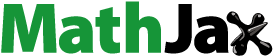
ABSTRACT
Introduction: Diabetes is a pandemic disease that causes relevant ocular pathologies. Diabetic retinopathy, macular edema, cataracts, glaucoma, or keratopathy strongly impact the quality of life of the patients. In addition to glycemic control, intense research is devoted to finding more efficient ocular drugs and improved delivery systems that can overcome eye barriers. Areas covered: The aim of this review is to revisit first the role of diabetes in the development of chronic eye diseases. Then, commercially available drugs and new candidates in clinical trials are tackled together with the pros and cons of their administration routes. Subsequent sections deal with self-assembled drug carriers suitable for eye instillation combining patient-friendly administration with high ocular bioavailability. Performance of topically administered polymeric micelles, liposomes, and niosomes for the management of diabetic eye diseases is analyzed in the light of ex vivo and in vivo results and outcomes of clinical trials. Expert opinion: Self-assembled carriers are being shown useful for efficient delivery of not only a variety of small drugs but also macromolecules (e.g. antibodies) and genes. Successful design of drug carriers may offer alternatives to intraocular injections and improve the treatment of both anterior and posterior segments diabetic eye diseases.
1. Introduction
Diabetes mellitus currently affects 8.5% people worldwide, and it is expected to impact on the lives of 570 million people in 2025 [Citation1]. There are five different forms of diabetes, with diabetes type 1 (failure in the production of insulin) and type 2 (deficient insulin sensitivity) being the most common. The other three forms are monogenic diabetes, which is hereditary due to a single gene mutation; gestational diabetes, related to pregnancy; and cystic fibrosis-related diabetes, which is linked to scarring of the pancreas that leads to insulin abnormalities. For people with type 1 diabetes, the immune system attacks pancreatic cells responsible for the production of insulin, disrupting their normal function. Both genetic and environmental factors have been identified as causal agents. Type 2 diabetes is indicative of insulin resistance, which may be caused by excess body weight.
Diabetes is considered a pandemic disease with an increasing morbidity and the highest rate in years of life lost due to disability in both high-income and lower-middle-income countries [Citation2]. Such a high incidence results in an increase in diseases secondary to diabetes. Diabetes-associated diseases range from cardiovascular problems to diabetic neuropathy including kidney failures and ocular diseases. Indeed, the term diabetic eye disease has a broad meaning as it may encompass multiple illnesses in different parts of the eye, mainly diabetic retinopathy, macular edema, cataracts, glaucoma, and keratopathy [Citation3–6].
Control of hyperglycemia is a critical main measure to avoid a fast progression of damage in ocular structures. Depending on the time lag between the first symptoms of diabetes and effective regulation of glycemia levels, the eyes may already be affected when therapeutic measurements are taken. Therefore, early diagnosis may prevent the apparition of diabetes-associated diseases. Nevertheless, continuous high basal glucose levels over time inevitably cause damage to a wide variety of tissues, especially those where glucose is freely accessible [Citation7].
Since diabetes is a chronic disease, ocular treatments may have to be applied for years. Therefore, finding drug delivery systems that combine the patient-friendly administration of topical formulations with the high ocular bioavailability of intraocular injections is an unmet clinical need. In the last decades, eye drops in which the drug molecules are encapsulated in nanocarriers have demonstrated notable enhancements in drug levels in both anterior and posterior eye segments [Citation8,Citation9]. Nanomicelles, liposomes, lipid nanoparticles, and polymer nanospheres provide protection against premature degradation, enhanced retention on eye surface, and novel pathways of penetration through corneal and transscleral routes [Citation10,Citation11]. Carriers that are spontaneously formed by self-assembly of their components in water are advantageous in terms of preparation and scale up because only few steps and energy are required. Polymeric micelles have outstanding capability to encapsulate hydrophobic drugs increasing apparent drug solubility, although concerns about premature disassembly may arise [Citation12]. Lipid-based vesicles, such as liposomes, may host both hydrophobic and hydrophilic active substances [Citation13], but the constituent lipids may be prone to chemical degradation [Citation14,Citation15]. In this context, niosomes as vesicles made of self-assembled nonionic surfactants may gather the advantages of both micelles and liposomes while providing improved physical and chemical stability. The development of niosomes as eye drops components is still incipient, but preliminary results have evidenced their potential [Citation16]. Other supramolecular structures such as self-assembling polypeptides have shown to be excellent drug carriers for other administration routes and may offer new avenues in the treatment of eye diseases not only as carriers but also as therapeutic agents [Citation17–19].
The aim of this review is to revisit first the role of diabetes in the development of eye diseases. Then, the pros and cons of different drug administration routes for diabetic eye treatments are considered. Commercially available drugs as well as those in clinical trials are analyzed in detail. Subsequent sections deal with the main self-assembled nano- and micro-carriers suitable for topical eye administration, namely, polymeric micelles, liposomes, and niosomes. Nanocarriers for treatment of diabetes-associated diseases using other administration routes have been tackled elsewhere [Citation20]. Finally, recent advances in the design of self-assembled carriers for topical diabetic eye drug administration are presented. Publications containing ex vivo or in vivo results or reporting on clinical trials have been prioritized.
2. Diabetic eye diseases
Diabetes may affect the anterior segment triggering the development of cataracts, dry eye syndrome, corneal ulcers, warts, tortuous conjunctival vessels, and keratopathy [Citation4,Citation21]. In the posterior segment, diabetes may contribute to glaucoma, retinopathy, and macular edema [Citation22]. Diabetic patients can also suffer from ocular neuropathy, difficulties in healing ocular wounds, and increased infection probabilities [Citation4].
Cataracts are described as the clouding of the lens. Three pathogenesis routes have been described [Citation23]: the polyol pathway where an accumulation of sorbitol induces hydropic lens fiber degeneration, the oxidative and osmotic stress leading to apoptosis of epithelial cells of the lens, and the autoimmunity. The polyol pathway is the one referred to most often and the most researched [Citation24]. Sugars (e.g. glucose and galactose) at elevated concentrations are favorable substrates for aldose reductase and generate intense osmotic stress (as explained in Section 3.1). Also, reducing sugars promote glycation (i.e. non-enzymatic glycosylation) of lens proteins, triggering cataract formation [Citation25].
Dry eye syndrome, which is characterized by an abnormal tear film, can be classified as either aqueous tear-deficient or evaporative (associated to a deficient tear film lipid layer) [Citation26]. Direct correlations have been found between prevalence of the syndrome and glycated hemoglobin and duration of diabetes. In vivo studies suggest that lachrymal glands undergo histological changes in diabetic patients and that oxidative stress derived from hyperglycemia might be involved in dry eye syndrome [Citation27].
Corneal ulcers are sores on the cornea that can alter the vision [Citation28]. The primary cause of corneal ulcers may be unrelated to diabetes (eye surgery, accidents), but the lengthier wound healing process makes ulcers a heavier burden for diabetic patients [Citation29,Citation30]. Therapeutic approaches to deal with diabetic keratopathy have been reviewed elsewhere [Citation31].
Glaucoma is the leading cause of blindness worldwide and is defined by damage of the retinal ganglion cells, leading to irreversible damage of the optic nerve [Citation32]. This is often accompanied by a rise of the intraocular pressure (IOP) triggered through different mechanisms. Open-angle glaucoma is caused by blocking of the trabecular meshwork, which in turn hinders fluid drainage and increases pressure. This is the most common form of glaucoma and happens at slow pace. Angle closure glaucoma is provoked by the iris coming forward and blocking the drainage angle between the iris and the cornea. It can happen over time or suddenly. Secondary angle closure glaucoma, in which the angle can be opened or closed, is caused by a secondary factor that leads to drainage hindrance, for example excessive pigment release blocking the trabecular meshwork (pigmentary glaucoma). Although the role of diabetes is unclear, direct correlations were found between diabetes duration and fasting glucose levels and the increase in IOP [Citation33].
Diabetic retinopathy involves damage to the retinal blood vessels, which has disastrous effects on the retina [Citation34]. In nonproliferative diabetic retinopathy, the retinal blood vessels are damaged and start leaking, which increases the pressure in the tissue. This condition can progress from microaneurysms to severe macular edema. In the proliferative diabetic retinopathy, the damaged blood vessels close and new blood vessels start to grow abnormally. The consequences may include increased IOP, accumulation of scar tissue, and ultimately nerve damage. Diabetic retinopathy affects one out of two persons with type 1 diabetes [Citation35]. The duration of diabetes is a risk factor, and the prevalence of diabetic retinopathy increases from 8% after 3 years of diabetes to 80% after 15 years [Citation36].
Diabetic macular edema may be a consequence of diabetic retinopathy, where leaking blood vessels increase the fluid volume in the macula, resulting in vision loss [Citation37]. Two types of diabetic macular edema can be clinically differentiated: focal macular edema, which is characterized by microaneurysms on the retinal capillaries, and diffuse macular edema, which shows leakage from the blood-retinal barrier due to generalized damage [Citation38].
3. Delivery of drugs to diabetic eye
3.1. Main drug classes and current administration routes
As described earlier, diabetes-related eye diseases are varied and evolve along time to become chronic. This means that their treatment should be designed according to the prolonged time the drug should be administered. In this regard, three main strategies are so far the most investigated and used ones: (i) topical administration, mostly for the treatment of anterior segment diseases, with the limitation of poor ocular bioavailability; (ii) intraocular administration, which is more efficient in terms of ocular bioavailability but entails relevant risks for the patient; and (iii) oral administration, which is the most patient friendly route, but the ocular bioavailability is quite low even when large doses are administered. Pros and cons of the different administration routes are explained in .
Table 1. Administration routes for delivery of drugs to the eye
Efficient ocular drug delivery is a difficult goal to reach in any case. Depending on the administration procedure and the dosage form, ocular barriers can be either physiological or anatomical, and either static or dynamic [Citation39]. Different barriers located in the anterior and the posterior segments protect the eye against foreign substances coming from outside or inside the body (e.g. the bloodstream). Also, the goal of drug delivery can be different depending on the target cells. If the drug must reach a tissue protected by many barriers, drug permeation through these barriers is critical; prodrugs, penetration enhancers, and encapsulation in nanocarriers may be helpful tools [Citation40,Citation41]. If the aim is to continuously supply the drug to an area with important dynamic turnover of fluids, sustained drug delivery systems may be required [Citation42].
Regarding topical administration, the eye is dynamically protected by the blinking reflex, the tear clearance rate, and the nasolacrimal drainage. Adhesion to the corneal surface and promotion of the penetration might overcome these barriers. Drugs can follow three routes: corneal, scleral, and conjunctival [Citation43–47]. Major static corneal barriers are the corneal epithelium, which limits the absorption of macromolecules and hydrophilic drugs through tight junctions, and the corneal stroma, which limits the penetration of lipophilic molecules due to its high aqueous content [Citation44]. Thus, mid-lipophilic drugs are the most suitable candidates for corneal penetration and subsequent diffusion through aqueous humor for intraocular distribution. The iris and the nonpigmented ciliary epithelium further block drugs from passing to the aqueous humor, making make up the blood–aqueous barrier [Citation47]. Furthermore, the aqueous humor flow from the ciliary body to the cornea counteracts the diffusion of hydrophilic molecules trying to enter further in the eye. On this blood–aqueous barrier and also on the corneal epithelium, there are efflux pumps that expel the drugs back to the front of the eye [Citation48].
The conjunctiva opposes to drug entry in the eye tissues mainly due to the presence of conjunctival blood capillaries and lymph vessels, which reroute a major fraction of the drug dose to the blood stream. Drug access through the conjunctiva to the posterior segment may occur via passive or active transport [Citation45]. Although there are tight junctions among conjunctival epithelium cells, polar solutes up to 20 kDa can enter through paracellular diffusion across 5-nm pores [Citation49]. Peptides and proteins may find the additional barrier of enzymatic degradation and require co-administration with a protease inhibitor [Citation50]. Lipophilic drugs can still penetrate better via the transcellular route; the surface area is larger than for paracellular pathway, although efflux pumps pose a relevant challenge. Intense active carrier-mediated transport occurs at conjunctiva for some ions and nutrients, which may be exploited for drug and prodrug absorption [Citation46]. Drugs encapsulated in nanocarriers take benefit of the additional pathway of endocytosis, which is feasible both in cornea and conjunctiva [Citation51–53].
Once conjunctiva is crossed, the drug can move through sclera into the uvea and the retinal pigmented epithelium, and then move forward to neural retina and vitreous humor. The sclera performs as a size exclusion barrier, with the permeability decreasing exponentially with molecular radius and lipophilicity [Citation54]. The sclera is negatively charged at physiological pH and therefore electrostatic interactions must be considered too. Bruch’s–choroid complex traps positively charged lipophilic drugs [Citation55], and since Bruch’s membrane becomes less elastic with age (due to calcification of elastin and cross-linking of collagen) drug diffusion is hindered in elderly patients.
Systemic administration of ocular drugs is compromised by the blood-retinal barrier. The inner limiting membrane of the retinal pigment epithelium prevents passage of high-molecular-weight molecules from blood to vitreous and vice versa [Citation56]. Müller cells and astrocytes form tight junctions to regulate the passage of molecules between the outer choroid and the inner retina. Although the information on ocular bioavailability after drug systemic administration in humans is limited, some reports evidenced that for small drugs, such as ciprofloxacin, similar drug levels can be obtained in aqueous humor after topical instillation of the free drug or oral administration. The levels in vitreous humor are commonly higher after oral administration, but at expenses of exposing the whole organism to high drug dose [Citation57]. Also, interestingly, the drug can be found in tear fluid after oral administration but not because of distribution through the eye, as reported for cyclosporine A [Citation58]. The blood-retinal barrier efficiently prevents cyclosporine A diffusion from blood to the anterior segment, except during concomitance of inflammatory processes [Citation59].
Pharmacological treatments intended to stop ocular damage caused by hyperglycemia or at least delay the process rely on (i) reducing IOP (), (ii) blocking the abnormal growth of blood vessels, or (iii) inhibiting negative chemical pathways. Drugs like prostaglandins [Citation60], rho kinase inhibitors [Citation61], nitric oxides [Citation62], or miotic/cholinergic agents [Citation63] drain ocular fluids. Alpha-adrenergic agonists, β-blockers, and carbonic anhydrase inhibitors lower the amount of fluid produced in the eye [Citation64]. Both strategies result in a lowering of the IOP and are usually addressed using eye drops (). Additionally, new drug candidates are intended to act on the heme oxygenase 1 (HO-1)/carbon monoxide (CO) physiological pathway that regulates the IOP. The HO-1 produces protection against ischemic insult by producing CO, which has anti-inflammatory properties. Incidentally, CO protects retinal ganglion cells from ischemic/reperfusion injury. Decreased CO levels have been related to increased IOP and, therefore, drugs that release CO may be useful in glaucoma treatment [Citation65].
Table 2. Some active substances of medicines used to reduce the IOP. Data from the European Medicines Agency, https://www.ema.europa.eu/en/medicines
The growth of abnormal ocular blood vessels can be handled with anti-vascular endothelial growth factor (anti-VEGF) drugs [Citation66]. Bevacizumab and ranibizumab, which are respectively full antibody and antibody fragment that bind VEGF-A, and aflibercept, a recombinant protein that traps VEGF-A and VEGF-B, are the cornerstones for the therapy of diabetes-related macular edema and retinopathy [Citation67]. They require intravitreal injection, which is not absent of complications [Citation68]. Intraocular injections should be used as infrequently as possible, according to pro re nata or treat-and-extend protocols [Citation69]. Biodegradable delivery systems that sustain intraocular release avoiding multiple treatment and maintaining drug stability are under investigation [Citation70,Citation71]. Since each available anti-VEGF agent interacts quite differently with VEGF, characterization of the molecular interactions can improve the design of novel biological drugs potentially useful in clinical practice [Citation72].
Hyperglycemia is also responsible for triggering the polyol pathway. Under normoglycemic conditions, the Embden–Meyerhof–Parnas catabolism route that transforms glucose into pyruvate, NADH, and ATP becomes saturated. Consequently, the polyol pathway, which commonly transforms 3% glucose, enters into action with the participation of two enzymes: (i) aldose reductase that transforms glucose into sorbitol with the consumption of NADPH and (ii) sorbitol dehydrogenase that slowly converts sorbitol into fructose while consuming NAD+. The polyol pathway, which is very active in retina and lens, metabolizes more than 30% glucose under diabetic conditions [Citation73]. Accumulation of sorbitol causes osmotic stress, triggers leukocyte accumulation, disrupts blood-retinal barrier, favors cells apoptosis, and starts a cascade of oxidative stress-mediated reactions [Citation74]. The excess of fructose acts as precursor of advanced glycation-end products (AGEs). In this context, aldose reductase inhibitors are gaining increased attention, and epalrestat is approved in some countries for oral administration. As an alternative, drugs that accelerate the metabolic rate of sorbitol dehydrogenase and, thus, decrease the levels of sorbitol are being tested [Citation73].
In the later stages of the disease, laser treatment (mainly for photocoagulation) or surgery (when blood vessel leakage becomes excessive or there is scar tissue) can be proposed [Citation75,Citation76]. Vitreoretinal surgery, for example, involves the removal of part of the vitreous and scar tissue in order to ameliorate the patient’s vision [Citation77].
3.2. Drugs, biologics, and gene therapy in clinical trials
Relevance of the morbidity caused by diabetes on eye structures is exemplified by the 868 clinical studies in phases 1 to 4 in February 2021 when searching for ‘diabetic eye’ in the ClinicalTrials.gov database. Refinement of the information to select recruiting, enrolling, active, terminated, or completed trails rendered an outcome of 657 studies, with an ample distribution worldwide (). Most clinical trials are focused on the efficacy and safety of new molecules or novel administration routes, drug combinations, or delivery systems such as implants, microparticle depot formulations, or biopolymer–antibody conjugates. Microneedle patches that can be applied onto cornea or sclera for direct drug delivery in the aqueous or vitreous humor, respectively, are gaining increasing interest, although still in the preclinical phase [Citation78–80].
Figure 1. Regional distribution of clinical trials related to diabetic eye. Data source: ClinicalTrials.gov. There were 657 outcomes for ‘diabetic eye’ on February 2021. Applied filters were Recruiting, Active not recruiting, Completed, Enrolling by invitation, and Terminated
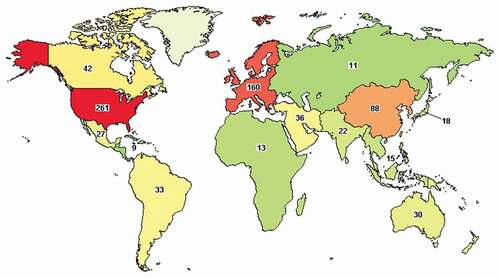
Most clinical trials related to diabetic eye refer to the conditions macular edema () and retinopathy (), and most interventions deal with drugs or biologics, particularly intravitreal injection of antibodies. However, the interest for oral administration as well as topical formulations does not decrease but is gaining attention, spearheaded by the search for novel active substances with improved ocular bioavailability and new therapeutic targets. Intense research on small molecules that perform as anti-inflammatory (e.g. nepafemac, loteprednol etabonate) or as anti-angiogenic/angiolytic (e.g. EXN407, OC-10X) is being carried out.
Table 3. Pharmacological treatments in clinical trials for diabetes-related macular edema classified as a function of the administration route, drug/biologic active substance, and number of clinical studies
Table 4. Pharmacological treatments in clinical trials for diabetes-related retinopathy classified as a function of the administration route, drug/biologic active substance, and number of clinical studies
For corneal epithelial defects, clinical trials deal with combinations of anti-inflammatory and antimicrobial drugs or autologous serum [Citation81]. Fonadelpar (SJP 0035), a peroxisome proliferator-activated receptor delta agonist, is in Phase III for dry eyes and Phase II for corneal disorders [Citation82]. Topical insulin and naltrexone eye drops have been shown to accelerate corneal epithelial healing and ameliorate dry eye symptoms in a variety of animal models [Citation83,Citation84]. Results of topical insulin and naltrexone clinical trials have not been posted yet [Citation85,Citation86].
Gene therapy of diabetic eye diseases is also an active field of research and clinical translation. The approval of Luxturna®, a virus-based gene delivery system for inherited retinal dystrophy, paved the road for other developments [Citation87–89]. There are currently 492 recruiting or active clinical trials on ocular gene therapy, most of which use adeno-associated viruses as carriers, according to ClinicalTrials.gov, clinicaltrialsregister.eu and rctportal.niph.go.jp. Differently to the repeated administration of drugs and biologics, gene therapy approaches pursue potential one-time treatment, namely the cells are instructed once to produce the needed therapeutic substance or to not produce the harmful substance. In the case of diabetes-related macular edema, three clinical trials with intravitreal formulations and one clinical trial with a suprachoroidal formulation for gene therapy are ongoing (). ADVM-022 (AAV.7m8-aflibercept) and RGX-314 (AAV8 vector containing a transgene for anti-VEGF fab) are intended to provide durable expression of an anti-VEFG antibody [Citation90,Citation91]. RGX-314 is also being tested for diabetic retinopathy [Citation92].
RNA interference therapy is in clinical trials too, although it may require repeated injections [Citation93]. For example, iCo-007 is a single-stranded antisense that degrades messenger RNA intended to target c-Raf kinase for diabetic macular edema treatment. Phase II results using intravitreal injections were not conclusive about safety and efficacy [Citation94]. PF-04523655 (RTP801I-14), a small-interfering RNA (siRNA) that may inhibit RTP801 gene transcription, is under evaluation as direct intravitreal injection. RTP801 is strongly upregulated in diabetic eyes and is associated with hypoxia and stress-related damage to retina cells [Citation95]. Gene therapy also offers excellent opportunities to address ocular inflammation triggered by sorbitol accumulation and AGEs [Citation74,Citation96,Citation97]. Gene therapy may allow for regulation of pro- and anti-inflammatory cytokines and neovascularization in keratitis, as reviewed elsewhere [Citation98].
4. Self-assembled nanocarriers for topical diabetic eye drugs
Most of drugs and new drug candidates in clinical trials for diabetic eye therapy, as referred in and , are quite hydrophobic and must be formulated as suspensions or ointments, showing limited ocular bioavailability after instillation. Thus, noninvasive topical therapeutic approaches may be notably improved if the drugs are formulated into delivery systems that could enhance their solubility and ocular permanence. In preclinical tests, micelles and cyclodextrin aggregates have been shown to enhance cornea and sclera accumulation and permeation of various hydrophobic diabetic eye drugs [Citation99–101]. Even in the search for novel delivery strategies, contact lenses have been designed specifically to deliver epalrestat [Citation102] and naltrexone [Citation103] and viral-based gene vectors [Citation104]. Regarding intravitreal gene therapy, siRNAs are extremely labile and rapidly cleared, and thus demand adequate nanocarriers. However, most non-viral vectors are strongly cationic polymers or lipids that may interact with negatively charged glycosaminoglycans in the vitreous humor. Such an interaction may prematurely break the poly/lipoplexes or alter the cell transfection and, thus, a very fine equilibrium in surface charge or a shell able to minimize retention in vitreous is required [Citation105,Citation106].
Self-assembled drug carriers that can be prepared in few steps and can encapsulate both small and large active substances, while providing a highly biocompatible, stealth interface are gaining increasing attention for ocular delivery. Although a plethora of novel self-assembled carriers are being tested, polymeric micelles, liposomes, and niosomes have already demonstrated in vivo promising performances [Citation13,Citation16,Citation99,Citation107]. Below, first the variables that drive the formation of these carriers and the main preparation protocols are revisited. Then, specific applications for management of diabetic eye diseases are analyzed.
4.1. The self-assembly process
Self-assembled carriers rely on amphiphilic components that bear regions of different affinity for water [Citation108]. The simplest self-assembled structure is that of common surfactant micelles. In contact with water, small surfactants move to the air–water interface with the polar head immersed in water while the apolar tail is prone to the air. Above a certain concentration, designed as critical micelle concentration (CMC), the air–water interface is saturated, and the surfactant molecules inside the aqueous medium associate to minimize the thermodynamically unfavorable exposure of the apolar tails to the aqueous environment. Thus, the apolar tails become the core (nucleus) of a supramolecular structure in which the polar heads remain exposed to the aqueous environment. The capability of surfactant micelles to encapsulate hydrophobic drugs is well known, but toxicity and physical instability limit their practical use as drug carriers [Citation109]. Micelles may extract relevant components from cells and, if they are made of ionic surfactants, may alter vital cell pathways, compromising the safety of the formulation [Citation110]. Moreover, the self-assembly is a reversible process, and assembled and non-assembled components are in a fragile equilibrium that can be displaced in any direction quite rapidly. Although the volume of liquid at the eye surface is less than in other administration routes, all topically instilled formulations are exposed to relevant tear turnover [Citation111]. Thus, one drop of common micelle formulation in contact with the lachrymal fluid undergoes a rapid decrease in surfactant concentration, and below the CMC, the micelle rapidly disassembles into their individual components [Citation112]. Consequently, few improvements (if any) compared to the instillation of the drug solely solution can be noticed, with the aggravating toxic effects that surfactant molecules may have on eye surface.
When searching for more biocompatible and stable self-assembled nanocarriers, two different strategies came up: core-shell polymeric micelles and bioinspired bilayered vesicles. This classification relies on the arrangement of the components, but as explained below, the same component can lead to micelles exhibiting a variety of shapes or to bilayered vesicles depending on its concentration and the presence of certain additives [Citation113]. For the sake of clarity, polymeric micelles are considered here as supramolecular assemblies of amphiphilic polymers (unimers) that have a core formed by apolar segments and a shell formed by polar segments [Citation112]. Thus, a gradient in polarity is observed from inside to outside [Citation113]. Differently, bilayered vesicles are defined as quasi-spherical structures in which the amphiphilic components are assembled in cell membrane-like bilayers (). Two or more bilayers can be arranged concentrically being separated from each other by an aqueous compartment. Therefore, bilayered vesicles do not exhibit progressive gradients in polarity, but stepped apolar-polar regions that can respectively encapsulate hydrophobic and hydrophilic compounds [Citation114,Citation115].
Figure 2. Dependence of the architecture of the self-assembled nanocarrier on the critical packing parameter (CPP)
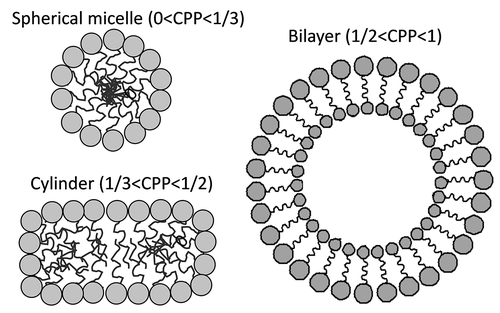
The amphiphilic component determines the physicochemical and biological properties of the self-assembled nanocarrier. The self-assembly process is regulated by hydrophobic interactions in aqueous environment, and thus the critical packing parameter (CPP) and the hydrophilic/lipophilic balance (HLB) of the amphiphilic component become decisive [Citation116,Citation117]. The hydrophilic heads maximize the contact surface with water, while the hydrophobic tails cluster together to minimize the contact with water. The formed structure arranges into the lower energy configuration, which is spherical or cylindrical depending on the CPP.
The volume of the head group, the volume of the hydrophobic tail (V), the equilibrium area per molecule at the interface surface (A), and the length of the hydrophobic tail (l) are the primary physical characteristics that determine the CPP, as follows [Citation116]:
The resulting value, which is unitless, determines the shape of the self-assembled structure. If the CPP is between 0 and 0.33, the self-assembled structure looks like a spherical micelle; between 0.33 and 0.5, the structure has rod-like shape; and only above 0.5 the structure becomes a bilayer, which can form vesicles () [Citation118].
The HBL, which is a measure of the balance of the size and strength of hydrophilic to hydrophobic regions, is calculated differently for different classes of amphiphilic substances. The HLB of polyoxyethylene alkyl ethers and polyoxyethylene esters is roughly estimated as the mass percentage of oxyethylene divided by five [Citation119]. The ideal HLB values for vesicle bilayer formation lies between 3 and 8, namely they fall in the range of ‘hydrophobic’ surfactants [Citation120]. Another relevant physical property is the gel–liquid transition temperature, which is the temperature at which the amphiphilic components go from closely packed in a gel state to a liquid state where they flow more freely. The aforementioned parameters come into play once the concentration of the amphiphilic component is appropriate for the structure desired, as it is possible to saturate the dispersions and create different aggregates based on the concentration of the surfactant. As an example, in the case of amphiphilic block copolymers, an increase in concentration may drive different unimers and micelles packaging leading to hydrogels and lyotropic liquid crystals [Citation121].
The physical stability of a self-assembled structure depends on thermodynamic and kinetic contributions [Citation112]. Self-assembly is a spontaneous phenomenon and, a priori, does not require solvent exchanges and purification, but it does not mean that occurs quickly and in many cases energy or multistep processes are required to obtain the desired structure. Moreover, an equilibrium between assembled and non-assembled components should be considered. The lower the CMC or the critical aggregation concentration (CAC), the less the ratio of free non-assembled components (unimers). The self-assembled structure is more thermodynamically stable when CMC or CAC are low and, therefore, less prone to disassembly once diluted. The strength of the interactions among the hydrophobic tails also determines the kinetics of the disassembly process. Closely packed components require more time for separation once the formulation is strongly diluted and, in turn, the integrity of the nanocarrier can be maintained for prolonged time [Citation12]. Indeed, physical stability under dilution is an index of the time that the unimers remain aggregate when the concentration is below the CMC and of the capability of the self-assembled carriers to retain the drug inside [Citation91,Citation122]. Strong changes in temperature as those that occur when steam heat sterilization is applied to prepare ophthalmic eye drops may trigger the aggregation or fusion of the self-assembled structure, or an increase in the permeability of the bilayer, which is quite common in the case of liposomes [Citation123].
4.2. Applications to diabetic eye
4.2.1. Polymeric micelles
Polymeric micelles can host a wide variety of low- and mid-polarity drugs and provide passive and active targeting [Citation99,Citation124]. Nevertheless, only a few papers have focused on the design of polymeric micelles for diabetes-related ocular diseases (). Soluplus® (polyvinyl caprolactam-polyvinyl acetate-polyethylene glycol copolymer) micelles (70–80 nm) have shown outstanding capability to solubilize alpha-lipoic acid and to withstand dilution in lachrymal fluid [Citation99]. Alpha-lipoic acid has beneficial effects in dry eye disease and diabetic retinopathy [Citation125], but its solubility and stability in aqueous medium are low. Drug-loaded Soluplus polymeric micelles could be sterilized through membrane filtration, freeze-dried, and reconstituted while maintaining their size and encapsulation efficiency. Corneal permeability (bovine) studies revealed that the micelles facilitate drug accumulation and pass across the tissue, providing alpha-lipoic acid levels well above those recorded for the commercially available eye drops [Citation125]. Moreover, the in situ gelling performance of Soluplus micelle formulations may provide prolonged retention time on the eye surface. Soluplus micelles also encapsulate progesterone, which has therapeutic potential against retinal degeneration, more efficiently than Pluronic micelles [Citation126]. The hydrophobic core of Soluplus facilitates the assembly at much lower CMC and the micelles are more stable. Studies carried out with cornea and sclera ex vivo from different animal sources (rabbit, pig, cow) revealed the strong influence of the interspecies anatomic differences on the drug permeability results, which may have an impact on the predictions of the performance on human eyes (
Figure 3. Progesterone (PG) apparent solubility in Soluplus and Pluronic F68 micelles, and permeability coefficients of cornea and sclera recorded for PG encapsulated in Soluplus 20% micelles or Pluronic F68 20% micelles. Reproduced from Alambiaga-Caravaca et al. [Citation126] (Creative Commons Attribution License)
![Figure 3. Progesterone (PG) apparent solubility in Soluplus and Pluronic F68 micelles, and permeability coefficients of cornea and sclera recorded for PG encapsulated in Soluplus 20% micelles or Pluronic F68 20% micelles. Reproduced from Alambiaga-Caravaca et al. [Citation126] (Creative Commons Attribution License)](/cms/asset/73d8f6e3-cc06-4169-81db-b9f6af591228/iedd_a_1953466_f0003_oc.jpg)
Table 5. Recent examples of self-assembled nanocarriers proposed for the topical ocular treatment of diabetic eye
Poly(ethylene glycol)-b-poly(lactic acid) micelles increased 10-fold triamcinolone acetonide apparent solubility using a copolymer concentration as low as 0.5 mg/mL. Drug-loaded micelles formulated in chitosan dispersion prolonged drug release for more than 1 week. In vivo (rabbit) evaluation in an inflammatory disease model revealed that twice a day instillation of the micelle solution with or without chitosan was able to recover the normal corneal epithelium [Citation127]. Inulin-based mucoadhesive micelles have been shown suitable for encapsulation of anti-inflammatory drugs adequate for macular edema treatment, such as dexamethasone, triamcinolone, and triamcinolone acetonide, enhancing drug permeability through corneal cells, which could be an alternative to intraocular injections [Citation128]. Also intended for macular degeneration, tacrolimus delivery may benefit from encapsulation in micelles made of PEG-hydrogenated castor oil-40 and octyxonyl-40, which have low CMC and provide slow drug release. Tacrolimus-loaded micelles showed faster cell internalization than the free drug [Citation129]. Mixed micelles of polyoxyl 40 stearate and polysorbate 80 successfully delivered dexamethasone (0.1%) to the back of the eye, providing therapeutic drug levels in retina and choroid after eye drop instillation [Citation130].
Prevention of abnormal growth of blood vessels in retina can be achieved by means of poly(ethylene glycol)-b-poly(propylene sulfide) micelles decorated with the anti-angiogenic peptide aANGP. The aANGP-micelles showed potent angiogenic inhibitory effect at 1000-fold lower concentration than the free peptide in cell cultures [Citation131].
Treatment of diabetic keratopathy may notably benefit from the encapsulation of genipin in dipotassium glycyrrhizinate micelles (29.5 nm) [Citation132]. Genipin and glycyrrhizin were shown to synergically block the high mobility group box 1 signaling and, in turn, attenuate the inflammation cascade overexpressed in diabetic eyes. These micelles showed good corneal permeability and favored corneal re-epithelialization and nerve regeneration in diabetic mice.
4.2.2. Liposomes
Liposomes are uni- or multi-bilayer vesicles composed of mainly amphiphilic lipids (phospholipids) and cholesterol. Each bilayer (also known as lamella) resembles the cell membrane, which endows liposomes with high biocompatibility. According to the number of bilayers and the overall size, liposome size may range from 10 to 100 nm (small unilamellar vesicles, SUV) to few microns (large unilamellar vesicles, LUV, and large multilamellar vesicles, LMV). General description of liposomes composition and preparation can be found elsewhere [Citation133]. Liposomes have been widely used as carriers since several decades ago because of their dual capability to simultaneously host hydrophilic and hydrophobic drugs plus the general advantages of passive and active targeting [Citation134,Citation135]. Usefulness of liposomes for topical ophthalmic drug delivery, and especially management of ocular surface diseases, has been recently reviewed [Citation13]. Importantly, the bioinspired structure and composition of liposomes facilitate the fusion with cell membrane at the cornea and conjunctiva surface and drug transfer to cell cytoplasm. Examples related to diabetic eye diseases are summarized in .
Regarding diabetic retinopathy, liposomes loaded with citicoline exhibited anti-inflammatory properties [Citation136]. Citicoline or cytidine 5´-diphosphocoline is an endogenous compound involved in the biosynthesis of phospholipids, showing neuroprotective activity. Citicoline oral solution is registered as Food for Special Medical purposed for patients suffering glaucoma, and an eye drop solution of a combination with vitamin B12 and sodium hyaluronate has been shown able to delay retinal changes in type 1 diabetic patients [Citation137]. The liposomal formulation has the advantage of avoiding the use of the benzalkonium chloride included in the eye drop solution. In a db/db mouse model, citicoline-loaded liposomes (instilled twice a day for 15 days) prevented the downregulation of synaptophysin in the retina and the upregulation of NF-κB and TNF-α induced by diabetes, which may be useful for the treatment in the early stages of the disease [Citation136].
Liposomes were also tested for the delivery of triamcinolone acetonide to the posterior segment to prevent macular edema [Citation138]. Preclinical studies revealed that 12 h after instillation (rabbits) of drug-loaded liposomes, a peak of triamcinolone acetonide concentration is reached in vitreous and retina, without triggering adverse events. The optimized formulation (QuSomes®) was formed spontaneously by adding Kolliphor HS15 and PEG-12 glyceryl dimyristate to an aqueous medium containing the drug (final concentration 2 mg/mL; i.e. 0.2%). In a clinical study with patients suffering from refractory pseudophakic cystoid macular edema, the triamcinolone acetonide-loaded liposomes (applied every 2 h for 90 days) improved the central foveal thickness and best-corrected visual acuity (BCVA) () [Citation139]. The formulation was well tolerated in both vitrectomized and nonvitrectomized eyes, being an alternative to the intraocular injections.
Figure 4. (a) Central foveal thickness (CFT), (b) best-corrected visual acuity (BCVA), and (c) intraocular pressure (IOP) recorded for patients with refractory pseudophakic cystoid macular edema during treatment with triamcinolone-loaded liposomes (one drop every 2 h for 90 days). Reproduced from Gonzalez-De la Rosa et al. [Citation139] (Creative Commons License)
![Figure 4. (a) Central foveal thickness (CFT), (b) best-corrected visual acuity (BCVA), and (c) intraocular pressure (IOP) recorded for patients with refractory pseudophakic cystoid macular edema during treatment with triamcinolone-loaded liposomes (one drop every 2 h for 90 days). Reproduced from Gonzalez-De la Rosa et al. [Citation139] (Creative Commons License)](/cms/asset/ec8296d3-a106-4ec6-8505-756fe23b3517/iedd_a_1953466_f0004_b.gif)
Coating of liposomes with chitosan may improve the encapsulation efficiency and accelerate the release of triamcinolone acetonide [Citation139]. After ocular instillation (rabbit) of coumarin-6 encapsulated in the chitosan-coated liposomes, OCT images of anterior and posterior segment evidenced an increase in fluorescence from the first 10 min up to 6 h and then the fluorescence slowly vanished. Differently, non-coated liposomes disappeared faster. As a control, free coumarin-6 solution did not significantly increase the fluorescence in the eye structures. These findings were attributed to the remarkably higher uptake of the chitosan-coated liposomes by corneal epithelial human colonic epithelial cells (HCECs) and retinal pigment epithelial (ARPE-19) [Citation140]. Similarly, polyamidoamine dendrimer (PAMAM G3.0)-coated liposomes have shown enhanced permeability in HCECs and in vivo (rabbit) transcorneal permeability of berberine hydrochloride [Citation141].
Interestingly, large molecules such as bevacizumab (avastin) may overcome the corneal barrier when encapsulated in liposomes that bind to the calcium-dependent phospholipid-binding protein annexin A5 [Citation142]. Annexin A5 can trigger the formation of endocytic vesicles and is quite abundant in the corneal epithelium [Citation143]. Liposomes (100 nm) formed by phospholipids, such as phosphatidylserine (PS) or phosphatidylethanolamine (PE), and annexin A5 (500:1 mol ratio) remarkably enhanced the transport through cornea compared to the same formulation without annexin A5. Instillation of one drop per day for 5 days (to rabbits) of bevacizumab-loaded liposomes resulted in levels 3-times higher in vitreous and 15-times higher in retina/choroid compared to a solution of free bevacizumab [Citation142]. Although these results are promising, the obtained levels of bevacizumab are still quite below those provided by intravitreal injection [Citation144]. Thus, intense research is devoted to improve the encapsulation yield of liposomes [Citation145]. Annexin A5-based unilaminar vesicles are also useful for the delivery of transforming growth factor-β1 (TGF-β1) for the treatment of macular degeneration. One hour after topical instillation (rabbit), therapeutical levels of TGF-β1 were quantified in the vitreous without signs of ocular irritation, which may help avoiding intraocular injections [Citation146].
Capability of liposomes to enhance corneal penetration has also been demonstrated for other therapeutic peptides. For example, vitamin E-based liposomes efficiently encapsulated a thrombospondin (TSP)-1-derived peptide for the handling of chronic ocular surface inflammation and tear film instability. Ex vivo studies revealed that the permeation through corneal tissue was remarkably improved for the encapsulated peptide [Citation147].
Improved gene delivery may be achieved with lipoplexes prepared with PEG-ceramide that enhances the transfection efficiency to the retinal pigment epithelium. PEG prevents lipoplexes aggregation in the vitreous, but hinders the transfection efficiency. Differently, PEG-ceramide detaches from the lipoplexes once in contact with the cell membranes, and the de-pegylated liposomes may escape from the endosome [Citation148]. Lipoplexes able to reach the posterior segment were produced using a microfluidizer and combining hydrogenated soy L-a-phosphatidylcholine, 1,2-distearoyl-sn-glycero-3-phosphoethanolamine-N-[amino(polyethylene glycol)-2000] and cholesterol. After one drop instillation (rat), small liposomes (<80 nm) decorated with transferrin permeated to the retinal pigment epithelium and were selectively retained in this tissue. Differently, larger liposomes lacking transferrin moved to the choroidal epithelium [Citation149].
4.2.3. Niosomes
Niosomes are gaining increasing interest due to the feasibility of combining the advantages of micelles (spontaneous self-assembly in water) and liposomes (encapsulation of both hydrophilic and lipophilic drugs) in unique polymeric multilayered vesicles while minimizing physical and chemical instability concerns.
Niosomes are defined as a uni- or multi-bilayer vesicles composed of mainly nonionic surfactants. This name was given by researchers at the L’Oréal laboratories in 1979 [Citation150], but niosomes have attracted interest as drug carriers only recently [Citation120]. Scientific research has been steadily increasing over the years from 4 articles about ‘niosomes AND drug’ in 2002 to 49 articles in 2020, and 1 article about ‘niosomes AND gene’ in 2005 to 14 articles in 2020 according to Web of Science database. The use of nonionic surfactants as primary building blocks grants niosomes high stability and long shelf life. Like liposomes, niosomes size may range from 10 to 100 nm (SUV) to few microns (LUV and LMV) [Citation120]. Tuning the affinity of the components for the drug as well as the permeability of the layer may allow for precise regulation of drug release [Citation151,Citation152]. These attributes make niosomes very attractive as drug nanocarriers.
To improve the performance of niosomes for specific applications multiple other molecules can be added. Examples are cholesterol and squalene, which increase the stiffness of the vesicle to enhance the stability [Citation153], dicetyl phosphate, or stearylamine that add charged groups to the vesicle to improve its drug delivery capabilities, or PEG that provides a stealth layer to increase blood residence time. Also, niosomes responsive to changes in pH or even to glucose have been designed [Citation154,Citation155].
Most techniques to prepare niosomes are derived from liposome formation and adapted to the needs of nonionic surfactants (). The drugs are dissolved either in an organic solvent or in the aqueous phase depending on their affinity for water. The preparation method may have a major impact on the size, structure and, therefore, properties of the niosomes [Citation120,Citation152,Citation156]. The methods of thin-film hydration, reverse-phase evaporation, and emulsification/sonication are quite similar as they use mechanical disruption to form the niosomes in a hydration step. Organic phase injection and microfluidics are positioning as suitable techniques for precise control of niosomes size and polydispersity and preliminary scale-up [Citation157]. On the other hand, proniosome appeared as a tool to extend shelf-life avoiding any premature leakage of the drug. Proniosomes can be presented as either anhydrous free-flowing powders or as gel-like mixtures of the niosome components in a liquid medium without water or with a minor content in water. Thus, proniosomes are intended for rapid reconstitution with water before administration or once in contact with the physiological fluids [Citation158].
Niosomes have been shown able to improve oral bioavailability of a variety of treatments for diabetes, including insulin [Citation159], metformin hydrochloride solely or combined with glipizide [Citation160,Citation161], and plant extracts [Citation162]. Niosomes also attract great attention as ocular drug carriers due to their excellent tolerability, prolonged precorneal residence, and enhanced ocular bioavailability [Citation16,Citation151]. Nevertheless, examples of their performance as delivery systems of diabetic eye drugs are still incipient (). Multilayered niosomes (7–14 μm) have been designed to encapsulate naltrexone hydrochloride, which is a potent opioid antagonist that markedly accelerates cornea healing and repairs the signs of diabetic keratopathy. The niosomes prepared with Span 60 and cholesterol using the thin-film hydration method showed high encapsulation efficiency [Citation163]. These niosomes had a gel–liquid transition of few degrees above physiological temperature, which is considered as an advantage to avoid dragging by blinking and to control drug release [Citation164]. Ex vivo tests in bovine cornea evidenced that niosomes sustainedly released the drug for several hours and allowed for high permeability [Citation165].
Span 60-based niosomes (2.8 μm) dispersed in 1% Carbopol 934 gel were shown suitable for delivery of the anti-inflammatory drug flurbiprofen in the aqueous humor [Citation166]. Once applied onto the cornea (rabbits), flurbiprofen-loaded niosomes either solely or in the Carbopol gel provided drug levels in aqueous humor one order of magnitude above those achieved using a free drug solution. Relative drug bioavailability from the niosome and the niosome-gel formulations was 3.6- and 6.2-fold that of the flurbiprofen solution because the gel notably extended the permanence time on the eye surface. The niosome formulations also demonstrated to be therapeutically efficient against keratitis (carrageenan-induced inflammation). The obtained results suggest that once a day instillation may be sufficient to manage ocular inflammatory diseases.
Feasibility of using niosomes for glaucoma therapy has been evidenced recently. Niosomes prepared by the reverse-phase evaporation technique covering wide ratios of cholesterol and Span 40 or Span 60 could host latanoprost with an encapsulation efficiency of ~98%. Latanoprost-loaded niosomes (8–10 μm size) were dispersed in Pluronic F127 gel (drug concentration 0.005%) and the effects on IOP were monitored after instillation (50 μL) in the cul-de-sac of rabbit eyes. Latanoprost-loaded niosomes reduced IOP for 3 days, which was remarkably longer than the outcome achieved with the standard latanoprost eye drops (Xalatan®; 0.005%) () [Citation167]. Proniosomal gel formulations are promising also for other anti-glaucoma agents, including dorzolamide hydrochloride and brimonidine tartrate [Citation168,Citation169].
Figure 6. Reduction in the intraocular pressure (IOP) observed after topical administration to normotensive rabbits of latanoprost-loaded niosome gel or conventional latanoprost eye drops. Error bars represent standard deviation of six replicates. Reproduced from Fathalla et al. [Citation167] with permission from Taylor & Francis (http://www.tandfonline.com)
![Figure 6. Reduction in the intraocular pressure (IOP) observed after topical administration to normotensive rabbits of latanoprost-loaded niosome gel or conventional latanoprost eye drops. Error bars represent standard deviation of six replicates. Reproduced from Fathalla et al. [Citation167] with permission from Taylor & Francis (http://www.tandfonline.com)](/cms/asset/5bbf12a9-4420-473a-8856-3e3afb29664f/iedd_a_1953466_f0006_oc.jpg)
Interestingly, in the last few years, most publications on niosomes refer to the feasibility of forming nioplexes for a variety of therapeutic applications, including gene therapy to the back of the eye [Citation170]. Nevertheless, most reports rely on subretinal or intravitreal injections [Citation171,Citation172]. To the best of our knowledge, the suitability of nioplexes for topical ocular administration is still to be explored.
5. Conclusions
With the upward trend in obesity worldwide, the number of cases of diabetic eye diseases is also bound to increase, making research on the delivery of ocular drugs an active field. This is reflected in the large number of ongoing clinical trials for diabetic eye diseases. The trials are primarily focused on macular edema and retinopathy, with a plethora of active substances to be administered through multiple routes. Although the eye presents challenging anatomical barriers, different self-assembled carriers have been proven to be capable of overcoming them and offer a promising future for drug delivery to the ocular therapeutic sites. Micelles, liposomes, and niosomes offer diverse advantages covering from the increase in drug solubility to the use of additional pathways of penetration into tissues. Preclinical and clinical studies have confirmed their ability to protect the cargo from degradation, target specific areas, enhance permeation through different ocular barriers, and sustain the release, which in turn improves the therapeutic effects both in intensity and in duration. Niosomes made of nonionic surfactants are particularly interesting, and scientific research has grown steadily in the last few years, as an alternative to liposomes in terms of higher stability and lower production costs. Advances in right choosing of the composition and size of the self-assembled carriers may result in more successful management of both anterior and posterior segment eye diseases.
6. Expert Opinion
The diabetic eye is one of the clearest examples of the need to develop adequate drug delivery systems that can improve or reverse the effects of a very relevant chronic disease. Given the pandemic character of diabetes, developing formulations that alleviate or reverse the deleterious effects at ocular level and the subsequent consequences on the quality of life of patients is an unsatisfied clinical demand. Most drugs currently approved or under evaluation in clinical trials require nonviable frequent instillation or intraocular administration using invasive procedures. Since diabetic-eye diseases need chronic treatments, an adequate balance between patient acceptability and drug ocular bioavailability should be reached.
Most clinical trials related to diabetic eye refer to macular edema and retinopathy, although affectation of anterior segment is also considered. Strong research efforts are being made at two levels: (i) to find new drug candidates and to test the therapeutic efficiency of drugs already approved for systemic administration when they are directly administered to the ocular tissues and (ii) to find suitable delivery vehicles that can overcome eye barriers and help the drug to get access to the damaged tissue and to remain in it for prolonged time. At the pharmacological level, a myriad of drugs and biologics are being tested, mostly searching for anti-inflammatory and anti-angiogenic activities. Although long-sought, the recent approval of gene therapy treatments for inherited eye diseases is undoubtedly driving the development of appropriate approaches for acquired diseases. The feasibility of transforming the patient’s eye cells into factories of the necessary drug is closer than ever. Gene therapy relies on a single administration or administrations widely separated in time. One-time treatment may be compatible with invasive maneuvers as the benefit may counteract the risks. Nevertheless, drugs, biologics, and genes may strongly benefit from more patient-friendly approaches.
From a pharmaceutical technology level, most therapeutic agents for diabetic eye have poor biopharmaceutic properties because they are poorly soluble or the size is quite large. Thus, self-assembled carriers (polymeric micelles, liposomes, and niosomes) that can facilitate the penetration of small and large molecules deep in the ocular tissues may become valuable tools. Topical management of eye surface and anterior segment diseases has already been shown to improve with self-assembled carriers that show prolonged permanence on ocular surface, facilitate drug permeation through cornea, and diffusion into the aqueous humor. Moreover, the feasibility of the carriers to encapsulate large molecules (including antibodies and genes) pass through sclera while minimizing systemic clearance, and modulate diffusion through vitreous may make topical administration a realistic alternative to intraocular injection. Remarkably, the gain in knowledge about transporters present in each ocular tissue and about the biomarkers that can be found altered due to diabetes may provide interesting clues for the design of drug carriers with greater penetration capacity and targeting ability. This field is still in its infancy, but the intense quest to provide the patients with efficient yet friendly formulations for long-term use will pave the way to clinic of novel topical formulations.
Article highlights
Diabetes mellitus currently affects 8.5% people worldwide and causes the highest lost in years of quality of life due to disability.
The term ‘diabetic eye disease’ has a broad meaning as it may encompass multiple illnesses in different eye tissues.
Pharmacological treatments for diabetic eye rely on reducing the intraocular pressure, blocking the abnormal growth of blood vessels, or inhibiting negative chemical pathways.
More than half a thousand clinical studies in phases 1–4 are currently in progress with a variety of drugs, biologics and gene therapy for diabetic eye diseases.
Chronic ocular treatments demand drug delivery systems that can become an alternative to intraocular injections combining patient-friendly administration and high ocular bioavailability.
Drug carriers for topical instillation prevent from premature degradation, enhance the retention on eye surface, and use alternative pathways for cornea and sclera penetration.
Polymeric micelles with hydrophobic core and hydrophilic shell show a polarity gradient from inside to outside facilitating the encapsulation of apolar drugs.
Bilayered vesicles formed by amphiphilic components assembled as concentric cell membrane-like bilayers show stepped apolar-polar regions, which allow for encapsulation of both hydrophilic and hydrophobic compounds.
Niosomes as vesicles made of self-assembled nonionic surfactants gather the advantages of micelles and liposomes while providing improved physical and chemical stability.
The development of drug-loaded niosomes as eye drops is still incipient, but preliminary results have evidenced their potential.
Despite the success of subretinal and intravitreal administration of nioplexes, their suitability for topical ocular administration is still to be explored.
This box summarizes key points contained in the article.
Declaration of interest
The authors have no relevant affiliations or financial involvement with any organization or entity with a financial interest in or financial conflict with the subject matter or materials discussed in the manuscript. This includes employment, consultancies, honoraria, stock ownership or options, expert testimony, grants or patents received or pending, or royalties.
Reviewer disclosures
Peer reviewers on this manuscript have no relevant financial or other relationships to disclose.
Additional information
Funding
References
- Lin X, Xu Y, Pan X, et al. Global, regional, and national burden and trend of diabetes in 195 countries and territories: an analysis from 1990 to 2025. Sci Rep. 2020;10:14790.
- Greiner W, Patel K, Crossman-Barnes CJ, et al. High-expenditure disease in the EU‑28: does drug spend correspond to clinical and economic burden in oncology, autoimmune disease and diabetes? Pharmacoecon Open. 2021. DOI:https://doi.org/10.1007/s41669-020-00253-4
- National Institute of Diabetes and Digestive and Kidney Diseases. Diabetic Eye Disease. [cited Feb 2021]. https://www.niddk.nih.gov/health-information/diabetes/overview/preventing-problems/diabetic-eye-disease
- Zhu L, Titone R, Robertson DM. The impact of hyperglycemia on the corneal epithelium: molecular mechanisms and insight. Ocul Surf. 2019;17:644–654.
- Sayin N. Ocular complications of diabetes mellitus. World J Diabetes. 2015;6:92.
- Han SB, Yang HK, Hyon JY. Influence of diabetes mellitus on anterior segment of the eye. Clin Interv Aging. 2019;14:53–63.
- Giri B, Dey S, Das T, et al. Chronic hyperglycemia mediated physiological alteration and metabolic distortion leads to organ dysfunction, infection, cancer progression and other pathophysiological consequences: an update on glucose toxicity. Biomed Pharmacother. 2018;107:306–328.
- Madni A, Rahem MA, Tahir N, et al. Non-invasive strategies for targeting the posterior segment of eye. Int J Pharm. 2017;530:326–345.
- Jumelle C, Gholizadeh S, Annabi N, et al. Advances and limitations of drug delivery systems formulated as eye drops. J Control Release. 2020;321:1–22.
- Liu S, Jones L, Gu FX. Nanomaterials for ocular drug delivery. Macromol Biosci. 2012;12:608–620.
- Mazureki Campos P, Petrilli R, Lopez RFV. The prominence of the dosage form design to treat ocular diseases. Int J Pharm. 2020;586:119577.
- Polat H, Kutluay G, Polat M. Analysis of dilution induced disintegration of micellar drug carriers in the presence of inter and intra micellar species. Colloid Surf A-Physicochem Eng Asp. 2020;601:124989.
- López-Cano JJ, González-Cela-Casamayor MA, Andrés-Guerrero V, et al. Liposomes as vehicles for topical ophthalmic drug delivery and ocular surface protection. Expert Opin Drug Deliv. 2021;18(7):819–847.
- FDA. Liposome Drug Products. Guidance for Industry. April 2018. [cited Feb 2021]. https://www.fda.gov/media/70837/download
- Delma KL, Lechanteur A, Evrard B, et al. Sterilization methods of liposomes: drawbacks of conventional methods and perspectives. Int J Pharm. 2021;597:120271.
- Durak S, Rad ME, Yetisgin AA, et al. Niosomal drug delivery systems for ocular disease—recent advances and future prospects. Nanomaterials. 2020;10:1191.
- MacEwan SR, Chilkoti A. Applications of elastin-like polypeptides in drug delivery. J Control Release. 2014;190:314–330.
- Castillo-Díaz LA, Ruiz-Pacheco JA, Elsawy MA, et al. Self-assembling peptides as an emerging platform for the treatment of metabolic syndrome. Int J Nanomed. 2020;15:10349–10370.
- Sreekumar PG, Kannan R. Mechanisms of protection of retinal pigment epithelial cells from oxidant injury by humanin and other mitochondrial-derived peptides: implications for age-related macular degeneration. Redox Biol. 2020;37:101663.
- Kesharwani P, Gorain B, Low SY, et al. Nanotechnology based approaches for anti-diabetic drugs delivery. Diabetes Res Clin Pract. 2018;136:52–77.
- Adeoti CO, Isawumi MA, Ashaye AO, et al. The anterior segment of the eye in diabetes. Clin Ophthalmol. 2012;6:667–671.
- Lutty GA. Effects of diabetes on the eye. Investig Ophthalmol Vis Sci. 2013;54:81–87.
- Kiziltoprak H, Tekin K, Inanc M, et al. Cataract in diabetes mellitus. World J Diabetes. 2019;10:140–153.
- Pollreisz A, Schmidt-Erfurth U. Diabetic cataract—pathogenesis, epidemiology and treatment. J Ophthalmol. 2010;2010:1–8.
- Abdelkader H, Alany RG, Pierscionek B. Age-related cataract and drug therapy: opportunities and challenges for topical antioxidant delivery to the lens. J Pharm Pharmacol. 2015;67:537–550.
- Zhang X, Zhao L, Deng S, et al. Dry eye syndrome in patients with diabetes mellitus: prevalence, etiology, and clinical characteristics. J Ophthalmol. 2016;2016:8201053.
- Módulo CM, Jorge AG, Dias AC, et al. Influence of insulin treatment on the lacrimal gland and ocular surface of diabetic rats. Endocrine. 2009;36:161–168.
- Hyndiuk RA, Kazarian EL, Schultz RO, et al. Neurotrophic corneal ulcers in diabetes mellitus. Arch Ophthalmol. 1977;95:2193–2196.
- Ioannidis AS, Zagora SL, Wechsler AW. A non-healing corneal ulcer as the presenting feature of type 1 diabetes mellitus: a case report. J Med Case Rep. 2011;5:539.
- Xu KP, Li Y, Ljubimov AV, et al. High glucose suppresses epidermal growth factor receptor/ phosphatidylinositol 3-kinase/akt signaling pathway and attenuates corneal epithelial wound healing. Diabetes. 2009;58:1077–1085.
- Abdelkader H, Patel DV, McGhee CN, et al. New therapeutic approaches in the treatment of diabetic keratopathy: a review. Clin Exp Ophthalmol. 2011;39:259–270.
- Weinreb RN, Aung T, Medeiros FA. The pathophysiology and treatment of glaucoma. JAMA. 2014;311:1901.
- Zhao D, Cho J, Kim MH, et al. Diabetes, fasting glucose, and the risk of glaucoma: a meta-analysis. Ophthalmology. 2015;122:72–78.
- Rübsam A, Parikh S, Fort PE. Role of inflammation in diabetic retinopathy. Int J Mol Sci. 2018;19:1–31.
- Mathur R, Douglas I, Bhaskaran K, et al. Diabetic eye disease: a UK Incidence and Prevalence Study; 2017. [cited Feb 2021]. https://www.rnib.org.uk/sites/default/files/Diabetic%20eye%20disease.%20A%20UK%20Incidence%20and%20Prevalence%20Study%20-%20Full%20report.pdf
- Fong DS, Aiello L, Gardner TW, et al. Retinopathy in diabetes. Diabetes Care. 2004;27:S84–87.
- Bandello F, Pognuz R, Polito A, et al. Diabetic macular edema: classification, medical and laser therapy. Semin Ophthalmol. 2003;18:251–258.
- Browning DJ, Altaweel MM, Bressler NM, et al. Diabetic retinopathy clinical research network. Diabetic macular edema: what is focal and what is diffuse? Am J Ophthalmol. 2008;146:649–655.
- Agrahari VV, Mandal A, Agrahari VV, et al. A comprehensive insight on ocular pharmacokinetics. Drug Deliv Transl Res. 2016;6:735–754.
- Gote V, Ansong M, Pal D. Prodrugs and nanomicelles to overcome ocular barriers for drug penetration. Expert Opin Drug Metab Toxicol. 2020;16:885–906.
- Thareja A, Hughes H, Alvarez-Lorenzo C, et al. Penetration enhancers for topical drug delivery to the ocular posterior segment – a systematic review. Pharmaceutics. 2021;13:276.
- Subrizi A, del Amo EM, Korzhikov-Vlakh V, et al. Design principles of ocular drug delivery systems: importance of drug payload, release rate, and material properties. Drug Discov Today. 2019;24:1446–1457.
- Mari K, Kirsi H, Seppo K, et al. Characterization of paracellular and aqueous penetration routes in cornea, conjunctiva, and sclera. Invest Ophthalmol Vis Sci. 1997;38:627–634.
- Gaudana R, Ananthula HK, Parenky A, et al. Ocular drug delivery. AAPS J. 2010;12:348–360.
- Lee SJ, He W, Robinson SB, et al. Evaluation of clearance mechanisms with transscleral drug delivery. Invest Ophthalmol Vis Sci. 2010;51:5205–5212.
- Hosoya K, Lee VHL, Kim KJ. Roles of the conjunctiva in ocular drug delivery: a review of conjunctival transport mechanisms and their regulation. Eur J Pharm Biopharm. 2005;60:227–240.
- Delamare NA. Ciliary body and ciliary epithelium. Adv Organ Biol. 2005;10:127–148.
- Dahlin A, Geier E, Stocker SL, et al. Gene expression profiling of transporters in the solute carrier and ATP-binding cassette superfamilies in human eye substructures. Mol Pharm. 2013;10:650–663.
- Horibe Y, Hosoya K, Kim KJ, et al. Polar solute transport across the pigmented rabbit conjunctiva: size dependent and the influence of 8-bromo cyclic adenosine monophosphate. Pharm Res. 1997;14:1246–1251.
- Sun L, Basu SK, Kim KJ, et al. Arginine vasopressin transport and metabolism in the pigmented rabbit conjunctiva. Eur J Pharm Sci. 1998;6:47–52.
- Liu D, Lian Y, Fang Q, et al. Hyaluronic-acid-modified lipid-polymer hybrid nanoparticles as an efficient ocular delivery platform for moxifloxacin hydrochloride. Int J Biol Macromol. 2018;116:1026–1036.
- Qaddoumi MG, Gukasyan HJ, Davda J, et al. Clathrin and caveolin-1 expression in primary pigmented rabbit conjunctival epithelial cells: role in PLGA nanoparticle endocytosis. Mol Vis. 2003;9:559–568.
- Müller-Lierheim WGK. Why chain length of hyaluronan in eye drops matters. Diagnostics. 2020;10:511.
- Ambati J, Adamis AP. Transscleral drug delivery to the retina and choroid. Prog Retin Eye Res. 2002;21:145–151.
- Cheruvu NPS, Kompella UB. Bovine and porcine transscleral solute transport: influence of lipophilicity and the choroid-Bruch’s layer. Invest Ophthalmol Vis Sci. 2006;47:4513–4522.
- Ramsay E, Hagström M, Vellonen KS, et al. Role of retinal pigment epithelium permeability in drug transfer between posterior eye segment and systemic blood circulation. Eur J Pharm Biopharm. 2019;143:18–23.
- Çekiç O, Batman C, Yasar U, et al. Human aqueous and vitreous humour levels of ciprofloxacin following oral and topical administration. Eye. 1999;13:555–558.
- Mora P, Ceglarek U, Manzotti F, et al. Cyclosporin A in the ocular fluids of uveitis patients following long-term systemic administration Graefe’s Arch. Clin Exp Ophthalmol. 2008;246:1047–1052.
- BenEzra D, Maftzir G, De Courten C, et al. Ocular penetration of cyclosporine A. III: the human eye. Br J Ophthalmol. 1990;74:350–352.
- Toris CB, Gabelt BT, Kaufman PL. Update on the mechanism of action of topical prostaglandins for intraocular pressure reduction. Surv Ophthalmol. 2008;53(Supplement 1):107–120.
- Moshirfar M, Parker L, Birdsong OC, et al. Use of rho kinase inhibitors in ophthalmology: a review of the literature. Med Hypothesis Discov Innov Ophthalmol J. 2018;7:101–111.
- Muenster S, Lieb WS, Fabry G, et al. The ability of nitric oxide to lower intraocular pressure is dependent on guanylyl cyclase. Invest Ophthalmol Vis Sci. 2017;58:4826–4835.
- Faiq MA, Wollstein G, Schuman JS, et al. Cholinergic nervous system and glaucoma: from basic science to clinical applications. Prog Retin Eye Res. 2019;72:100767.
- Schmidl D, Schmetterer L, Garhöfer G, et al. Pharmacotherapy of glaucoma. J Ocul Pharmacol Ther. 2015;31:63–77.
- Bucolo C, Drago F. Carbon monoxide and the eye: implications for glaucoma therapy. Pharmacol Ther. 2011;130:191–201.
- Akiyode O, Tran C. Overview of ocular anti-vascular endothelial growth factor therapy in the management of diabetic eye complications. Diabetes Spectr. 2016;29:44–49.
- Gale MJ, Scruggs BA, Flaxel CJ. Diabetic eye disease: a review of screening and management recommendations. Clin Exp Ophthalmol. 2021;49:128–145.
- Smith SJ, Smith BD, Mohney BG. Ocular side effects following intravitreal injection therapy for retinoblastoma: a systematic review. Br J Ophthalmol. 2014;98:292–297.
- Augsburger M, Sarra GM, Imesch P. Treat and extend versus pro re nata regimens of ranibizumab and aflibercept in neovascular age-related macular degeneration: a comparative study Graefe’s. Arch Clin Exp Ophthalmol. 2019;257:1889–1895.
- Radhakrishnan K, Sonali N, Moreno M, et al. Protein delivery to the back of the eye: barriers, carriers and stability of anti-VEGF proteins. Drug Discov Today. 2017;22:416–423.
- Conti B, Bucolo C, Giannavola C, et al. Biodegradable microspheres for the intravitreal administration of acyclovir: in vitro/in vivo evaluation. Eur J Pharm Sci. 1997;5:287–293.
- Platania CB, Di Paola L, Leggio GM, et al. Molecular features of interaction between VEGFA and anti-angiogenic drugs used in retinal diseases: a computational approach. Front Pharmacol. 2015;6:248.
- Patil KK, Gacche RN. The fate of aldose reductase inhibition and sorbitol dehydrogenase activation. Austin J Endocrinol Diabetes. 2019;6:1064.
- Obrosova IG, Kador PF. Aldose reductase/polyol inhibitors for diabetic retinopathy. Curr Pharm Biotechnol. 2011;12:373–385.
- Evans JR, Michelessi M, Virgili G. Laser photocoagulation for proliferative diabetic retinopathy. Cochrane Database Syst Rev. 2014;2014:CD011234.
- Crosson JN, Mason L, Mason JO. The role of focal laser in the anti-vascular endothelial growth factor era. Ophthalmol Eye Dis. 2017;9:1179172117738240.
- Papastefanou VP, Dooley I, Zambarakji H. Management of macular edema in vitrectomized patients with diabetes. Expert Rev Ophthalmol. 2018;13:87–103.
- Thakur RRS, Tekko IA, Al-Shammari F, et al. Rapidly dissolving polymeric microneedles for minimally invasive intraocular drug delivery. Drug Deliv Transl Res. 2016;6:800–815.
- Roy G, Galigama RD, Thorat VS, et al. Microneedle ocular patch: fabrication, characterization, and ex-vivo evaluation using pilocarpine as model drug. Drug Dev Ind Pharm. 2020;46:1114–1122.
- Amer M, Chen RK. Self-adhesive microneedles with interlocking features for sustained ocular drug delivery. Macromol Biosci. 2020;20:2000089.
- Chen YM, Hu FR, Huang JY, et al. The effect of topical autologous serum on graft re-epithelialization after penetrating keratoplasty. Am J Ophthalmol. 2010;150:352–359.e2.
- A study to assess the safety and effectiveness of sjp-0035 for the treatment of patients with dry eye disease. https://clinicaltrials.gov/ct2/show/NCT03527212
- Klocek MS, Sassani JW, McLaughlin PJ, et al. Naltrexone and insulin are independently effective but not additive in accelerating corneal epithelial healing in type I diabetic rats. Exp Eye Res. 2009;89:686–692.
- Ionascu I, Argaseala A, Uzun S, et al. A new eye drop formulation used in the management of corneal ulcers in dogs and cats. Agrolife Sci J. 2020;9:164–171.
- Topical Autologous Insulin Application for the Treatment of Corneal Epithelium Defect After Ocular Surgeries. https://clinicaltrials.gov/ct2/show/study/NCT01031888?term=topical+insulin&draw=2&rank=2
- Effect of Topical Naltrexone Ophthalmic Solution on the Signs and Symptoms of Dry Eye in Diabetic Subjects. https://ClinicalTrials.gov/show/NCT03660475
- Keeler AM, Flotte TR. Recombinant adeno-associated virus gene therapy in light of Luxturna (and Zolgensma and Glybera): where are we, and how did we get here? Annu. Rev Virol. 2019;6:601–621.
- Auricchio A, Smith AJ, Ali RR. The future looks brighter after 25 years of retinal gene therapy. Hum Gene Ther. 2017;28:982–987.
- Bastola P, Song LJ, Gilger BC, et al. Adeno-associated virus mediated gene therapy for corneal diseases. Pharmaceutics. 2020;12:767.
- ADVM-022 Intravitreal Gene Therapy for DME (INFINITY). https://ClinicalTrials.gov/show/NCT04418427
- Liu Y, Fortmann SD, Shen J, et al. AAV8-antiVEGFfab ocular gene transfer for neovascular age-related macular degeneration. Mol Ther. 2018;26:542–549.
- RGX-314 Gene Therapy Administered in the Suprachoroidal Space for Participants With Diabetic Retinopathy (DR) Without Center Involved-Diabetic Macular Edema (CI-DME) (ALTITUDE). https://clinicaltrials.gov/ct2/show/record/NCT04567550
- Jiang J, Zhang X, Tang Y, et al. Progress on ocular siRNA gene-silencing therapy and drug delivery systems. Fundam Clin Pharmacol. 2021;35:4–24.
- A randomized, multi-center, phase ii study of the safety, tolerability and bioactivity of repeated intravitreal injections of iCo-007 as monotherapy or in combination with ranibizumab or laser photocoagulation in the treatment of diabetic macular edema (the iDEAL Study) (iDEAL). https://ClinicalTrials.gov/show/NCT01565148
- Rittenhouse KD, Johnson TR, Vicini P, et al. RTP801 gene expression is differentially upregulated in retinopathy and is silenced by PF-04523655, a 19-Mer siRNA directed against RTP801. Invest Ophthalmol Vis Sci. 2014;55:1232–1240.
- Kallinikou D, Soldatou A, Tsentidis C, et al. Diabetic neuropathy in children and adolescents with type 1 diabetes mellitus: diagnosis, pathogenesis, and associated genetic markers. Diabetes Metab Res Rev. 2019;35:e3178.
- Papachristoforou E, Lambadiari V, Maratou E, et al. Association of glycemic indices (hyperglycemia, glucose variability, and hypoglycemia) with oxidative stress and diabetic complications. J Diabetes Res. 2020;2020:7489795.
- Torrecilla J, del Pozo-Rodríguez A, Vicente-Pascual M, et al. Targeting corneal inflammation by gene therapy: emerging strategies for keratitis. Exp Eye Res. 2018;176:130–140.
- Mandal A, Bisht R, Rupenthal ID, et al. Polymeric micelles for ocular drug delivery: from structural frameworks to recent preclinical studies. J Control Release. 2017;248:96–116.
- Alvarez-Rivera F, Fernandez-Villanueva D, Concheiro A, et al. α-Lipoic acid in Soluplus® polymeric nanomicelles for ocular treatment of diabetes-associated corneal diseases. J Pharm Sci. 2016;105:2855–2863.
- Loftsson T, Stefansson E. Cyclodextrins and topical drug delivery to the anterior and posterior segments of the eye. Int J Pharm. 2017;531:413–423.
- Alvarez-Rivera F, Concheiro A, Alvarez-Lorenzo C. Epalrestat-loaded silicone hydrogels as contact lenses to address diabetic-eye complications. Eur J Pharm Biopharm. 2018;122:126–136.
- Alvarez-Rivera F, Serro AP, Silva D, et al. Hydrogels for diabetic eyes: naltrexone loading, release profiles and cornea penetration. Mater Sci Eng C. 2019;105:110092.
- Alvarez-Rivera F, Rey-Rico A, Venkatesan JK, et al. Controlled release of rAAV vectors from APMA-functionalized contact lenses for corneal gene therapy. Pharmaceutics. 2020;12:335.
- Huang X, Chau Y. Investigating impacts of surface charge on intraocular distribution of intravitreal lipid nanoparticles. Exp Eye Res. 2019;186:107711.
- Ryoo NK, Lee J, Lee H, et al. Therapeutic effects of a novel siRNA-based anti-VEGF (siVEGF) nanoball for the treatment of choroidal neovascularization. Nanoscale. 2017;9:15461–15469.
- Lai S, Wei Y, Wu Q, et al. Liposomes for effective drug delivery to the ocular posterior chamber. J Nanobiotechnol. 2019;17:64.
- Akter N, Radiman S, Mohamed F, et al. Self-assembled potential bio nanocarriers for drug delivery. Mini Rev Med Chem. 2013;13:1327–1339.
- Lu Y, Yue Z, Xie J, et al. Micelles with ultralow critical micelle concentration as carriers for drug delivery. Nat Biomed Eng. 2018;2:318–325.
- Kapoor Y, Howell BA, Chauhan A. Liposome assay for evaluating ocular toxicity of surfactants. Invest Ophthalmol Vis Sci. 2009;50:2727–2735.
- Garaszczuk IK, Montes Mico R, Iskander DR, et al. The tear turnover and tear clearance tests – a review. Expert Rev Med Dev. 2018;15:219–229. .
- Owen SC, Chan DPY, Shoichet MS. Polymeric micelle stability. Nano Today. 2012;7:53–65.
- Söderman O, Herrington KL, Kaler EW, et al. Transition from micelles to vesicles in aqueous mixtures of anionic and cationic surfactants. Langmuir. 1997;13:5531–5538.
- Dutta R, Ghosh S, Banerjee P, et al. Micelle-vesicle-micelle transition in aqueous solution of anionic surfactant and cationic imidazolium surfactants: alteration of the location of different fluorophores. J Colloid Interf Sci. 2017;490:763–773.
- Afri M, Alexenberg C, Bodner E, et al. NMR-based molecular ruler for determining the depth of intercalants within the lipid bilayer. Part V: a comparison of liposomes, bioliposomes and erythrocyte ghosts. Chem Phys Lipids. 2014;184:52–60.
- Israelachvili JN, Mitchell DJ, Ninham BW. Theory of self-assembly of lipid bilayers and vesicles. BBA - Biomembr. 1977;470:185–201.
- Segota S, Tezak D. Spontaneous formation of vesicles. Adv Colloid Interface Sci. 2006;121:51–75.
- Nagarajan R. Molecular packing parameter and surfactant self-assembly: the neglected role of the surfactant tail. Langmuir. 2002;18:31–38.
- Pasquali RC, Taurozzi MP, Bregni C. Some considerations about the hydrophilic – lipophilic balance system. Int J Pharm. 2008;356:44–51.
- Moghassemi S, Hadjizadeh A. Nano-niosomes as nanoscale drug delivery systems: an illustrated review. J Control Release. 2014;185:22–36.
- Bodratti AM, Alexandridis P. Amphiphilic block copolymers in drug delivery: advances in formulation structure and performance. Expert Opin Drug Deliv. 2018;15:1085–1104.
- Polat H, Kutluay G, Polat M. Analysis of dilution induced disintegration of micellar drug carriers in the presence of inter and intra micellar species. Colloid Surf A. 2020;301:124989.
- Kikuchi H, Carlsson A, Yachi K, et al. Possibility of heat sterilization of liposomes. Chem Pharm Bull. 1991;39:1018–1022.
- Hwang D, Ramsey JD, Kabanov AV. Polymeric micelles for the delivery of poorly soluble drugs: from nanoformulation to clinical approval. Adv Drug Deliv Rev. 2020;156:80–118.
- Ajith TA. Alpha-lipoic acid: a possible pharmacological agent for treating dry eye disease and retinopathy in diabetes. Clin Exp Pharmacol Physiol. 2020;47:1883–1890.
- Alambiaga-Caravaca AM, Calatayud-Pascual MA, Rodilla V, et al. Micelles of progesterone for topical eye administration: interspecies and intertissues differences in ex vivo ocular permeability. Pharmaceutics. 2020;12:702.
- Safwat MA, Mansour HF, Hussein AK, et al. Polymeric micelles for the ocular delivery of triamcinolone acetonide: preparation and in vivo evaluation in a rabbit ocular inflammatory model. Drug Deliv. 2020;27:1115–1124.
- Di Prima G, Saladino S, Bongiovi F, et al. Novel inulin-based mucoadhesive micelles loaded with corticosteroids as potential transcorneal permeation enhancers. Eur J Pharm Biopharm. 2017;117:385–399.
- Gote V, Mandal A, Alshamrani M, et al. Self-assembling tacrolimus nanomicelles for retinal drug delivery. Pharmaceutics. 2020;12:1072.
- Patel S, Garapati C, Chowdhury P, et al. Development and evaluation of dexamethasone nanomicelles with potential for treating posterior uveitis after topical application. J Ocul Pharmacol Ther. 2015;31:215–227.
- Nagaraj R, Stack T, Yi SJ, et al. High density display of an anti-angiogenic peptide on micelle surfaces enhances their inhibition of αvβ3 integrin-mediated neovascularization in vitro. Nanomaterials. 2020;10:581.
- Hou Y, Xin M, Li Q, et al. Glycyrrhizin micelle as a genistein nanocarrier: synergistically promoting corneal epithelial wound healing through blockage of the HMGB1 signaling pathway in diabetic mice. Exp Eye Res. 2021;204:108454.
- Ahmed KS, Hussein SA, Ali AH, et al. Liposome: composition, characterisation, preparation, and recent innovation in clinical applications. J Drug Target. 2019;27:742–761.
- Torchilin VP. Recent advances with liposomes as pharmaceutical carriers. Nat Rev Drug Discov. 2005;4:145–160.
- Khan AA, Allemailem KS, Almatroodi SA, et al. Recent strategies towards the surface modification of liposomes: an innovative approach for different clinical applications. 3 Biotech. 2020;10:163.
- Bogdanov P, Sampedro J, Solà-Adell C, et al. Effects of liposomal formulation of citicoline in experimental diabetes-induced retinal neurodegeneration. Int J Mol Sci. 2018;19:2458.
- Parravano M, Scarinci F, Parisi V, et al. Citicoline and vitamin B12 eye drops in type 1 diabetes: results of a 3-year pilot study evaluating morpho-functional retinal changes. Adv Ther. 2020;37:1646–1663.
- Altamirano-Vallejo JC, Navarro-Partida J, Gonzalez-de La Rosa A, et al. Characterization and pharmacokinetics of triamcinolone acetonide-loaded liposomes topical formulations for vitreoretinal drug delivery. J Ocul Pharmacol Ther. 2018;34:416–425.
- Gonzalez-de La Rosa A, Navarro-Partida J, Altamirano-Vallejo JC, et al. Novel triamcinolone acetonide-loaded liposomes topical formulation for the treatment of cystoid macular edema after cataract surgery: a pilot study. J Ocul Pharmacol Ther. 2019;35:106–115.
- Li J, Cheng T, Tian Q, et al. A more efficient ocular delivery system of triamcinolone acetonide as eye drop to the posterior segment of the eye. Drug Deliv. 2019;26:188–198.
- Lai S, Wei Y, Wu Q, et al. Liposomes for effective drug delivery to the ocular posterior chamber. J Nanobiotechnol. 2019;17:64.
- Davis BM, Normando EM, Guo L, et al. Topical delivery of avastin to the posterior segment of the eye in vivo using annexin A5-associated liposomes. Small. 2014;10:1575–1584.
- Giambanco I, Pula G, Ceccarelli P, et al. Immunohistochemical localization of annexin V (CaBP33) in rat organs. J Histochem Cytochem. 1991;39:1189–1198.
- Urtti A. Comment on “Topical Delivery of Avastin to the Posterior Segment of the Eye In Vivo Using Annexin A5-Associated Liposomes”: topical Liposomal Bevacizumab Results in Negligible Retinal Concentrations. Small. 2019;15:1805199.
- Malakouti-Nejad M, Bardania H, Aliakbari F, et al. Formulation of nanoliposome-encapsulated bevacizumab (Avastin): statistical optimization for enhanced drug encapsulation and properties evaluation. Int J Pharm. 2020;590:119895.
- Platania CBM, Fisichella V, Fidilio A, et al. Topical ocular delivery of TGF-β1 to the back of the eye: implications in age-related neurodegenerative diseases. Int J Mol Sci. 2017;18:2076.
- Soriano-Romaní L, Alvarez-Trabado J, López-García A, et al. Improved in vitro corneal delivery of a thrombospondin-1-derived peptide using a liposomal formulation. Exp Eye Res. 2018;167:118–121.
- Peeters L, Sanders NN, Jones A, et al. Post-pegylated lipoplexes are promising vehicles for gene delivery in RPE cells. J Control Release. 2007;121:208–217.
- Lajunen T, Hisazumi K, Kanazawa T, et al. Topical drug delivery to retinal pigment epithelium with microfluidizer produced small liposomes. Eur J Pharm Sci. 2014;62:23–32.
- Hanjdani-Vila RM, Ribier A, Rondot B, et al. Dispersions of lamellar phases of non‐ionic lipids in cosmetic products. Int J Cosmet Sci. 1979;1:303–314.
- Biswas GR, Majee S. Niosomes in ocular drug delivery. Eur J Pharm Med Res. 2018;4:813–819.
- Marianecci C, Di Marzio L, Rinaldi F, et al. Niosomes from 80s to present: the state of the art. Adv Colloid Interface Sci. 2014;205:187–206.
- Ritwiset A, Krongsuk S, Roy J. Molecular structure and dynamical properties of niosome bilayers with and without cholesterol incorporation: a molecular dynamics simulation study. Appl Surf Sci. 2016;380:23–31.
- Marianecci C, Di Marzio L, Del Favero E, et al. Niosomes as drug nanovectors: multiscale pH-dependent structural response. Langmuir. 2016;32:1241–1249.
- Mandal D, Das S. Glucose-triggered dissolution of phenylboronic acid-functionalized cholesterol-based niosomal self-assembly for tuneable drug release. New. J. Chem. 2019;43:7855–7865.
- Baillie AJ, Florence AT, Hume LR, et al. The preparation and properties of niosomes—non‐ionic surfactant vesicles. J Pharm Pharmacol. 1985;37(12):863–868.
- Machado ND, Garcia-Manrique P, Fernandez MA, et al. Cholesterol free niosome production by microfluidics: comparative with other conventional methods. Chem Eng Res Des. 2020;162:162–171.
- Khatoon M, Shah KU, Din FU, et al. Proniosomes derived niosomes: recent advancements in drug delivery and targeting. Drug Deliv. 2017;24:56–69.
- Pardakhty A, Moazeni E, Varshosaz J, et al. Pharmacokinetic study of niosome-loaded insulin in diabetic rats. DARU. 2011;19:404–411.
- Hasan AA, Madkor H, Wageh S. Formulation and evaluation of metformin hydrochloride-loaded niosomes as controlled release drug delivery system. Drug Deliv. 2013;20:120–126.
- Samed N, Sharma V, Sundaramurthy A. Hydrogen bonded niosomes for encapsulation and release of hydrophilic and hydrophobic anti-diabetic drugs: an efficient system for oral anti-diabetic formulation. Appl Surf Sci. 2018;449:567–573.
- Kamble B, Talreja S, Gupta A, et al. Development and biological evaluation of Gymnema sylvestre extract-loaded nonionic surfactant-based niosomes. Nanomedicine. 2013;8:1295–1305.
- Abdelkader H, Ismail S, Kamal A, et al. Preparation of niosomes as an ocular delivery system for naltrexone hydrochloride: physicochemical characterization. Pharmazie. 2010;65:811–817.
- Uchegbu IF, Vyas SP. Non-ionic surfactant based vesicles (niosomes) in drug delivery. Int J Pharm. 1998;172:33–70.
- Abdelkader H, Ismail S, Kamal A, et al. Design and evaluation of controlled-release niosomes and discomes for naltrexone hydrochloride ocular delivery. J Pharmaceutical Sci. 2011;100(5):1833–1846.
- El-Sayed MM, Hussein AK, Sarhan HA, et al. Flurbiprofen-loaded niosomes-in-gel system improves the ocular bioavailability of flurbiprofen in the aqueous humor. Drug Dev Ind Pharm. 2017;43:902–910.
- Fathalla D, Fouad EA, Soliman GM. Latanoprost niosomes as a sustained release ocular delivery system for the management of glaucoma. Drug Dev Ind Pharm. 2020;46:806–813.
- Fouda NH, Abdelrehim RT, Hegazy DA, et al. Sustained ocular delivery of dorzolamide-HCl via proniosomal gel formulation: in-vitro characterization, statistical optimization, and in-vivo pharmacodynamic evaluation in rabbits. Drug Deliv. 2018;25:1340–1349.
- Emad Eldeeb A, Salah S, Ghorab M. Proniosomal gel-derived niosomes: an approach to sustain and improve the ocular delivery of brimonidine tartrate; formulation, in-vitro characterization, and in-vivo pharmacodynamic study. Drug Deliv. 2019;26:509–521.
- Al Qtaish N, Gallego I, Villate-Beitia I, et al. Niosome-based approach for in situ gene delivery to retina and brain cortex as immune-privileged tissues. Pharmaceutics. 2020;12:198.
- Villate-Beitia I, Gallego I, Martinez-Navarrete G, et al. Polysorbate 20 non-ionic surfactant enhances retinal gene delivery efficiency of cationic niosomes after intravitreal and subretinal administration. Int J Pharm. 2018;550:388–397.
- Qin Y, Tian Y, Liu Y, et al. Hyaluronic acid-modified cationic niosomes for ocular gene delivery: improving transfection efficiency in retinal pigment epithelium. J Pharm Pharmacol. 2018;70:1139–1151.