research findings have shown that amino acid (AA) restrictions play a significant role in cancer interventions, given that many tumor cells show exogenous amino acid-dependency. Among the other, the exogenous source of the sulfur amino acid methionine (Met), makes dietary methionine restriction (MR) a promising tool in the treatment of cancer, as extensively documented [Citation30,Citation31].
Met depletion induces many modifications in tumor cells, including cell arrest in the S and G2 phases of the cell cycle, apoptosis, decrease of glutathione (GSH) content, and reduced activity of the O6-methylguanine-DNA methyltransferase (MGMT) [Citation32,Citation33]. In addition to inhibiting cancer cell growth, an increasing efficacy of chemo- and radiotherapy has been observed in animal models following MR [Citation34]. Moreover, the avidity of aggressive brain tumor cells for Met led to use 11C-Met positron emission tomography (PET) in the clinical management of glioblastoma. Some phase I–II clinical trials of dietary Met restriction in association with chemotherapeutic drugs were carried out in patients with solid tumors and demonstrated feasibility, good safety, and good compliance of the Met-free diet on short period (1–3 days every 2 weeks) [Citation35–37]. Differently, patients found it difficult to maintain sufficient energy needs and experienced weight loss, early satiety, and lack of appetite in a long-term study of Met restriction [Citation38]. A phase 2 clinical study (ClinicalTrials.gov Identifier: NCT03186937) is recruiting patients with breast cancer to confirm that MR enhances cell surface expression of TRAIL receptor-2 in triple-negative breast cancer. The overall aim of this study is to establish the feasibility and acceptability of the dietary intervention, which is based on methionine-free amino acid-modified medical food (Hominex-2, Abbott Nutrition) up to 3 weeks.
An alternative approach to dietary MR, as a more effective and rapid method to deplete serum methionine, might be the use of methionine gamma-lyase (MGL) [EC 4.4.1.11], a non-mammalian pyridoxal 5ʹ Phosphate (PLP)-dependent enzyme that metabolizes Met to alpha-ketobutyrate, ammonia, and thiols. Although MGL has been isolated from bacteria, fungi, and protozoa, the one which has been purified from Pseudomonas putida has shown the better kinetic properties and thus its recombinant form is among those more extensively tested in vitro and in vivo for tumor growth inhibitory efficacy [Citation39,Citation40]. As MGL exhibits low bioavailability due to its short biological half-life and rapid clearance, a PEGylated enzyme (PEG-MGL) has been used for in vivo studies [Citation41,Citation42]. However, several problems remain to be solved: a) frequent injections of PEG-PAL are still necessary for maintaining a depletion >50% of the physiological value [Citation41]; b) known PEG ability to elicit immune response; c) low bioavailability of enzyme cofactor PLP due to its rapid binding to plasma proteins. Again, most of these problems could be solved by administering the therapeutic enzyme confined into erythrocytes. Interestingly, a preclinical study [Citation43] in mice has demonstrated the enhanced pharmacokinetic properties of the encapsulated MGL from P. putida (erymet) compared to the free form and its efficacy against tumor growth when administered together with pyridoxine (PN), one B6 vitamer rapidly converted to PLP by erythrocytes [Citation44]. More in detail, NMRI nude mice were xenografted with U-87 MG-luc2 glioblastoma cells and were then treated with a weekly single IV injection of erymet for 5 weeks in association with daily PN supplemented by oral gavage. Overall, Met plasma level was decreased by 75% after the five cumulative erymet injections and the average absolute tumor volume was reduced by 85%. A parallel toxicity study in CD1 mice revealed moderate side effects (i.e. a score of <2) in 79% of mice treated with erymet plus PN compared to only 8% in the control group; however, the majority of them were reversed during the recovery period.
Furthermore, a preclinical study on mice bearing orthotopic EMT-6 syngeneic breast carcinoma revealed the potentiality for the combination of erymet with immune checkpoint inhibition [Citation45]. A significant inhibition of tumor growth was observed when infused with erythrocyte-encapsulated methionine-γ-lyase from P. putida at a dose of 60 U/kg and in combination with antimouse PD-1 antibody, compared to the separate drugs. Survival time was 35 days for the combined therapy, compared to 23 days for the separate one.
However, it is noteworthy that MGL from P. putida, a parasitic and potentially pathogenic microorganism, possesses the ability to partially catabolize L-cysteine [Citation46]; hence, it would be desirable to carry on preclinical studies where a MGL from safer origin than P. putida and with a different specificity can be loaded inside RBCs to preserve cysteine plasma levels. MGL-BL929 from Brevibacterium aurantiacum [Citation47], a safe microorganism abundantly present in food, could be a possible candidate to this extend.
3.2. Asparaginase as cancer therapy
Besides methionine restriction as anticancer strategy, asparagine (ASN) depletion has proved to be an extremely effective tool for the treatment of several type of cancers and it is noteworthy that asparaginase-loaded RBCs represent one of the most clinically advanced applications for the treatment of acute lymphoblastic leukemia (ALL). Indeed, certain neoplastic tissues, including ALL cells, express a significantly lower level of asparagine synthetase than most human tissues and thus have to rely solely on extracellular source of ASN to maintain protein synthesis. Therefore, systemic depletion of ASN would lead to cancer cells death through important cellular dysfunction. For this reason, the enzyme asparaginase (EC 3.5.1.1) from E. coli and Erwinia chrysanthemi, which is able to hydrolyze ASN to L-aspartic acid and ammonia, has been in clinical use for ALL since the early 1970s. However, its use was associated with liver and pancreas toxicity and high immunogenicity. In addition, given the fact that the enzymes have a short circulatory half-life (ranging between 8 and 30 hours), frequent infusions were needed [Citation48]. PEGylated formulations of the therapeutic enzyme were developed to overcome these limitations and were firstly used in clinical trials in the 1980s. However, the administration of PEGylated asparaginase was associated with hepatotoxicity, pancreatitis, and thrombosis [Citation49], paving the way for alternative delivery strategies. Again, the possibility to administer the enzyme confined into RBCs has been explored in animal models. The first in vivo studies were carried out in monkeys by Updike et al. and showed that circulating asparaginase activity was several orders of magnitude higher in the monkeys injected with RBC-loaded asparaginase than in animals injected with native asparaginase. In addition, suppression of the serum asparagine level occurred after 20 days, which was twice as long as the suppression in the control monkeys [Citation50,Citation51]. Similar results were obtained in Swiss mice by Kravtzoff et al. [Citation52], which demonstrated that asparaginase loaded into erythrocytes was far more effective in eliminating plasma asparagine compared to the same dose of free asparaginase injected in solution, during a sustained period of 14 days. Interestingly, asparaginase-loaded RBCs were efficient in improving the mean survival time of DBA/2 mice bearing mouse lymphoma cell L5178Y by ≈ 44% compared to the saline group [Citation53]. Altogether, these preclinical studies provided the basis for the development of phase I/II clinical studies by Erytech Pharma to investigate the safety and efficacy of erythrocytes encapsulated asparaginase (GRASPA) for the treatment of human ALL [Citation54]. More recently, erythrocytes encapsulated with asparaginase (relabeled as eryaspase) have been evaluated for other oncology indications such as pancreatic cancer and triple-negative breast cancer. A phase III study in patients with ductal adenocarcinoma of the pancreas is currently active and recruiting; its primary outcome is to evaluate if the addition of eryaspase to chemotherapy improves overall survival when compared to chemotherapy alone (https://clinicaltrials.gov/ct2/show/NCT03665441). In parallel, a phase II/III trial is ongoing on patients with selected metastatic triple-negative breast cancer, with the aim to evaluate the efficacy of eryaspase in combination with gemcitabine and carboplatin chemotherapy, compared to chemotherapy alone, as a first-line treatment (https://clinicaltrials.gov/ct2/show/NCT03674242).
3.3. Arginine deiminase as cancer therapy
Another interesting microbial enzyme for deprivation of amino acid metabolism in malignant cells is the prokaryotic arginine deiminase (ADI, EC 3.5.3.6), a hydrolase that metabolizes L-arginine to citrulline and ammonia. Arginine represents a precursor of important molecules such as nitric oxide, polyamines, nucleotides, proline, and glutamate, and it is involved in several biosynthetic pathways able to influence carcinogenesis [Citation55]. It is noteworthy that a number of tumors, such as hepatocellular carcinoma (HCC) and malignant melanoma, exhibit arginine auxotrophy due to the variable loss or downregulation of argininosuccinate synthetase, an enzyme involved in arginine synthesis [Citation56]. Therefore, the depletion of exogenous arginine through ADI administration could be used as a treatment for these kinds of tumors, which are strictly dependent on this amino acid for their survival, in opposition to what happens in normal cells, which are able to prevent the loss being able to normally synthesize arginine. The antitumor activity of ADI can be ascribed to inhibition of protein synthesis, suppression of angiogenesis, and induction of the apoptotic pathway [Citation57]. ADI purified from Mycoplasma has a short half-life (≈ 4 h) in the circulation and was found to be highly immunogenic. Therefore, as mentioned several times in this review, a PEGylated form of the enzyme has been developed in order to increase its half-life and to limit its immunogenicity. Actually, ADI-PEG20, PEGylated recombinant Mycoplasmal ADI, has already completed either a phase III clinical trial for patients with advanced HCC (NCT01287585) and various phase II clinical trials for unresectable HCC (NCT02006030) and melanoma (NCT00450372), while phase II clinical trials for the treatment of soft tissue sarcoma are in progress (NCT03449901). All these clinical trials are sponsored by Polaris group (Cayman Islands), the leading company in the establishment of ADI as antitumor-drug. Importantly, it was reported that ADI-PEG20 treatment in mesothelioma patients produces anti-ADI-PEG20 neutralizing antibodies by the fourth week, thereby resisting drug usage [Citation58]. Again, encapsulation of ADI into RBCs could be a promising alternative to improve the half-life and to reduce the immunogenicity of the protein. Indeed, preclinical studies carried out by Erytech Phasma showed that injections of ADI-loaded erythrocytes into CD-1 mice (5.5 IU/mL) reduced plasmatic arginine level to 30% of control values over a period of 5 days. The same dose of free ADI strongly depleted arginine (<5 μM) for 24 hours but its plasma levels returned to baseline by 2.5 days. Higher dose (10.4 IU/mL) resulted in the complete depletion of plasmatic arginine over the 5-day period of the study without causing tolerability problems [Citation59]. Anyway, to date there is no evidence of preclinical efficacy of this therapeutic strategy in cancer animal models; differently, ADI-loaded RBCs have been shown to be efficient in a preclinical model for Arginase-1 deficiency, where promising results been recently generated (www.erytech.com).
Interestingly, deprivation of amino acid metabolism by microbial enzymes as a promising biological strategy for cancer treatment has been recently reviewed [Citation60].
4. Phenylketonuria
Phenylketonuria (PKU) is the most common hereditary disorder of amino acid metabolism among Caucasians (overall incidence 1:10,000). It is caused by a deficiency in the enzyme phenylalanine hydroxylase (PAH; EC 1.14.16.1) (OMIM*612349) that converts L-phenylalanine (Phe) into L-tyrosine, resulting in the accumulation of neurotoxic levels of Phe and severe mental retardation. Genetic screening identifies newborns with PKU who are then immediately placed on a dietary restriction of Phe intake, thus preventing mental disability. However, some specific deficits in the higher-order cognitive processes and frequent psychopathological symptoms can be observed even in early and continuously treated PKU patients [Citation61,Citation62]. Moreover, a survey of patients with PKU reported that only 23% of adult patients [Citation63] were able to maintain blood Phe within the therapeutic range of 120 to 360 µmol/L.
Recently, FDA (May 2018) and EMA (April 2019) approved an enzyme-replacement therapy based on subcutaneous administration of Pegvaliase (PalynziqTM, BioMarin Pharmaceutical Inc.), a recombinant Anabaena variabilis phenylalanine ammonia-lyase (PAL) conjugated with polyethylene glycol (PEG) which is able to metabolize Phe to trans-cinnamic acid and ammonia. A phase III clinical trial showed the efficacy of this treatment in reducing blood Phe (≤360 µM) in 60% of adult treated patients but, at the same time, it revealed the presence of antibodies against PAL and PEG which caused adverse reactions and anaphylaxis [Citation64].
These limitations could be easily overcome by delivering the therapeutic enzyme through RBCs. In fact, the PEGylation step, carried out in order to reduce enzyme immune recognition and improve its pharmacodynamic stability, can be avoided since the foreign enzyme is confined inside the erythrocytes, allowing it to be hidden from the immunological system and thus from any neutralizing antibodies that may be present in circulation. The feasibility of this strategy has been well documented in preclinical studies both in normal mice and in a validated murine model of PKU (BTBR-Pahenu2 mice). At first, Yew et al. [Citation65] showed that RBCs encapsulated with PAH from the cyanobacterium Chromobacterium violaceum, a more thermally stable and more active enzyme than the mammalian one, were able to decrease Phe levels by 80% in normal mice when injected into the bloodstream. Moreover, the encapsulated enzyme remained active for at least 10 days vs for less than 1 h of free PAH and exhibited improved pharmacokinetics, which resulted in a better persistence in the circulation. Unfortunately, the same strategy was ineffective in lowering Phe levels in PKU mice, probably due to low specific activity of the enzyme (0.3 U/mg protein) and to the large amount of substrate into the circulation, that is 10–20 times higher than that of normal mice. However, these results demonstrated the ability of engineered RBCs to act as Phe metabolizing bioreactors once a specific un-PEGylated enzyme is loaded inside them. This claim was subsequently confirmed by our study [Citation66], wherein a long time reduction (10 weeks) of Phe levels in adult BTBR-Pahenu2 mice has been reached by repeated IV injections of PAL-loaded RBCs at 9–10 day-intervals, which maintained blood Phe at significantly lower levels than their starting conditions. PAL was from the BioMarin clinical manufacturing group and possessed a specific activity of at least five times higher compared to that of PAH previously mentioned and, moreover (differently from PAH), it did not require the presence of sufficient levels of the essential cofactor tetrahydrobiopterin. Furthermore, in our study, animals received about 20 times more enzymatic units than in the Yew’s one, thus validating the efficacy of PAL-loaded RBCs in reducing Phe levels in PKU mice. In addition, it is noteworthy that the presence of antibodies against PAL did not modify the ability of PAL-loaded RBCs to decrease blood Phe levels despite their numerous and frequent administrations. More recently, the same strategy has been proven as effective in preventing intellectual disability in developing PKU mice [Citation67]. In this preclinical study, BTBR-Pahenu2 mice received weekly infusions of PAL-loaded RBCs from 15 to 64 post-natal days and specific biochemical, behavioral, neurochemistry and morphology parameters, known markers of PKU mice diseases, have been examined in comparison with untreated PKU and wild-type mice. Following the treatment with the engineered RBCs, animals showed normal levels of blood and brain Phe for the whole period examined and, moreover, they exhibited improvements in cognitive performance, brain serotonin content, dendritic spine morphology, and myelination. Again, no inactivating immune reaction or adverse events were observed. These recent results have demonstrated the efficacy of an early treatment with repeated PAL-loaded RBCs injections to prevent the rise of the clinical phenotype associated with untreated PKU. We are confident that our preclinical studies on BTBR-Pahenu2 mice can constitute the basis for the set-up of a clinical trial in order to prove the safety and efficacy of PAL-loaded RBC injections in PKU patients. This belief is also due to the successful clinic results obtained so far in different trials from phase I to phase III, in which RBCs were used as drug delivery system.
5. Alcohol detoxification
Alcohol use is recognized as a leading risk factor for disease burden worldwide. Globally, alcohol use is the seventh leading risk factor for death and disability-adjusted life years (DALYs) accounting for nearly 10% of global deaths among populations aged 15–49 years [Citation68]. It has also been estimated that from 2019 to 2040, 1,003,400 (95% CI 896,800–1,036,200) people are projected to die from alcohol-related liver disease in the USA alone [Citation69]. Furthermore, the use of alcohol disproportionately affects people in low human development index (HDI) countries and young people [Citation70]. Based on these simple premises it became immediately evident that actions are urgently needed and alcohol control policies should be implemented in all countries [Citation71]. In addition, the risk for alcohol use disorders (AUD) has been also associated with risk genes. Recently, a meta-analysis of problematic alcohol use (PAU) has identified 29 independent risk variants, permitting a genetic correlation with substance abuse and psychiatric traits [Citation72]. While knowledge of the functional effects of the variants will eventually contribute to personalized treatment of PAU, permitting the identification of individuals who may be most treatment responsive, at the same time, this genetic variability makes more complex the effort in treating the alcohol use disorders. Fortunately, AUD are treatable and include psychosocial and pharmacologic interventions. FDA-approved medications comprise disulfiram, naltrexone, and acamprosate. Alcoholism, as other substance use disorders, is widely under-treated with less than 10% of individuals with AUD receiving treatment [Citation73]. Some years ago, the possibility of using red blood cells for alcohol detoxification was first introduced by our group [Citation2]. Based on the evidence that acetaldehyde (the primary oxidation product of ethanol generated by the action of the NAD-dependent alcohol dehydrogenase and by the microsomal ethanol oxidizing system) is the causal factor in many pathogenic processes, acetaldehyde dehydrogenase-loaded erythrocytes were produced and shown to be able to perform as bioreactors improving alcohol and acetaldehyde metabolism [Citation2] both in vitro and in vivo. These experiments were performed using a high affinity enzyme from Alcaligenes eutrophus, with an apparent Km similar to that of the mitochondrial enzyme. Several years later Lizano et al. [Citation74,Citation75] in attempts to increase alcohol consumption by enzyme-loaded erythrocytes, co-encapsulated both alcohol dehydrogenase and acetaldehyde dehydrogenase from yeast without a significant improvement in alcohol degradation with respect to the figures found using a single enzyme. Furthermore, Alexandrovich et al. [Citation76], using in vitro studies and mathematical modeling, concluded that the metabolic ability of enzyme-loaded erythrocytes to degrade ethanol is not limited by the amount of enzyme(s) loaded but rather by the NAD+/NADH ratio inside the cell, confirming a previous observation [Citation2]. It is noteworthy that several experiments were performed using a low-affinity acetaldehyde dehydrogenase rather than a high-affinity enzyme for acetaldehyde.
Alcohol oxidase (AlOx) from Pichia pastoris (a methylotrophic yeast) has a much higher affinity for methanol than for ethanol. Based on this observation, we investigate the potential use of this enzyme for the treatment of methanol intoxication [Citation77]. In fact, in humans, methanol is metabolized to formaldehyde by alcohol dehydrogenase and formaldehyde is metabolized to formic acid by acetaldehyde dehydrogenase, leading to cytochrome C oxidase inhibition. AlOx was encapsulated into human and murine erythrocytes up to 2 units/ml of packed cells. Enzyme‐loaded erythrocytes showed an increased rate of the hexose‐monophosphate‐shunt activity and a significant methemoglobin production resulting from the intracellular generation of H2O2. However, the in vivo survival of these cells did not seem to be significantly affected by methanol catabolism. In vivo, mice receiving AlOx‐loaded erythrocytes were able to keep the blood methanol concentrations below values that were about 50% of those found in control mice who received similar amounts of methanol. Thus, AlOx‐loaded erythrocytes may add an important contribution to the detoxification protocol against methanol poisoning. Formic acid can eventually be further degraded by encapsulate formate dehydrogenase [Citation78].
In conclusion, enzyme-loaded erythrocytes have been used at preclinical level to possibly treat several conditions that have a large impact and clear medical needs with limited therapeutic options.
6. Hyperammonemia
Ammonia is a byproduct of many metabolically important reactions but, at high levels, it is neurotoxic. In ureotelic animals, including humans, ammonia is maintained at low levels in blood (50–100 µM) mainly by its incorporation in urea through the hepatic urea cycle. Differently, in other tissues, including the brain (in which a functional urea cycle is absent), ammonia is mostly eliminated by glutamate and glutamine biosynthesis catalyzed by glutamate dehydrogenase (GDH) and glutamine synthetase, respectively. It is therefore understandable that if ammonia detoxification does not occur properly, as in several pathological conditions, hyperammonemia may arise. The most common situations causing hyperammonemia are chronic liver diseases (mainly liver cirrhosis) and congenital defects in enzymes of the urea cycle, both leading to hepatic encephalopathy, tremors, convulsions, until coma and death [Citation79]. Treatments to reduce ammonia levels include a combination of a) restriction of dietary protein in order to mitigate the production of nitrogenous waste together with a hypercaloric glucose-based solution to enhance anabolism; b) use of oral non-adsorbable disaccharides which decrease the production and absorption of ammonia in the intestine; c) intravenous infusion of sodium benzoate to directly remove ammonia in the form of hippurate; d) intravenous infusion of sodium phenylacetate to eliminate ammonia as phenylacetylglutamine; e) intravenous infusion of arginine that becomes an essential amino acid in patients with urea cycle defects, while stimulates the urea cycle in other pathological conditions; f) hemodialysis, when ammonia levels do not fall after 8 hours of continual treatment. Intermittent hemodialysis is the most efficient method to reduce plasma ammonia levels; however, it leads to hypotension and, furthermore, ammonia levels tend to rebound shortly after discontinuation [Citation80]; thus, a continuous renal replacement therapy (CRRT) has been recently proposed [Citation81]. Nevertheless, as reported in [Citation82], despite improvement in renal replacement therapy and intensive care, the mortality associated with neonatal hyperammonemia remains high. Moreover, the ammonia level corrections using the known medications above mentioned are not always sufficiently effective, since a decrease in ammonia concentrations to normal level in patient’s blood usually requires 2–10 days [Citation83–86]; this period of time is, in some cases, too long to avert the damages caused by hyperammonemia. Therefore, it is comprehensible the necessity to find more effective therapeutic strategies capable of reducing blood ammonia levels for longer times. Enzymes able to catalyze reactions in which ammonia can be transferred to other molecules may represent a possible alternative to the commonly used therapies. In addition, RBCs could be used as bioreactors for the dissemination of the therapeutic protein in circulation, with the well-known advantages already discussed. The enzyme L-glutamate dehydrogenase (GDH, EC. 1.4.1.3), which catalyzes the formation of L-glutamic acid from α-ketoglutarate and ammonia in the presence of NADPH, was the first enzyme tested in a preclinical study on hyperammonaemic mice [Citation87]. Hyperammonemia was induced in Swiss CD1 mice by IP injections of urease (33 U/Kg BW), which lead to a significant increase in the ammonia level compared to the basal level (50–80 µM) from 1 h (1332.7 ± 44 µM) to 25 h (135.4 ± 10.1 µM). Mice receiving GDH-loaded RBCs consumed 50% and 75% of ammonia, respectively, 2 h and 5 h after the urease injection. Differently, in control mice receiving washed RBCs, ammonia remained unchanged 2 h after urease injections while 50% was still present after 5 h. At 24 h, no significant differences between the 2 groups could be detected. Successively, another group [Citation88] has assessed the efficacy in ammonia removal of erythrocytes loaded with glutamine synthetase (GS, EC. 6.3.1.2), the enzyme catalyzing the formation of L-glutamine starting from L-glutamic acid and ammonia in the presence of ATP, in hyperammonaemic Swiss mice. To increase the intracellular content of L-glutamic acid, allowing more substrate to form L-glutamine and eliminate ammonia, this amino acid was co-encapsulated with GS during the loading procedure. Mice received loaded RBCs immediately before hyperammonemia induction by acute IP injection of ammonium acetate (2.5 mmol/kg BW), a concentration that can induce a sufficient increase in blood ammonia concentration at least within 4 h. Blood ammonia levels were evaluated at 30, 60 and 120 min post hyperammonemia induction and values significantly lower (0.67 ± 0.26; 0.59 ± 0.08; 0.27 ± 0.13 mM, respectively) compared to control mice which received unloaded RBCs (1.02 ± 0.17; 0.83 ± 0.03; 0.63 ± 0.05 mM, respectively) were obtained. However, in both the cited studies, enzyme-loaded RBCs worked only for a short period of time, after which blood ammonia concentration decreased approximately at the same rate in treated and control hyperammonaemic mice. Recently [Citation89], it has been supposed that the poor efficiency and duration in the performances of these bioreactors could be due to the low permeability of RBC membranes to α-ketoglutarate and L-glutamic acid. Following these assumptions, an interesting mathematical model allowing to analyze the efficiency of a new enzyme system to load into RBCs has been developed and tested in hyperammonaemic mice. It is reasonable to think that α-ketoglutarate and L-glutamic acid may be almost completely depleted by reactions occurred into GDH- and GS-loaded RBCs, respectively. In addition, L-glutamic acid could accumulate in GDH-loaded RBCs, causing a shift in the equilibrium of the reaction and leading to ammonia production. To bypass these obstacles, the Authors proposed the co-entrapment in RBCs of GDH and alanine aminotransferase (AAT), thus creating a metabolic pathway where α-ketoglutarate and L-glutamic acid were produced and consumed cyclically. The efficacy of these new bioreactors was evaluated in Swiss mice receiving GDH-AAT-loaded RBCs immediately before the injection of ammonium acetate (2.5 mmol/kg BW). The treatment has been able to remove blood ammonia at the rate of 2.0 mmol/h/L RBCs; unfortunately, this was only a proof of concept, given that the result was obtained by injecting a large volume of engineered cells and was limited to a total time of 2 h. However, as smartly discussed by the Authors, the efficiency of the proposed strategy could be greatly improved by using GDH from other biological sources different than the bovine one, which has a high molecular weight and easily undergone to polymerization, leading to a very efficient system able to remove ammonia in a rate up to 10 times higher than common medications.
7. Expert opinion
Enzyme delivery is a medical need for several conditions, including the treatment of metabolic diseases, the treatment of acute or chronic assumption of alcohol and the treatment of certain forms of cancer. The key requirements for an effective benefit in the usage of therapeutic enzymes are listed below and, among the other, we find that the drug must be specific for the bioconversion of the target substrate, must be stable, must be retained in circulation, and has no or limited immunogenicity. These conditions are not easily obtainable and frequently one achievement is obtained at the expense of another relevant property. The selection of the therapeutic enzyme usually benefits from the genetic variability found in a number of living organisms and the selected enzyme may be advantageously optimized by protein engineering. This approach was successful in several cases. We currently have therapeutic enzymes that derive from genes present in algae, bacteria and in other living organisms but not in the human genome. A far more complex issue is the handling of immunogenicity. In fact, many therapeutic enzymes have been approved by the FDA and other regulatory agencies by the inclusion of a black box highlighting the risk of anaphylaxis and guidelines that have to be strictly followed through drug titration, administration in the presence of an expert clinician and other mandatory procedures. To overcome this critical issue experienced by several therapeutic enzymes, the protein is commonly PEGylated. PEGylation apparently reduce immunogenicity and protect the therapeutic protein from the inactivation by anti-drug antibodies but, in some cases, patients have also developed anti-PEG antibodies.
Red blood cells provide several comparative advantages over other modalities of therapeutic enzymes administration. Among the other we find a long circulation time (usually administered with a schedule of once-a-month), a reduced immunogenicity, they do not need further chemical modifications (i.e. by PEGylation) and, even in case of antibody induction in vivo, the enzyme remains active (i.e. is not inactivated) because it is protected by the red blood cell membrane.
A number of papers and excellent reviews have highlighted the advantages and the limits of this approach. In this paper, we have focused on few selected examples that illustrate the progress at preclinical level. We have previously discussed the use of enzyme-loaded red blood cells in current clinical applications [Citation6]. It is noteworthy that many other papers have provided data in vitro and in vivo and discussed alternative modalities to obtain enzyme-expressing red blood cells (i.e. by lentiviral transfection of immature red cells precursors) [Citation9], but the examples here discussed were selected because, in our opinion, can be informative about the steps necessary not only to confirm that a selected application works also in vivo but also because they have been developed to collect a significant piece of information that is necessary before planning a formal clinical development program. The reader will find information on preclinical progress in different fields that could be representative of the many areas were enzyme-loaded red blood cells can have a place. Finally, we would like to mention that enzymes targeted to selected red blood cell membrane proteins by fusion with single-chain fragment antibodies could provide an alternative to enzyme-loading for selected applications [Citation90].
Disclosure statement
M. Magnani and L. Rossi hold shares in EryDel SpA.
References
- Ihler GM, Glew RH, Schnure FW. Enzyme loading of erythrocytes. Proc Natl Acad Sci USA. 1973;70(9):2663–2666. DOI:10.1073/pnas.70.9.2663.
- Magnani M, Laguerre M, Rossi L, et al. In vivo accelerated acetaldehyde metabolism using acetaldehyde dehydrogenase-loaded erythrocytes. Alcohol Alcohol. 1990;25(6):627–637.
- Bourgeaux V, Aufradet E, Campion Y, et al. Efficacy of homologous inositol hexaphosphate-loaded red blood cells in sickle transgenic mice. Br J Haematol. 2012;157:357–369.
- Bax BE, Bain MD, Talbot PJ, et al. Survival of human carrier erythrocytes in vivo. Clin Sci. 1999;96(2):171–178.
- Coker SA, Szczepiorkowski ZM, Siegel AH, et al. A study of the pharmacokinetic properties and the in vivo kinetics of erythrocytes loaded with dexamethasone sodium phosphate in healthy volunteers. Transfus Med Rev. 2018;32(2):102–110.
- Rossi L, Pierigè F, Aliano MP, et al. Ongoing developments and clinical progress in drug-loaded red blood cell technologies. BioDrugs. 2020;34:265–272.
- DeLoach JR. Hypotonic dialysis encapsulation in erythrocytes of mammalian species. Bibl Haematol. 1985;51:1–6.
- Pierigè F, Bigini N, Rossi L, et al. Reengineering red blood cells for cellular therapeutics and diagnostics. WIREs Nanomed Nanobiotechnol. 2017;9:e1454.
- Bax EB. Erythrocytes as carriers of therapeutic enzymes. Pharmaceutics. 2020;12:435–455. .
- Pei L, Omburo G, McGuinn WD, et al. Encapsulation of phosphotriesterase within murine erythrocytes. Toxicol Appl Pharmacol. 1994;124:296–301.
- Leung P, Cannon EP, Petrikovics I, et al. In vivo studies on rhodanese encapsulation in mouse carrier erythrocytes. Toxicol Appl Pharmacol. 1991;110:268–274.
- Pei L, McGuinn WD, Omburo G, et al. Spectrophotometric determination of paraoxonase within mouse carrier red blood cells. Biotechnol Appl Biochem. 1994;20(Pt1):35–41.
- Bustos NL, Batlle AM. Enzyme replacement therapy in Porphyrias–V. In vivo correction of delta-aminolaevulinate dehydratase defective in erythrocytes in lead intoxicated animals by enzyme-loaded red blood cell ghosts. Drug Des Deliv. 1989;5:125–131.
- Ito Y, Ogiso T, Iwaki M, et al. Encapsulation of human urokinase in rabbit erythrocytes and its disposition in the circulation system in rabbits. J Pharmacobiodyn. 1987;10:550–556.
- Flynn G, Hackett TJ, McHale L, et al. Encapsulation of the thrombolytic enzyme, brinase, in photosensitized erythrocytes: a novel thrombolytic system based on photodynamic activation. J Photochem Photobiol B Biol. 1994;26:193–196.
- Muzykantov VR, Sakharov DV, Smirnov MD, et al. Immunotargeting of erythrocytes-bound streptokinase provides local lysis of a fibrin clot. BBA Gen Subj. 1986;884:355–362.
- Murciano JC, Medinilla S, Eslin D, et al. Prophylactic fibrinolysis through selective dissolution of nascent clots by tPA-carrying erythrocytes. Nat Biotechnol. 2003;21:891–896.
- Adriaenssens K, Karcher D, Marescau B, et al. Hyper-argininemia: the rat as a model for the human disease and the comparative response to enzyme replacement therapy with free arginase and arginase-loaded erythrocytes in vivo. Int J Biochem. 1984;16:779–786.
- Thorpe SR, Fiddler MB, Desnick RJ, et al. In Vivo Fate of Erythrocyte-Entrapped β-Glucuronidase in β- Glucuronidase-Deficient Mice. Pediatr Res. 1975;9:918–923.
- Magnani M, Mancini U, Bianchi M, et al. Comparison of uricase-bound and uricase-loaded erythrocytes as bioreactors for uric acid degradation. Adv Exp Med Biol. 1992;326:189–194.
- Zocchi E, Benatti U, Guida L, et al. Encapsulation of glucose oxidase in mouse erythrocytes: an experimental model of oxidant-induced cytotoxicity and a means for splenic targeting of carrier erythrocytes. In: Ropars C, Chassaigne M, Nicolau C, editors. Red blood cells as carriers for drugs. Oxford (UK): Pergamon Press; 1987:95–101.
- Rossi L, Bianchi M, Magnani M. Increased glucose metabolism by enzyme-loaded erythrocytes in vitro and in vivo normalization of hyperglycemia in diabetic mice. Biotechnol Appl Biochem. 1992;15:207–216.
- Garin M, Rossi L, Luque J, et al. Lactate catabolism by enzyme-loaded red blood cells. Biotechnol Appl Biochem. 1995;22:295–303.
- Naqi A, DeLoach JR, Andrews K, et al. Determination of parameters for enzyme therapy using L-asparaginase entrapped in canine erythrocytes. Biotechnol Appl Biochem. 1988;10:365–372.
- Moran NF, Bain MD, Muqit MM, et al. Carrier erythrocyte entrapped thymidine phosphorylase therapy for MNGIE. Neurology. 2008;71:686–688.
- Bax BE, Bain MD, Scarpelli M, et al. Clinical and biochemical improvements in a patient with MNGIE following enzyme replacement. Neurology. 2013;81:1269–1271.
- Levene M, Bain MD, Moran NF, et al. Safety and efficacy of erythrocyte encapsulated thymidine phosphorylase in mitochondrial neurogastrointestinal encephalomyopathy. J Clin Med. 2019;8(4):457.
- Bax BE, Levene M, Bain MD, et al. Erythrocyte encapsulated thymidine phosphorylase for the treatment of patients with mitochondrial neurogastrointestinal encephalomyopathy: study protocol for a multi-centre, multiple dose, open label trial. J Clin Med. 2019;8(8):1096.
- Levene M, Coleman DG, Kilpatrick HC, et al. Preclinical toxicity evaluation of erythrocyte-encapsulated thymidine phosphorylase in BALB/c mice and beagle dogs: an enzyme-replacement therapy for mitochondrial neurogastrointestinal encephalomyopathy. Toxicol Sci. 2013;131:311–324.
- Wanders D, Hobson K, Ji X. Methionine restriction and cancer biology. Nutrients. 2020;12(3):684.
- Kang JS. Dietary restriction of amino acids for Cancer therapy. Nutr Metab. 2020;17(1):1–12.
- Poirson-Bichat F, Goņalves RAB, Miccoli L, et al. Methionine depletion enhances the antitumoral efficacy of cytotoxic agents in drug-resistant human tumor xenografts. Clin Cancer Res. 2000;6(2):643–653.
- Kokkinakis DM, Hoffman RM, Frenkel EP, et al. Synergy between methionine stress and chemotherapy in the treatment of brain tumor xenografts in athymic mice. Cancer Res. 2001;61(10):4017–4023.
- Gao X, Sanderson SM, Dai Z, et al. Dietary methionine links nutrition and metabolism to the efficacy of cancer therapies. Nature. 2019;572:397–401.
- Thivat E, Durando X, Demidem A, et al. A methionine-free diet associated with nitrosourea treatment down-regulates methylguanine-DNA methyl transferase activity in patients with metastatic cancer. Anticancer Res. 2007;27(4 C):2779–2783.
- Durando X, Farges MC, Buc E, et al. Dietary methionine restriction with FOLFOX regimen as first line therapy of metastatic colorectal cancer: a feasibility study. Oncology. 2010;78:205–209.
- Thivat E, Farges MC, Bacin F, et al. Phase II trial of the association of a methionine-free diet with cystemustine therapy in melanoma and glioma. Anticancer Res. 2009;29(12):5235–5240.
- Epner DE, Morrow S, Wilcox M, et al. Nutrient intake and nutritional indexes in adults with metastatic cancer on a phase I clinical trial of dietary methionine restriction. Nutr Cancer. 2002;42(2):158‐166.
- Machover D, Zittoun J, Broët P, et al. Cytotoxic synergism of methioninase in combination with 5-fluorouracil and folinic acid. Biochem Pharmacol. 2001;61(7):867–876.
- Hoffman RM. Development of recombinant methioninase to target the general cancer-specific metabolic defect of methionine dependence: a 40-year odyssey. Expert Opin Biol Ther. 2015;15(1):21–31.
- Sun X, Yang Z, Li S, et al. In vivo efficacy of recombinant methioninase is enhanced by the combination of polyethylene glycol conjugation and Pyridoxal 5′-phosphate supplementation. Cancer Res. 2003;63(23):8377–8383.
- Yang Z, Sun X, Li S, et al. Circulating half-life of PEGylated recombinant methioninase holoenzyme is highly dose dependent on cofactor pyridoxal-5′-phosphate. Cancer Res. 2004;64(16):5775–5778.
- Gay F, Aguera K, Sénéchal K, et al. Methionine tumor starvation by erythrocyte-encapsulated methionine gamma-lyase activity controlled with per Os Vitamin B6. Cancer Med. 2017;6:1437–1452.
- Anderson BB, Fulford-Jones CE, Child JA, et al. Conversion of vitamin B6 compounds to active forms in the red blood cell. J Clin Invest. 1971;50(9):1901–1909.
- Sénéchal K, Maubant S, Leblanc M, et al. Abstract 2258: erymethionase (Methionine-Gamma-Lyase Encapsulated into Red Blood Cells) Potentiates Anti-PD-1 therapy in TNBC syngeneic mouse model. In Cancer Research. Vol. 79. American Association for Cancer Research (AACR): Philadelphia (PA); 2019. p. 2258.
- Machover D, Bonnarme P. Polypeptides isolated from Brevibacterium aurantiacum and their use for the treatment of cancer. WO Patent App. PCT/EP2012/061,497; 2012.
- Machover D, Rossi L, Hamelin J, et al. Effects in cancer cells of the recombinant l-methionine gamma-lyase from brevibacterium aurantiacum. Encapsulation in human erythrocytes for sustained l-methionine elimination. J Pharmacol Exp Ther. 2019;369(3):489–502.
- Wriston JC, Yellin TO. L-Asparaginase: A review. Advances in enzymology and related areas of molecular biology. Vol. 39. Hoboken (NJ): Wiley; 2006. p. 185–248.
- Heo YA, Syed YY, Keam SJ. Pegaspargase: A review in acute lymphoblastic leukaemia. Drugs. 2019;79:767–777.
- Updike SJ, Wakamiya RT. Infusion of red blood cell-loaded asparaginase in monkey. Immunologic, metabolic, and toxicologic consequences. J Lab Clin Med. 1983;101(5):679–691.
- Kravtzoff R, Colombat PH, Desbois I, et al. Tolerance evaluation of L-asparaginase loaded in red blood cells. Eur J Clin Pharmacol. 1996;51(3–4):221–225.
- Kravtzoff R, Ropars C, Laguerre M, et al. Erythrocytes as carriers for L-asparaginase. Methodological and mouse in-vivo studies. J Pharm Pharmacol. 1990;42(7):473–476.
- Kwon YM, Chung HS, Moon C, et al. L-asparaginase encapsulated intact erythrocytes for treatment of acute lymphoblastic leukemia (ALL). J Control Release. 2009;139:182–189.
- Hunault-Berger M, Leguay T, Huguet F, et al. Group for Research on Adult Acute Lymphoblastic Leukemia (GRAALL). A Phase 2 Study of L-Asparaginase Encapsulated in erythrocytes in elderly patients with philadelphia chromosome negative acute lymphoblastic leukemia: the GRASPALL/GRAALLSA2-2008 study. Am J Hematol. 2015;90::811–818.
- Patil MD, Bhaumik J, Banerjee UC, et al. Arginine dependence of tumor cells: targeting a chink in cancer’s armor. Oncogene. 2016;35:4957–4972.
- Xiong L, Teng JLL, Botelho MG, et al. Arginine metabolism in bacterial pathogenesis and cancer therapy. Int J Mol Sci. 2016;17:363–381.
- Kuo MT, Savaraj N, Feun LG. Targeted cellular metabolism for cancer chemotherapy with recombinant arginine-degrading enzymes. Oncotarget. 2010;1(4):246–251.
- Szlosarek PW, Luong P, Phillips MM, et al. Metabolic response to pegylated arginine deiminase in mesothelioma with promoter methylation of argininosuccinate synthetase. J Clin Oncol. 2013;31(7):e111–e113.
- Gay F, Aguera K, Senechal K, et al. Abstract 4812: arginine deiminase loaded in erythrocytes: a promising formulation for L-Arginine deprivation therapy in cancers. In Cancer Research. Vol 6. American Association for Cancer Research (AACR): Philadelphia (PA); 2016. p. 4812.
- Dhankhar R, Gupta V, Kumar S, et al. Microbial enzymes for deprivation of amino acids metabolism in malignant cells: biological strategy for cancer treatment. Appl Microbiol Biotechnol. 2020;104:2857–2869.
- Palermo L, Geberhiwot T, MacDonald A, et al. Cognitive outcomes in early-treated adults with phenylketonuria (PKU): a comprehensive picture across domains. Neuropsychology. 2017;31(3):255–267.
- Manti F, Nardecchia F, Chiarotti F, et al. Psychiatric disorders in adolescent and young adult patients with phenylketonuria. Mol Genet Metab. 2016;117(1):12–18.
- Brown CS, Lichter-Konecki U. Phenylketonuria (PKU): A problem solved?. Mol Genet Metab Rep. 2015;6:8–12.
- Gupta S, Lau K, Harding CO, et al. Association of immune response with efficacy and safety outcomes in adults with phenylketonuria administered pegvaliase in phase 3 clinical trials. EBioMedicine. 2018;37:366–373.
- Yew NS, Dufour E, Przybylska M, et al. Erythrocytes encapsulated with phenylalanine hydroxylase exhibit improved pharmacokinetics and lowered plasma phenylalanine levels in normal mice. Mol Genet Metab. 2013;109:339–344.
- Rossi L, Pierigè F, Carducci C, et al. Erythrocyte-mediated delivery of phenylalanine ammonia lyase for the treatment of phenylketonuria in BTBR-Pahenu2mice. J Control Release. 2014;194:37–44.
- Pascucci T, Rossi L, Colamartino M, et al. A new therapy prevents intellectual disability in mouse with phenylketonuria. Mol Genet Metab. 2018;124(1):39–49.
- Griswold MG, Fullman N, Hawley C, et al. Alcohol use and burden for 195 countries and territories, 1990-2016: A systematic analysis for the Global Burden of Disease Study 2016. Lancet. 2018;392(10152):1015–1035.
- Julien J, Ayer T, Bethea ED, et al. Projected prevalence and mortality associated with alcohol-related liver disease in the USA, 2019-40: a modelling study. Lancet Public Health. 2020;5(6):e316–e323.
- Shield K, Manthey J, Rylett M, et al. National, regional, and global burdens of disease from 2000 to 2016 attributable to alcohol use: a comparative risk assessment study. Lancet Public Heal. 2020;5(1):e51–e61.
- Flor LS, Gakidou E. The burden of alcohol use: better data and strong policies towards a sustainable development. Lancet Public Heal. 2020;5(1):e10–e11.
- Zhou H, Sealock JM, Sanchez-Roige S, et al. Genome-wide meta-analysis of problematic alcohol use in 435,563 individuals yields insights into biology and relationships with other traits. Nat Neurosci. 2020;23:809–818.
- Fairbanks J, Umbreit A, Prakash Kolla B, et al. Evidenced-based pharmacotherapies for alcohol use disorder: clinical pearls. Mayo Clin Proc. 2020;95(9):1964–1977.
- Lizano C, Pérez MT, Pinilla M. Mouse erythrocytes as carriers for coencapsulated alcohol and aldehyde dehydrogenase obtained by electroporation in vivo survival rate in circulation, organ distribution and ethanol degradation. Life Sci. 2001;68:2001–2016.
- Lizano C, Sanz S, Luque J, et al. In vitro study of alcohol dehydrogenase and acetaldehyde dehydrogenase encapsulated into human erythrocytes by an electroporation procedure. Biochim Biophys Acta. 1998;1425(2):328–336.
- Alexandrovich YG, Kosenko EA, Sinauridze EI, et al. Rapid elimination of blood alcohol using erythrocytes: mathematical modeling and in vitro study. Biomed Res Int. 2017;2017:1–14.
- Magnani M, Fazi A, Mangani F, et al. Methanol detoxification by enzyme-loaded erythrocytes. Biotechnol Appl Biochem. 1993;18(3):217–226.
- Muthuvel A, Rajamani R, Manikandan S, et al. Detoxification of formate by formate dehydrogenase-loaded erythrocytes and carbicarb in folate-deficient methanol-intoxicated rats. Clin Chim Acta. 2006;367:162–169.
- Felipo V, Butterworth RF. Neurobiology of ammonia. Prog Neurobiol. 2002;67:259–279.
- Picca S, Dionisi-Vici C, Abeni D, et al. Extracorporeal dialysis in neonatal hyperammonemia: modalities and prognostic indicators. Ped Nephrol. 2001;16(11):862–867.
- Häberle J, Burlina A, Chakrapani A, et al. Suggested guidelines for the diagnosis and management of urea cycle disorder: first revision. J Inherit Metab Dis. 2019;42(86):1192–1230.
- Ames EG, Luckritz KE, Ahmad A. A retrospective review of outcomes in the treatment of hyperammonemia with renal replacement therapy due to inborn errors of metabolism. Pediatr Nephrol. 2020;35(9):1761–1769.
- Sharma BC, Sharma P, Lunia MK, et al. A randomized, double-blind, controlled trial comparing rifaximin plus lactulose with lactulose alone in treatment of overt hepatic encephalopathy. Am J Gastroenterol. 2013;108(9):1458–1463.
- Sushma S, Dasarathy S, Tandon RK, et al. Sodium benzoate in the treatment of acute hepatic encephalopathy: a double-blind randomized trial. Hepatology. 1992;16(1):138–144.
- Honda S, Yamamoto K, Sekizuka M, et al. Successful treatment of severe hyperammonemia using sodium phenylacetate powder prepared in hospital pharmacy. Biol Pharm Bull. 2002;25(9):1244–1246.
- Mizutani N, Kato T, Maehara M, et al. Oral administration of arginine and citrulline in the treatment of lysinuric protein intolerance. Tohoku J Exp Med. 1984;142(1):15–24.
- Sanz S, Lizano C, Luque J, et al. In vitro and in vivo study of glutamate dehydrogenase encapsulated into mouse erythrocytes by a hypotonic dialysis procedure. Life Sci. 1999;65:2781–2789.
- Kosenko EA, Venediktova NI, Kudryavtsev AA, et al. Encapsulation of glutamine synthetase in mouse erythrocytes: a new procedure for ammonia detoxification. Biochem Cell Biol. 2008;86:469–476.
- Protasov ES, Borsakova DV, Alexandrovich YG, et al. Erythrocytes as bioreactors to decrease excess ammonium concentration in blood. Sci Rep. 2019;9:1455.
- Glassman PM, Villa CH, Ukidve A, et al. Vascular drug delivery using carrier red blood cells: focus on RBC surface loading and pharmacokinetics. Pharmaceutics. 2020;12(5):440.