ABSTRACT
Introduction
Drugs for the treatment of respiratory diseases are commonly administered by oral inhalation. Yet surprisingly little is known about the pulmonary pharmacokinetics of inhaled molecules. Nuclear medicine imaging techniques (i.e. planar gamma scintigraphy, single-photon emission computed tomography [SPECT] and positron emission tomography [PET]) enable the noninvasive dynamic measurement of the lung concentrations of radiolabeled drugs or drug formulations. This review discusses the potential of nuclear medicine imaging techniques in inhalation biopharmaceutical research.
Areas covered
(i) Planar gamma scintigraphy studies with radiolabeled inhalation formulations to assess initial pulmonary drug deposition; (ii) imaging studies with radiolabeled drugs to assess their intrapulmonary pharmacokinetics; (iii) receptor occupancy studies to quantify the pharmacodynamic effect of inhaled drugs.
Expert opinion
Imaging techniques hold potential to bridge the knowledge gap between animal models and humans with respect to the pulmonary disposition of inhaled drugs. However, beyond the mere assessment of the initial lung deposition of inhaled formulations with planar gamma scintigraphy, imaging techniques have rarely been employed in pulmonary drug development. This may be related to several technical challenges encountered with such studies. Considering the wealth of information that can be obtained with imaging studies their use in inhalation biopharmaceutics should be further investigated.
1. Introduction
Drugs for the treatment of respiratory diseases are commonly administered by oral inhalation [Citation1,Citation2]. This offers the advantage of delivering high local drug doses to the lungs while minimizing systemic exposure and side effects. In addition, the pulmonary route can be used to deliver medicines to the systemic circulation [Citation3]. The human respiratory tract consists of a conducting and respiratory zone with 23 airway generations reaching down from the trachea to the alveolar sacs [Citation4]. The thickness of the respiratory epithelium decreases from the trachea-bronchial region to the alveolus. The efficacy of drug delivery to the lungs is governed by an interplay of several different factors, i.e. physicochemical properties of the drug itself, formulation of the drug, the employed inhalation device (e.g. dry powder inhalers, pressurized metered dose inhalers, nebulizers or soft-mist inhalers) as well as patient- and disease-related factors (e.g. breathing pattern, airway constriction). The rate and extent of systemic absorption of inhaled drugs depends largely on the site of deposition (peripheral versus central lungs), whereby the thin respiratory epithelium and the large surface area (~100 m2) of the alveolar region favors drug absorption into the systemic circulation [Citation5]. Similar to drugs intended for other disease conditions, the magnitude of the free (non-protein bound) concentration-time profile in the tissue targeted for treatment is in most cases directly related to the pharmacodynamic effect. For inhaled drugs, the target tissue is located in respiratory tissues, even though the effect site within tissue may differ from drug to drug [Citation2]. It may for instance comprise the airway lumen for some antibiotics, membrane-bound receptors on airway smooth muscle cells (e.g. β2-adrenoceptor agonists and muscarinic acetylcholine receptor antagonists) or intracellular receptors (glucocorticoids). Some drugs may preferentially target the central airways (muscarinic acetylcholine receptor antagonists) while other drugs may preferentially target the peripheral airways (β2-adrenoceptor agonists), which needs to be considered in the choice and design of an inhalation formulation/device (e.g. particle size distribution). Efforts are being made in the development of respiratory drugs to increase drug retention in lung tissues (e.g. by decreasing solubility or membrane permeability or by increasing nonspecific binding or lysosomal trapping in lung tissue), while at the same time minimizing both systemic absorption of the drug from the lungs as well as oral bioavailability of the swallowed drug fraction [Citation1]. This means that the ideal characteristics of an inhaled lung-targeted drug molecule will substantially differ from a drug molecule that is intended for oral administration [Citation6]. While for orally administered drugs blood or plasma concentrations are often used as a surrogate to estimate drug tissue concentrations, this is not possible for inhalation drugs, as the blood compartment is downstream from the lungs. In addition, both drug absorbed from the lungs and drug absorbed from the gastrointestinal tract may contribute to plasma concentrations after inhalation delivery, so that plasma concentrations are unlikely to reflect lung tissue concentrations.
The biggest challenge inhaled drugs face as moving toward approval are pivotal pharmacokinetic studies; historically the pass rate in these studies is <50%. Failure in pharmacokinetic studies leads to at least one further round of re-optimization and clinical evaluation that can add several months of additional development time. This incremental project time leads to delays in launch, increased project cost and ultimately a lack of access to essential medicines for patients. Due to several different factors preclinical animal models often serve as poor predictors of drug delivery to the human lungs [Citation1]. To improve the pass rate in pivotal pharmacokinetic studies, a critical knowledge gap is understanding how formulation, morphological and process differences in drug product lead to differences in drug disposition in the lung.
Apart from bronchoalveolar lavage and tissue biopsies, each with its own limitations [Citation7], it is very difficult to measure lung tissue concentrations of drugs in humans. Against this background, noninvasive nuclear medicine imaging techniques bear great potential. These include planar gamma scintigraphy, single-photon emission computed tomography (SPECT) and positron emission tomography (PET). Nuclear medicine imaging techniques allow to dynamically measure the lung concentrations of radiolabeled molecules, so-called radiotracers. There are three approaches how these imaging techniques can be used in the context of pulmonary drug delivery (). The first approach involves the radiolabeling of a component of the drug formulation (e.g. solid particles or solvent) under the assumption that this component will behave similarly to the drug contained in the formulation. This approach is frequently applied in lung deposition studies, to quantify the initial lung-deposited dose and its regional deposition pattern after oral inhalation. The second approach involves radiolabeling of the drug molecule itself. This approach is technically more challenging due to the necessity to develop a radiolabeling strategy for the drug of interest and to introduce the radiolabeled drug into the inhalation device, but it offers the great advantage that, apart from initial lung deposition, it also allows assessing retention of the drug in the lungs over time as well as clearance processes from the lungs. The third approach employs an imaging biomarker for the effect of the inhaled drug, such as target receptor occupancy. While such an approach will provide valuable data related to the efficacy of drug delivery to the lungs, it relies on knowledge of the actual drug target and the availability of a radiotracer to measure the binding of the inhalation drug to this target. Lung deposition studies with planar gamma scintigraphy have been thoroughly covered in previous review articles [Citation8–11] and will, therefore, only be briefly discussed. In the present article, we will place an emphasis on studies, in which the drug molecule itself has been radiolabeled to assess its pulmonary disposition by means of imaging.
Figure 1. Illustration of the three approaches how nuclear medicine imaging methods can be used to assess pulmonary drug disposition: (i) planar gamma scintigraphy with radiolabeled inhalation formulations to assess initial pulmonary drug deposition, (ii) PET imaging studies with radiolabeled drugs to assess their intrapulmonary pharmacokinetics and (iii) receptor occupancy studies to quantify the pharmacodynamic effect of an inhaled drug. This figure was partly generated using Servier Medical Art, provided by Servier, licensed under a Creative Commons Attribution 3.0 unported license.
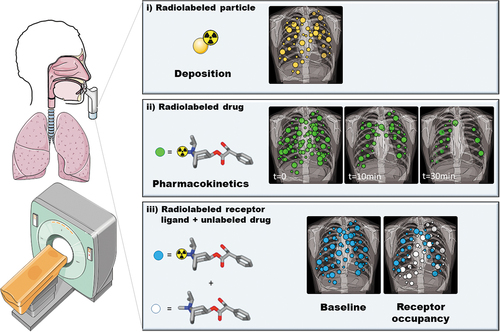
2. Pulmonary drug deposition studies with planar gamma scintigraphy/SPECT
Both planar gamma scintigraphy and SPECT employ gamma-emitting radionuclides with technetium-99 m (99mTc, radioactive half-life: 6.01 hours) being the most commonly used radionuclide [Citation9]. Gamma scintigraphy involves planar two-dimensional imaging, which provides good temporal resolution but poor quantification of radioactivity due to difficulties to correct the emitted radiation for scatter and tissue attenuation [Citation9]. Depending on the radiotracer employed, high temporal resolution may be of importance to measure initial deposition patterns, before pulmonary absorption of the radiotracer from the airways occurs. Planar gamma scintigraphy accounts for the majority of inhaled drug imaging applications and is often used for marketing purposes of already approved drugs to display regional lung deposition patterns or to compare different inhalation devices in the same subjects [Citation8]. SPECT, on the other hand, provides three-dimensional images with good quantifiability. However, due to low sensitivity, a SPECT acquisition takes time, so that rapidly changing distributions of activity over time cannot be measured with good temporal resolution. The combination of SPECT with computed tomography (CT) helps localize the anatomic structure from which the signal originates and also allows for attenuation correction. For lung studies, radiolabeling for planar gamma scintigraphy and SPECT relies almost exclusively on 99mTc. Because technetium is not contained in drug molecules, it is physically not possible to radiolabel the drug molecule itself without altering its chemical structure. Therefore, it is in general the formulation rather than the drug itself which is radiolabeled with 99mTc. The complexity of radiolabeling depends on the employed formulation. If the formulation is a solution, then the 99mTc-labeled compound can simply be dissolved. Here, the chemical form of 99mTc and the rate of its absorption into lung tissue governs the time window during which initial deposition of the aerosolized solution containing the drug can be imaged. The employed 99mTc-labeled compounds range from [99mTc]pertechnetate with a short pulmonary absorption half-life (approximately 15 min) [Citation12] and [99mTc]Tc-DTPA ([99mTc]technetium-diethylene triamine pentaacetic acid) [Citation13,Citation14] to 99mTc-labeled human albumin colloids [Citation15]. For formulations which contain solid particles, radiolabeling is more complex [Citation9]. A typical approach to labeling dry powders is by adsorbing [99mTc]pertechnetate on the surface of the drug particles in such a way that the radiolabel is evenly distributed across the particles in a similar fashion as the drug and remains tightly bound to the particles [Citation16–19]. Moreover, the radiolabeled particles need to be introduced into the inhaler after the radiolabeling. This labeling approach also requires some validation studies to prove that the radiolabeled formulation behaves identically to the unlabeled formulation, e.g. by using an impactor or impinger to measure particle size distributions. In principle, lung deposition studies may also be performed with PET, for which 2-deoxy-2-[18F]fluoro-D-glucose ([18F]FDG) could be used to label the drug formulation [Citation20]. There is a wealth of studies in the literature employing planar gamma scintigraphy to assess pulmonary deposition of inhaled drug formulations in humans covering different drug classes, such as β2-adrenoceptor agonists [Citation16,Citation21–28], glucocorticoids [Citation17,Citation29,Citation30], muscarinic acetylcholine receptor antagonists [Citation19,Citation31], combination products [Citation32–35], antibiotics [Citation36,Citation37] and insulin [Citation18,Citation38]. In addition, a few studies have employed SPECT to assess lung deposition [Citation39,Citation40]. However, in none of these studies the drug itself has been radiolabeled, so that the imaging study could only assess the initial lung deposition of the formulation. A detailed review of these studies is beyond the scope of the present article.
3. Positron emission tomography (PET)
PET uses positron-emitting radionuclides such as carbon-11 (11C, half-life: 20.4 min) or fluorine-18 (18F, half-life: 109.8 min) and provides fully quantitative three-dimensional images with a temporal resolution in the order of seconds to minutes. The available PET radionuclides offer the opportunity to radiolabel drug molecules without changing their chemical structure, so that the disposition of the drug itself in the lungs can be assessed rather than the initial deposition of the formulation as it is done with planar gamma scintigraphy. However, nuclear medicine imaging methods measure total radioactivity per volume unit and cannot distinguish radiolabeled parent drug from radiolabeled metabolites. If extensive metabolism of a radiolabeled drug occurs during the time course of the imaging experiment, the imaging signal may represent a mixture of different radiolabeled entities, which will complicate data interpretation. With respect to the labeling of low molecular weight drugs, 11C is the radionuclide of choice as virtually every drug molecule contains carbon in its chemical structure, so that radiolabeling can proceed without structural modification of the drug of interest. A challenge with respect to 11C is its short radioactive half-life (i.e. 20.4 min), which limits the observation period to a maximum of 1.5–2 hours. 18F, on the other hand, has a more practical radioactive half-life (i.e. 109.8 min), but a limitation comes from the fact that only a fraction of drug molecules contains fluorine in their chemical structure. For PET radiolabeling, dedicated research infrastructure is needed, which must often include a cyclotron for on-site production of short-lived radionuclides such as 11C. Details of PET radiochemistry have been thoroughly covered elsewhere [Citation41–44] and will not be subject of this review article.
4. Imaging studies with radiolabeled drugs to assess pulmonary drug disposition
The concept of using imaging to assess the tissue distribution and pharmacokinetics of radiolabeled drugs has been termed ‘pharmacokinetic imaging’ [Citation45,Citation46] and offers valuable information related to drug disposition, which cannot be obtained in conventional pharmacokinetic studies, in which drug concentrations are most commonly only assessed in plasma. The amount of mass associated with PET and SPECT radiotracers is usually only in the range of a few micrograms, which satisfies the definition of a microdose set forth by regulatory authorities and therefore requires a reduced non-clinical safety testing package compared to conventional phase 1 studies [Citation47]. Imaging studies with radiolabeled drugs both small-molecules and biologicals such as antibodies, have been conducted, e.g. to assess the distribution of drugs to the tissue targeted for treatment [Citation48–55]. In these studies, the radiolabeled drugs were usually administered by intravenous (i.v.) administration. Depending on the scanned body segment of the subject and the axial field of view (FOV) of the used scanner, lung tissue is also accessible to imaging-based pharmacokinetic analysis [Citation50,Citation55]. A considerably lower number of studies has administered radiolabeled drugs by oral inhalation in humans and used imaging to assess the pulmonary disposition of these drugs. The administration of radiotracers as inhaled aerosols has a firmly established role in diagnostic nuclear medicine (ventilation-perfusion scans) [Citation56], so that this methodology can be harnessed to assess pulmonary drug disposition. A methodological challenge with the use of nuclear medicine imaging techniques to assess pulmonary drug disposition is related to the fact that imaging measures total radioactivity per volume unit and cannot distinguish different sub-compartments in lung tissue in which the drug may be located (e.g. epithelial lining fluid, epithelial cells, interstitial space, blood vessels, macrophages). Moreover, protein- or lipid-bound drug cannot be distinguished from unbound drug based on the imaging signal. It may, thus, be challenging to derive the pharmacologically active free drug concentration, to which the molecular target of the drug is exposed (e.g. receptor proteins, such a β2-adrenoceptors or muscarinic acetylcholine receptors), from the total tissue radioactivity signal obtained with imaging. For instance, the same total concentration of a radiolabeled drug in the lungs measured with imaging may lead to different pharmacodynamic effects depending on whether, e.g. 10% or 90% of the drug is trapped in intracellular lysosomes. However, novel techniques are available to measure the tissue binding of drugs in lung tissue, e.g. precision-cut lung slices [Citation57], which can provide correction factors that may be applied to imaging data to obtain the free drug concentration in lung tissue, in analogy to studies of the brain distribution of drugs [Citation58]. When the temporal profile of lung tissue retention and clearance of the drug from the lungs is of primary interest, imaging may offer valuable information, notwithstanding the lack of precise knowledge of the free drug concentration at its specific target site. Probably due to these and other technical challenges, only relatively few studies have so far assessed the pulmonary disposition of inhaled radiolabeled drugs, which will be discussed in the following section. gives an overview of the chemical structures of the radiolabeled molecules which have been studied so far.
Figure 2. Chemical structures of radiolabeled drugs for which pulmonary disposition has been assessed with nuclear medicine imaging methods.
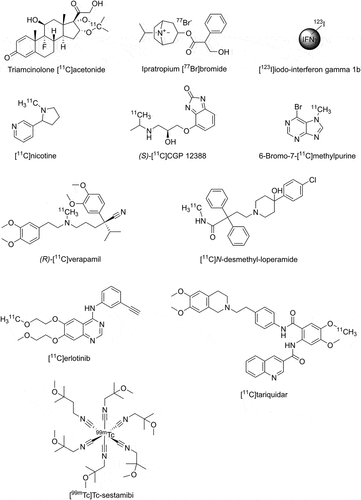
Berridge et al. performed PET studies with the 11C-labeled glucocorticoid triamcinolone [11C]acetonide () administered to healthy volunteers with a custom-made metered dose inhaler without or with use of a spacer [Citation59]. Triamcinolone [11C]acetonide was synthesized by reaction of [11C]acetone and triamcinolone [Citation60] and the radiolabeled drug dissolved in ethanol was added to the metered dose inhaler via a rubber septum [Citation59]. The particle size distribution of radioactivity emitted from the inhalation device was determined with a cascade impactor to ensure that the formulation containing radiolabeled drug met the specifications of the unlabeled formulation. The employed PET scanner had an axial FOV of 15 cm, so that three consecutive bed positions had to be imaged to cover the oropharyngeal region, the airways and the whole lungs. Dynamic imaging was performed up to a total length of 90 min after drug administration in order to measure the clearance of radiolabeled drug from the lungs. PET data were co-registered with CT data obtained on a separate scanner and different volumes of interest were defined. The study revealed markedly decreased (−40%) oral deposition of radioactivity when the spacer was used which resulted in 4-fold increased deposition in target tissues (bronchi and peripheral lung), corresponding to approximately 14% of the inhaled dose (). Reduced oral deposition is expected to reduce systemic side effects caused by gastrointestinal absorption of the swallowed drug. Kinetic analysis revealed a markedly faster elimination of radioactivity from the conducting airways as compared with the peripheral lungs, which was attributed to mucociliary clearance of the deposited particles. A limitation of the employed approach is that PET analysis does not allow distinguishing the signal arising from drug deposited in the airways and dissolved drug made available to the pharmacological target (glucocorticoid receptors). Of interest, the same group directly compared the pulmonary deposition measured with either triamcinolone [11C]acetonide PET or with planar gamma scintigraphy using the [99mTc]pertechnetate-labeled formulation in the same subjects [Citation9,Citation61]. They found that planar gamma scintigraphy, without the application of correction methods, markedly overestimated the peripheral-to-central deposition pattern, and that elimination kinetics of radioactivity from the lungs substantially differed between the two imaging modalities, as was expected given the different employed radiolabeled entities [Citation9,Citation61]. PET was also used by the same group to assess the nasal deposition and residence time of triamcinolone [11C]acetonide delivered via two different nasal drug delivery devices in healthy volunteers [Citation62,Citation63]. Two studies used planar gamma scintigraphy to assess pulmonary disposition of radiolabeled drugs, i.e. one small molecule [Citation64] and one biological [Citation65]. In the first study, the muscarinic acetylcholine receptor antagonist ipratropium bromide was radiolabeled with the gamma-emitting radionuclide bromine-77 (77Br, half-life: 56 hours) () and introduced into a metered dose inhaler to study its pulmonary deposition in healthy control subjects and patients with chronic bronchitis [Citation64]. However, the potential value of direct drug labeling was diminished by the fact that only the counter ion of the drug salt was radiolabeled so that pulmonary residence and clearance of the drug itself could not be measured. This study revealed no major differences between patients and control subjects in terms of lung-deposited dose (approximately 11%) and regional deposition patterns in the lungs. This study underlined the potential of imaging to assess the effect of disease on pulmonary disposition of inhaled drugs, which is far from completely understood. The radiolabeling of ipratropium bromide by 11C-methylation of the quaternary ammonium moiety has also been reported [Citation66] and this may be a more appropriate labeling approach to assess pulmonary disposition of ipratropium, but no clinical imaging results have been reported yet. In the other study, the pulmonary deposition of inhaled aerosolized iodine-123 (123I, half-life: 13.22 hours) labeled interferon gamma 1b (), an immunomodulatory cytokine for treatment of chronic granulomatous disease, was assessed in healthy volunteers by planar gamma scintigraphy [Citation65]. The study demonstrated a long residence time of the inhaled radiolabeled drug in the lungs and satisfactory deposition in target tissue in the peripheral lung and low systemic absorption of the unlabeled cytokine. In addition, whole-body planar gamma scintigraphy was used to measure urinary and hepatobiliary excretion of radioactivity. The radiation dose of the radiotracer was also assessed, which amounted to an effective dose of 9 mSv for an inhaled activity of 180 MBq. This study was unique in the sense that it assessed lung deposition of a protein drug in humans, which was directly radiolabeled with a gamma-emitting radionuclide. However, the study did not comprehensively assess the in vivo stability of the radiolabeled protein and the observed excretion pattern may reflect that of free radioiodine rather than that of the protein itself. An interesting option for future studies to assess the disposition of inhaled biologicals may be the use of long axial FOV PET systems [Citation67] in combination with zirconium-89 (89Zr, half-life: 3.27 days) labeled drugs employing chelators with good in vivo stability. The radiolabeling of the glucocorticoid fluticasone propionate with 18F has been reported and its incorporation into a pressurized metered dose inhaler for lung deposition studies in humans [Citation68], but no imaging results have so far appeared in the literature.
Figure 3. PET images of triamcinolone [11C]acetonide deposition (green color) in the lungs of one healthy volunteer. Triamcinolone [11C]acetonide was administered with a metered dose inhaler without (A) and with (B) the use of a spacer during inhalation. Note the increased deposition in the bronchi and peripheral lung when the spacer was used. This research was originally published in JNM. Marc S. Berridge, Zhenghong Lee, and Donald L. Heald. Pulmonary Distribution and Kinetics of Inhaled [11C]Triamcinolone acetonide. J. Nucl. Med. 2000;41:1603–1611 [Citation59].
![Figure 3. PET images of triamcinolone [11C]acetonide deposition (green color) in the lungs of one healthy volunteer. Triamcinolone [11C]acetonide was administered with a metered dose inhaler without (A) and with (B) the use of a spacer during inhalation. Note the increased deposition in the bronchi and peripheral lung when the spacer was used. This research was originally published in JNM. Marc S. Berridge, Zhenghong Lee, and Donald L. Heald. Pulmonary Distribution and Kinetics of Inhaled [11C]Triamcinolone acetonide. J. Nucl. Med. 2000;41:1603–1611 [Citation59].](/cms/asset/44cb703c-5871-411e-a651-847204d30d87/iedd_a_2137143_f0003_oc.jpg)
A few studies used PET to assess the pulmonary disposition of inhaled [11C]nicotine () in humans. Assessment of pulmonary [11C]nicotine disposition is particularly challenging as compared with drugs which are intended to exert their pharmacological effect in the lungs, due to its very fast pulmonary absorption kinetics. In an early study, Bergström et al. used PET to measure the deposition pattern of [11C]nicotine released from a nicotine vapor inhaler, developed as a smoking cessation aid, in healthy volunteers and found that a large portion of the inhaled dose (~45%) was deposited in the oral cavity with only a minor portion deposited in the lungs (~5%) which was consistent with buccal rather than pulmonary absorption as the major absorption route of nicotine [Citation69]. However, pharmacokinetic assessment was limited by the short axial FOV of the PET scanner (10 cm), which hampered a complete description of the deposition pattern of the inhaled [11C]nicotine vapor. The same group compared in a follow-up study in one single subject the deposition pattern of [11C]nicotine administered via the vapor inhaler and via a conventional cigarette [Citation70]. This study also included arterial blood sampling. The main finding was a markedly higher pulmonary [11C]nicotine deposition (14% versus 4%) for the cigarette than for the vapor inhaler resulting in a rapid increase in arterial blood concentrations of [11C]nicotine for the cigarette. In an elegant study by Berridge et al. [11C]nicotine was administered via single bolus inhalation to healthy smokers using cigarettes labeled with [11C]nicotine [Citation71]. The [11C]nicotine added to the cigarette behaved as a true tracer of the unlabeled nicotine contained in the cigarette, in that it showed a nearly identical release pattern from the cigarette [Citation72]. The study was unique in that it included dynamic PET scanning with high temporal resolution of the lungs and the brain as a pharmacological target tissue and also measured [11C]nicotine pharmacokinetics in arterial and venous blood [Citation71]. Due to the limited axial FOV of the employed PET scanner (15 cm) the brain and the lung curves had to be recorded in two separate imaging sessions in each subject. The main finding of this study was that [11C]nicotine was rapidly absorbed from the lungs into the blood and that brain concentrations of [11C]nicotine rose very rapidly after cigarette smoke inhalation, which may be a contributing factor to nicotine addiction of smokers. A second study published in the same year measured the brain and lung kinetics of [11C]nicotine administered via cigarette smoke with high temporal resolution in two separate PET scans in dependent and nondependent smokers [Citation73]. This study found a slower rate of brain uptake of [11C]nicotine in dependent than in nondependent smokers, which was a consequence of a slower rate of [11C]nicotine elimination from the lungs of dependent smokers. Intriguingly, a strong correlation was found between the rate of [11C]nicotine elimination from the lungs and the rate of its brain uptake [Citation73]. The same group later on used PET to compare the brain delivery of [11C]nicotine administered via electronic and conventional cigarettes and found a similar velocity of brain entry for both administration modes [Citation74]. However, pulmonary deposition of [11C]nicotine was not measured apart from whole body scans at late time points after inhalation, which mainly served to estimate the total administered dose. Finally, a study by Walls et al. used a newer-generation PET/CT scanner with an axial FOV of 25 cm to compare the lung and brain kinetics (measured in two different imaging sessions) of [11C]nicotine administered via an e-cigarette either in the form of the free base or in the form of the lactate salt [Citation75]. The study found lower deposition of [11C]nicotine in the oral cavity, higher lung deposition and a higher and faster peak in the brain concentration for the lactate salt than for free base.
5. Receptor occupancy studies
Receptor occupancy studies provide an indirect approach to assessing pulmonary drug disposition by measuring a biomarker of drug effect. The drug itself is not radiolabeled but instead a validated radiotracer is used that provides an effect readout. As far as imaging techniques are concerned, target receptor occupancy is the most commonly employed effect biomarker. This approach benefits from ample experience gained with using imaging to assess receptor occupancy for central nervous system (CNS)-targeted drugs. With respect to lung-targeted inhaled drugs, a high degree of pulmonary target receptor occupancy may be indicative of satisfactory lung-targeting properties of the inhaled drug. An advantage of this approach is that it only measures the effect of the pharmacologically active part of the drug in lung tissue as opposed to imaging studies employing the radiolabeled drug, in which the total tissue concentration of the drug is measured, potentially comprising different tissue sub-compartments. Receptor occupancy studies have the potential to bridge the knowledge gap between pharmacokinetics and pharmacodynamics in early respiratory drug development. However, the applicability of this approach relies on the availability of a radiotracer that can measure the binding of the drug of interest to its target receptor. Efforts have been made to develop effective PET radiotracers for pulmonary receptors that are commonly therapeutically targeted (β2-adrenoceptors or muscarinic acetylcholine receptors) [Citation76], but for new therapeutic targets the applicability of this approach may be limited due to the lack of effective radiotracers. Available radiotracers include the β2-antagonist radiotracers (S)-[11C]CGP 12177 and (S)-[11C]CGP 12388 (which both also bind to β1-adrenoceptors), and the muscarinic acetylcholine receptor (M1/M2/M3) antagonist [11C]VC-002. An important pre-requisite for radioligands targeting receptors in the lungs is good metabolic stability, as potential radiolabeled metabolites are expected to be able to freely access lung tissue and may, therefore, confound the interpretation of the imaging signal. While receptor occupancy studies form an integral part of CNS drug development (see, e.g [Citation77,Citation78].), the concept of pulmonary receptor occupancy studies is fairly new. In the few published pulmonary receptor occupancy studies [Citation79–81], the radiotracer was administered i.v., the most common application route of radiotracers, while the drug of interest was administered by inhalation. However, there is also one example in which a PET radioligand for β-adrenoceptors ((S)-[11C]CGP 12388, () was administered to healthy volunteers by inhalation with a jet nebulizer, in an attempt to selectively image airway β2-adrenoceptors (which are the primary pharmacological target site of β2-agonist drugs) [Citation82]. Inhalation of the short acting β2-adrenoceptor agonist salbutamol prior to the PET scan accelerated the washout of radioactivity from different lung regions (peripheral, central, tracheobronchial) without affecting initial lung deposition, which was interpreted as a consequence of β2-adrenoceptor occupancy induced by the inhaled unlabeled drug [Citation82]. In a very informative study by Schou et al. muscarinic acetylcholine receptor occupancy in the lungs of anesthetized cynomolgus monkeys was measured with PET and [11C]VC-002 both after inhalation and i.v. pretreatment with the muscarinic acetylcholine receptor antagonist ipratropium [Citation79]. As opposed to the study with (S)-[11C]CGP 12388 [Citation82], pulmonary receptor occupancy by unlabeled drug (ipratropium) did not accelerate radiotracer washout from the lungs, but rather led to a decrease in the total volume of distribution (VT) of the radiotracer in the lungs, which is equivalent to the radiotracer’s tissue-to-plasma concentration ratio at steady state. Modeling of the data indicated a lower inhibitory constant (Ki) of ipratropium for pulmonary muscarinic receptors based on its plasma concentrations after inhalation than after i.v. administration, i.e. a given ipratropium plasma concentration induced a greater degree of pulmonary receptor occupancy after administration by inhalation than after i.v. administration. This was consistent with lung-targeting of the drug after inhalation. Importantly, the study additionally measured muscarinic receptor occupancy in the pituitary gland, which was within the FOV of the PET scanner and which allowed to assess the systemic effect of the inhaled drug. Due to the large inter-individual differences in radiotracer binding, regional differences in receptor occupancy of the drug in the lungs could not be analyzed in the study by Schou et al. [Citation79]. The same group used [11C]VC-002 to quantify muscarinic acetylcholine receptor binding in the human lungs [Citation83] and a clinical trial to measure pulmonary receptor occupancy of the dual muscarinic receptor antagonist and β2-adrenoceptor agonist AZD2115 in healthy volunteers appears to be underway (ClinicalTrials.gov Identifier: NCT03097380). The study by Maher et al. is the first one which attempted to estimate pulmonary receptor occupancy of a drug candidate (the inhaled alpha-v beta-6 (αvβ6) integrin inhibitor GSK3008348) in a patient population, i.e. in patients with idiopathic pulmonary fibrosis [Citation80]. They employed the αvβ6-selective peptide PET ligand [18F]fluorobenzoyl-A20FMDV2 ([18F]FB-A20FMDV2) and used VT estimated with an image-derived arterial plasma input function corrected for radiolabeled metabolites as a parameter of target specific binding of the radiotracer in the lungs. PET imaging was performed at baseline and at two timepoints after inhalation of GSK3008348 (i.e. 30 min and 24 h) and a moderate reduction of VT (~20%) was observed in the 30 min post-dosing PET scan, after which it returned to baseline values at the 24 h post-dosing scan, which suggested a short duration of action of the investigational drug and sub-optimal lung-targeting properties.
6. Use of imaging to assess the influence of membrane transporters on pulmonary drug disposition
Inhaled drugs have to cross the pulmonary epithelium to reach their effect sites in the lung interstitium (e.g. β2-adrenoceptors or muscarinic acetylcholine receptors on airway smooth muscle cells) [Citation1]. It is generally assumed that pulmonary epithelial cells offer a greater passive permeability than other biological barriers (e.g. intestinal epithelial- or blood-brain barrier), so that pulmonary absorption occurs quickly and efficiently, in particular for lipophilic, low molecular weight drugs [Citation84]. Nevertheless, lung epithelial cells are known to express several different membrane transporters belonging either to the adenosine triphosphate (ATP)-binding cassette (ABC) or the solute carrier (SLC) families [Citation85–87]. ABC transporters are primary active transporters and use the energy of ATP to transport their substrates against concentration gradients out of cells. SLC transporters most commonly mediate the uptake of drugs into cells and are either secondary active transporters, i.e. they use an electrochemical gradient generated by the transport of an ion as an energy source, or facilitative transporters, i.e. they mediate transport along a concentration gradient without the use of metabolic energy. Immunohistochemical analysis and quantitative proteomic-based analysis of lung tissue and primary lung epithelial cells revealed that multidrug resistance-associated protein 1 (MRP1, encoded by the ABCC1 gene), P-glycoprotein (P-gp/ABCB1) and breast cancer resistance protein (BCRP/ABCG2) are among the most abundantly expressed ABC transporters in lung epithelial cells, with P-gp and BCRP located in the apical (airway lumen facing) membrane and MRP1 in the basolateral membrane [Citation86,Citation88]. Among SLC transporters, the organic cation transporters 1 and 3 (OCT1/SLC22A1 and OCT3/SLC22A3) and the novel organic cation transporters 1 and 2 (OCTN1/SLC22A4 and OCTN2/SLC22A5) are abundantly expressed [Citation85]. A wealth of in vitro studies indicate that currently used inhalation drugs interact with pulmonary ABC and SLC transporters [Citation86]. For instance, muscarinic acetylcholine receptor antagonists (e.g. ipratropium, tiotropium and glycopyrronium) are all permanent cations (quaternary ammonium salts) and supposed substrates of OCTs and OCTNs [Citation85]. Based on their localization, epithelial membrane transporters may affect the airway residence time of inhaled drugs, by modulating their uptake into epithelial cells, and control the access of drugs to the interstitium [Citation87]. Apart from epithelial cells, some of these transporters are also expressed at other locations in lung tissue (e.g. alveolar macrophages, seromucinous glands, capillary endothelial cells) which may further influence the intrapulmonary distribution and retention of drugs as well as their elimination into blood. Therefore, pulmonary ABC and SLC transporters may modulate the pulmonary pharmacokinetics and pharmacodynamics of inhaled drugs. However, so far, the effect of transporters on pulmonary drug disposition has been mainly assessed based on in vitro experiments in epithelial cell lines or on ex vivo experiments in isolated perfused lungs [Citation89,Citation90]. The majority of available in vivo studies have only assessed drug concentrations in plasma (and not in lung tissue), which fails to give a complete picture of the effect of transporters on pulmonary drug disposition [Citation86,Citation87]. Again, this is mainly related to difficulties to dynamically measure drug concentrations in the lungs in vivo and this is where imaging methods may come into play. The availability of dedicated small-animal imaging PET and SPECT scanners as well as of rodent models in which the genes encoding certain ABC or SLC transporters are knocked out [Citation91] offers the opportunity to assess transporter effects in a preclinical setting. Transgenic animal models may be preferred over the co-administration of prototypical transporter inhibitors, as the latter often lack selectivity for a given transporter and may exert additional pharmacological effects that may confound the interpretation of the imaging data. One particular challenge associated with rodent studies is related to physiological differences (breathing style) and the impossibility to administer inhaled drugs in the same way as in humans, e.g. by using a sophisticated delivery device such as a metered dose inhaler [Citation1]. Available administration modes for rodents include exposure chambers, in which either the whole animal or only the nose is exposed to an aerosol cloud of the material to be inhaled [Citation1]. Alternative administration modes include intratracheal liquid bolus instillation or intratracheal aerosolization using a range of commercial devices (e.g. Microsprayer® aerosolizer or AeroProbeTM nebulization catheters) [Citation84,Citation92,Citation93]. An advantage of these latter techniques is that it is more straightforward to determine the administered dose of the inhaled compound as compared to inhalation chambers, while a disadvantage stems from the fact that they can only be applied in anesthetized animals, which is unlikely to represent inhalation at a normal breathing rate. Another limitation of rodent models is a different bronchial tree structure and a lack of well-developed respiratory bronchioles as compared to humans [Citation94].
There are a handful of imaging studies that addressed the effects of transporters on pulmonary drug disposition. None of these studies have applied clinically used inhalation drugs, but instead used prototypical transporter substrates that have been developed for other purposes than the imaging of pulmonary transporters. Okamura et al. have developed 6-bromo-7-[11C]methylpurine () as a radiotracer to image the activity of MRP1 in the brain [Citation95]. The radiotracer itself is not a MRP1 substrate, but is rapidly converted in vivo into the corresponding radiolabeled glutathione conjugate via glutathione-S-transferase enzymes. The radiolabeled glutathione conjugate has a low passive permeability and is effluxed from cells by MRP1 [Citation95]. Following i.v. administration of 6-bromo-7-[11C]methylpurine, the elimination of radioactivity from the brain was markedly reduced in Abcc1 knockout mice (Abcc1(-/-)) as compared with wild-type mice, which highlighted the critical role of MRP1 in the brain elimination of the radiolabeled glutathione conjugate [Citation95]. Similar observations have been made for the mouse lungs, from which radioactivity elimination after i.v. radiotracer administration was markedly reduced when Abcc1 was knocked out [Citation96,Citation97]. In order to specifically address the role of MRP1 located in pulmonary epithelial cells, we administered 6-bromo-7-[11C]methylpurine by intratracheal aerosolization to wild-type and Abcc1(-/-) rats [Citation98]. Dynamic PET imaging of the lungs revealed a reduced initial elimination half-life (t1/2,elim) of radioactivity from the lungs and increased lung radioactivity exposure in Abcc1(-/-) rats as compared with wild-type rats (t1/2,elim, wild-type: 1.9 min, Abcc1(-/-) 13 min), which was consistent with a basolateral localization of MRP1 in pulmonary epithelial cells. The radiotracer presumably crossed the apical membrane of pulmonary epithelial cells by passive diffusion, was converted inside the epithelial cells into the radiolabeled glutathione conjugate, which was retained in the epithelial cells in absence of MRP1 [Citation98]. These data suggested that MRP1 may modulate the pulmonary exposure and elimination of inhaled MRP1 substrate drugs. In another study, we used in a similar experimental set-up (R)-[11C]verapamil and [11C]N-desmethyl-loperamide () as radiotracers to assess the effect of P-gp on pulmonary drug disposition [Citation99]. Both radiotracers are substrates of P-gp but not of MRP1 and BCRP and have been developed to image the activity of P-gp at the rodent and human blood-brain barrier [Citation100]. Both radiotracers were intratracheally aerosolized in groups of wild-type rats without and with co-administration of the prototypical P-gp inhibitor tariquidar and in Abcb1a/b(-/-) rats followed by dynamic PET imaging of the lungs [Citation99]. Both radiotracers showed rapid initial elimination from the rat lungs with t1/2,elim values in all studied groups < 10 min, which indicated rapid pulmonary absorption and was probably due to the high lipophilicity of the radiotracers. However, in absence of P-gp activity, either by tariquidar co-administration or by genetic knockout, pulmonary elimination tended to be faster (i.e. t1/2,elim was decreased), which was the opposite of the effects seen for the MRP1 radiotracer [Citation98]. This effect resulted in significant decreases in lung exposure to both radiotracers in absence of P-gp and is consistent with an apical localization of P-gp in lung epithelial cells [Citation99]. These data support the concept that transport by P-gp may retain inhaled drugs in the lungs by re-cycling them between the epithelial cells and the epithelial lining fluid. In another study, we assessed the pulmonary disposition of the dual P-gp/BCRP substrate radiotracers [11C]erlotinib and [11C]tariquidar () in wild-type, Abcb1a/b(-/-) and Abcg2(-/-) rats and in Abcg2(-/-) rats treated with tariquidar [Citation101]. The combined absence of both P-gp and BCRP activity (tariquidar-treated Abcg2(-/-) rats) led to marked (~4-fold) increases in lung exposure to [11C]erlotinib, while absence of P-gp alone (Abcb1a/b(-/-) rats) or BCRP alone (Abcg2(-/-) rats) had only small effects. Interestingly, the effect of transporter knockout/inhibition was opposite to that seen for the P-gp substrate radiotracers (R)-[11C]verapamil and [11C]N-desmethyl-loperamide, which showed decreased lung exposure in Abcb1a/b(-/-) rats and tariquidar-treated wild-type rats. A possible explanation for this may be a contribution of transporters localized in other cells than lung epithelial cells to the pulmonary disposition of [11C]erlotinib. The radiotracers tested so far undergo rapid pulmonary absorption and therefore do not reflect the physicochemical properties of lung-targeted inhalation drugs. It, therefore, remains to be determined whether transporter effects differ for clinically used inhaled drugs. It may be speculated that transporter effects may be greater for compounds which have lower permeability of the epithelial cell layer. There are also some studies that used [99mTc]Tc-sestamibi (), a myocardial perfusion imaging agent and known substrate of P-gp and MRP1, in an attempt to visualize pulmonary transporter activity with planar gamma scintigraphy in humans [Citation102–104]. Based on the observation that the pulmonary elimination of inhaled aerosolized [99mTc]Tc-sestamibi was slower in humans than that of [99mTc]Tc-DTPA, despite the fact that [99mTc]Tc-sestamibi is more lipophilic than [99mTc]Tc-DTPA, it has been hypothesized that this effect may be due to the action of transporters which retain the radiotracer in the lungs [Citation102]. In comparison to the transporter radiotracers tested with PET imaging in rats described before [Citation98,Citation99], t1/2,elim values of [99mTc]Tc-sestamibi were markedly longer in humans (80–190 min), which points to more ideal properties as a transporter imaging radiotracer. On the other hand, [99mTc]Tc-sestamibi is transported by both P-gp and MRP1 [Citation105], which work in opposite directions in the lung epithelium and cellular uptake of the radiotracer is additionally dependent on the mitochondrial and plasma membrane potential of cells [Citation106]. This may complicate the use of [99mTc]Tc-sestamibi as a transporter imaging radiotracer. No mechanistic studies in transporter knockout rodent models have been performed yet to elucidate to which extent individual transporters affect the pulmonary disposition of [99mTc]Tc-sestamibi.
Taken together, imaging techniques appear to be a promising approach to assess the effects of transporters on pulmonary drug disposition, but this field is still in its infancy and the next necessary step would be to move from radiolabeled prototypical transporter substrates to clinically used inhaled drugs.
7. Conclusions
Knowledge of the lung pharmacokinetics of inhaled drugs is essential to understand and optimize their pharmacological effects. While lung pharmacokinetic data can be generated in a preclinical setting by invasive tissue sampling, data on intrapulmonary pharmacokinetics of inhalation drugs in humans are rarely available. Moreover, the effect of disease on pulmonary disposition of inhaled drugs is incompletely understood. This translational knowledge gap can be potentially bridged by using nuclear medicine imaging techniques (i.e. planar gamma scintigraphy, SPECT and PET), which can dynamically and noninvasively measure the lung tissue concentrations of radiolabeled drugs both in animal models and in humans. There are three approaches how imaging can be used to assess pulmonary drug disposition (): (i) planar gamma scintigraphy with radiolabeled inhalation formulations to assess initial pulmonary drug deposition, (ii) PET imaging studies with radiolabeled drugs to assess their intrapulmonary pharmacokinetics and (iii) receptor occupancy studies to quantify the pharmacodynamic effect of inhaled drugs. Apart from lung deposition studies, imaging techniques have so far been rarely used, but they indeed bear a great potential to make an important contribution to pulmonary drug development.
8. Expert opinion
Imaging techniques currently play an important role in drug development as they provide answers to important questions related to pharmacokinetics and pharmacodynamics. They may either be employed in combination with a radiolabeled drug to assess the distribution of the drug to the tissue targeted for treatment or to tissue relevant for drug toxicity or they may be used to quantify drug effect (target occupancy or downstream effects) [Citation107]. Further than that imaging methods play an important role as a diagnostic tool in precision medicine, e.g. to tailor targeted therapies to subgroups of patients with specific molecular alterations [Citation108]. While these questions are also of high relevance to pulmonary drug development, the application of imaging techniques in this field is still in its infancy. Beyond the mere assessment of initial drug deposition, imaging techniques may potentially provide information on the intrapulmonary pharmacokinetics of inhaled drugs, the effect of transporters on pulmonary drug disposition and the extent of pulmonary target engagement. The underutilization of imaging techniques in pulmonary drug development is most certainly related to several technical challenges associated with imaging the lungs. To image the lung disposition of an inhaled drug, the drug first has to be radiolabeled with a suitable radionuclide, ideally without changing its chemical structure. Considerable advances have been made in PET radiochemistry over the past decades which allows to rapidly introduce short-lived radionuclides (11C, 18F) into many different chemical scaffolds [Citation41–44]. For human applications and depending on often country-specific regulatory requirements, the radiolabeling of drugs has to be performed under good manufacturing practice (GMP)-like conditions, which forms an additional hurdle to conduct PET studies in humans with novel radiotracers. In addition to these radiochemistry-related issues, which apply to the application of imaging studies in all therapeutic fields, a particular challenge related to inhalation drugs comes with the necessity to incorporate the radiolabeled drug into the delivery device [Citation68]. Considering the short radioactive half-lives of PET radionuclides this needs to be rapidly done and be technically feasible within the environment of a radiochemistry laboratory. In addition, some quality control is needed to ascertain that the radiolabeled drug formulation retains the same properties as the unlabeled formulation [Citation68]. This can most commonly only be performed after administration of the radiolabeled drug due to time constraints associated with the short radioactive half-lives. Once successful radiolabeling has been achieved, drug administration has to be tackled. One challenge comes here with the fact that imaging is usually performed in a supine position, while inhalation is usually performed in an upright position. This means that there will be a time delay of a few minutes between drug administration and the start of the scan due to the necessity to position the subject under the scanner, so that the initial phase of drug deposition cannot be measured. This may pose a particular challenge for drugs which undergo rapid pulmonary absorption. In the context of the inhalation of radiolabeled compounds, precautions have to be made to avoid room air contamination by radioactivity and exposure of study personnel to radioactive aerosols. Drug inhalation is often not very efficient and the fraction of drug deposited in the lungs may only be in the range of 10–20% of the drug emitted from the inhalation device. The remainder of the radiolabeled drug may be initially deposited in the oral cavity or larynx and then be swallowed. To quantitatively determine the lung-deposited dose of a radiolabeled drug, the total administered dose needs to be known as well as the amount of drug delivered to the lungs. This is often done by whole-body imaging to quantify total radioactivity in the entire body. Here, however, a limitation comes from the short axial FOVs of many, in particular older-generation PET scanners, so that whole-body imaging cannot be performed simultaneously with lung imaging and rather needs to be performed at the end of the imaging session by consecutively covering the entire body in different segments. In this context, the upcoming availability of total-body PET systems with axial FOVs of up to 194 cm offers the unique opportunity to perform rapid dynamic imaging of the entire body [Citation67]. This should be very helpful to quantify lung-deposited dose and simultaneously assess drug disposition in other organs of the body than the lungs, which may be of particular relevance when oral inhalation is employed as a means to enable systemic drug delivery. Moreover, due to their higher sensitivity as compared with conventional PET scanners, total-body PET systems may allow following the disposition of drugs labeled with short-lived radionuclides such as 11C for an extended period of time (up to 3 hours). An important factor that needs to be considered in studies involving the administration of radiolabeled compounds to humans is radiation dose. Due to short radioactive half-life of 11C, effective doses associated with typically administered 11C-activity amounts are generally rather low (~2 mSv) and justifiable in a medical research context. However, for longer lived PET radionuclides (e.g. 18F, 89Zr) dosimetry is of concern, in particular in the context of the complex disposition pattern after oral inhalation in which part of the radiolabeled drug may be swallowed leading to radiation exposure of the gastrointestinal tract. Further challenges with lung imaging come from the fact that the density of lung tissue is far below 1 (~0.28 g/ml) due to a substantial fraction of air in the lungs (~72%). Pulmonary air content may differ in patient groups as compared with healthy volunteers [Citation109]. Correction for pulmonary air content can be made based on low-dose attenuation correction CT scans [Citation110]. Moreover, a considerable fraction of imaging signal in the lungs comes from the blood pool (~16%), which can be corrected for by kinetic modeling approaches [Citation109]. Further than that, lung tissue contains different sub-compartments and imaging measures the total tissue radioactivity and cannot distinguish intracellular from extracellular drug or protein-bound drug from unbound drug. In addition, radiolabeled parent drug cannot be distinguished from radiolabeled metabolites. Fortunately, the lungs contain a low amount of metabolic enzymes [Citation5], so that inhaled drugs most commonly do not undergo metabolic conversion in lung tissue. This may be different when radiotracers are administered i.v. for imaging the lungs. In this case, radiolabeled metabolites may contribute to the lung imaging signal as access of polar metabolites to lung tissue from the blood is usually not restricted, as is for instance the case for the blood-brain barrier. For the quantitative analysis of radiolabeled drug disposition after systemic (i.v.) administration compartmental modeling is commonly performed [Citation111,Citation112]. This usually requires an arterial input function (i.e. the time course of unmetabolized radiotracer in arterial blood), which is either obtained by sampling or derived from the PET imaging data (e.g. by placing a region of interest into the aorta or the left ventricle of the heart). In case of the occurrence of radiotracer metabolism, discrete blood samples need to be analyzed for radiolabeled metabolites by chromatographic methods (i.e. high-performance liquid chromatography [HPLC] or thin-layer chromatography [TLC]) to derive metabolite correction factors which are then applied to the arterial input function. This is not feasible for radiotracers delivered by inhalation as the input function would be the time course of radiotracer in the airway lumen, which can neither be distinguished from lung tissue in the imaging signal nor can it be independently sampled/measured. This complicates the application of compartmental modeling approaches to assess pulmonary disposition of inhaled drugs. In this context, it may be of interest to measure the ‘output’ function, i.e. the time course of the radiolabeled drug in the pulmonary vein. This information cannot be derived from the PET imaging data as the blood signal will be overlaid with the lung tissue signal. However, blood sampling can be done from the radial artery, which may offer a good approximation of the radiotracer pharmacokinetics in the pulmonary vein [Citation71]. However, arterial kinetics after inhalation may be very rapid (in particular for rapidly absorbed drugs), which will necessitate high temporal resolution blood sampling [Citation71]. Another challenge comes with the occurrence of imaging artifacts related to respiratory motion, which can be potentially mitigated by respiratory gating [Citation113]. For detailed regional assessment of pulmonary drug disposition three-dimensional imaging techniques (PET, SPECT) are clearly preferred over two-dimensional gamma scintigraphy. However, even when employing three-dimensional imaging, a lack of anatomical information may still hamper regional analysis, e.g. to distinguish different airway generations from the peripheral lungs. To overcome this limitation, nuclear medicine imaging data may be co-registered with anatomical imaging data (CT or magnetic resonance imaging [MRI]) [Citation114] which are ideally acquired in the same imaging session in hybrid scanners. For receptor occupancy studies, a challenge comes with the need of a suitable radiotracer to quantify drug binding to the target receptor. Radiotracer development is not a trivial task and is associated with a high failure rate as several different criteria need to be fulfilled for an effective radiotracer (e.g. high selectivity for the target receptor, low nonspecific binding, lack of tissue-penetrant radiolabeled metabolites) [Citation115].
To summarize, several technical challenges are associated with the use of imaging techniques to assess pulmonary drug disposition so that they have so far not been frequently employed in pulmonary drug development. However, considering the wealth of information that can be potentially obtained with imaging studies in this field it is certainly of interest to develop improved and refined imaging protocols and data analysis approaches to further explore this application.
Article highlights
A comprehensive and up-to-date overview of how nuclear medicine imaging techniques can be employed to assess pulmonary drug disposition.
Emphasis is placed on studies in which the inhaled drug molecule itself is radiolabeled and not the formulation, so that in addition to initial deposition, the intrapulmonary pharmacokinetics of the drug can be studied.
The emerging concept of pulmonary receptor occupancy studies is covered.
Using nuclear medicine imaging techniques as a tool to study membrane transporters effects on disposition of inhaled drugs.
Detailed discussion of the technical challenges associated with imaging studies of the lungs.
This box summarizes key points contained in the article.
Declaration of Interest
The authors have no relevant affiliations or financial involvement with any organization or entity with a financial interest in or financial conflict with the subject matter or materials discussed in the manuscript. This includes employment, consultancies, honoraria, stock ownership or options, expert testimony, grants or patents received or pending, or royalties.
Reviewer disclosures
Peer reviewers on this manuscript have no relevant financial or other relationships to disclose.
Additional information
Funding
References
- Cooper AE, Ferguson D, Grime K. Optimisation of DMPK by the inhaled route: challenges and approaches. Curr Drug Metab. 2012;13(4):457–473.
- Anderson S, Atkins P, Bäckman P, et al. Inhaled medicines: past, present, and future. Pharmacol Rev. 2022;74(1): 48–118.
- de Kruijf W, Ehrhardt C. Inhalation delivery of complex drugs-the next steps. Curr Opin Pharmacol. 2017;36:52–57.
- Weibel ER, Gomez DM. Architecture of the human lung. Use of quantitative methods establishes fundamental relations between size and number of lung structures. Science. 1962;137(3530):577–585.
- Patton JS, Byron PR. Inhaling medicines: delivering drugs to the body through the lungs. Nat Rev Drug Discov. 2007;6(1):67–74.
- Bäckman P, Cabal A, Clark A, et al. iBCS: 2. mechanistic modeling of pulmonary availability of inhaled drugs versus critical product attributes. Mol Pharm. 2022;19(7): 2040–2047.
- Forbes B, Asgharian B, Dailey LA, et al. Challenges in inhaled product development and opportunities for open innovation. Adv Drug Deliv Rev. 2011;63(1–2):69–87.
- Scheuch G, Bennett W, Borgstrom L, et al. Deposition, imaging, and clearance: what remains to be done? J Aerosol Med Pulm Drug Deliv. 2010;23(Suppl 2): S39–57.
- Berridge MS, Lee Z, Heald DL. Regional distribution and kinetics of inhaled pharmaceuticals. Curr Pharm Des. 2000;6(16):1631–1651.
- Conway J. Lung imaging - two dimensional gamma scintigraphy, SPECT, CT and PET. Adv Drug Deliv Rev. 2012;64(4):357–368.
- Newman SP, Pitcairn GR, Hirst PH, et al. Radionuclide imaging technologies and their use in evaluating asthma drug deposition in the lungs. Adv Drug Deliv Rev. 2003;55(7):851–867.
- Walker PS, Conway JH, Fleming JS, et al. Pulmonary clearance rate of two chemically different forms of inhaled pertechnetate. J Aerosol Med. 2001;14(2):209–215.
- Corcoran TE, Niven R, Verret W, et al. Lung deposition and pharmacokinetics of nebulized cyclosporine in lung transplant patients. J Aerosol Med Pulm Drug Deliv. 2014;27(3):178–184.
- Sangwan S, Agosti JM, Bauer LA, et al. Aerosolized protein delivery in asthma: gamma camera analysis of regional deposition and perfusion. J Aerosol Med. 2001;14(2):185–195.
- Häussermann S, Winnips C, Edelman J, et al. Lung deposition of alpha1-proteinase inhibitor (human) (A1-PI[H]) inhalation solution using two inhalation modes of the I-neb adaptive aerosol delivery (AAD) system in healthy subjects and subjects with cystic fibrosis. J Aerosol Med Pulm Drug Deliv. 2016;29(3):242–250.
- Köhler D, Fleischer W, Matthys H. New method for easy labeling of beta-2-agonists in the metered dose inhaler with technetium 99m. Respiration. 1988;53(2):65–73.
- Lee Z, Berridge MS, Nelson AD, et al. The effect of scatter and attenuation on aerosol deposition as determined by gamma scintigraphy. J Aerosol Med. 2001;14(2):167–183.
- Depreter F, Burniat A, Blocklet D, et al. Comparative pharmacoscintigraphic and pharmacokinetic evaluation of two new formulations of inhaled insulin in type 1 diabetic patients. Eur J Pharm Biopharm. 2012;80(1):4–13.
- Brand P, Meyer T, Weuthen T, et al. Lung deposition of radiolabeled tiotropium in healthy subjects and patients with chronic obstructive pulmonary disease. J Clin Pharmacol. 2007;47(10):1335–1341.
- Dolovich MB, Bailey DL. Positron emission tomography (PET) for assessing aerosol deposition of orally inhaled drug products. J Aerosol Med Pulm Drug Deliv. 2012;25(1):S52–71.
- Borgström L, Newman S, Weisz A, et al. Pulmonary deposition of inhaled terbutaline: comparison of scanning gamma camera and urinary excretion methods. J Pharm Sci. 1992;81(8):753–755.
- Newman SP, Moren F, Trofast E, et al. Deposition and clinical efficacy of terbutaline sulphate from Turbuhaler, a new multi-dose powder inhaler. Eur Respir J. 1989;2(3):247–252.
- Fuller HD, Dolovich MB, Turpie FH, et al. Efficiency of bronchodilator aerosol delivery to the lungs from the metered dose inhaler in mechanically ventilated patients. A study comparing four different actuator devices. Chest. 1994;105(1):214–218.
- Häussermann S, Acerbi D, Brand P, et al. Lung deposition of formoterol HFA (Atimos/Forair) in healthy volunteers, asthmatic and COPD patients. J Aerosol Med. 2007;20(3):331–341.
- De Backer W, Devolder A, Poli G, et al. Lung deposition of BDP/formoterol HFA pMDI in healthy volunteers, asthmatic, and COPD patients. J Aerosol Med Pulm Drug Deliv. 2010;23(3):137–148.
- Tal A, Golan H, Grauer N, et al. Deposition pattern of radiolabeled salbutamol inhaled from a metered-dose inhaler by means of a spacer with mask in young children with airway obstruction. J Pediatr. 1996;128(4):479–484.
- Verbanck S, Biddiscombe MF, Usmani OS. Inhaled aerosol dose distribution between proximal bronchi and lung periphery. Eur J Pharm Biopharm. 2020;152:18–22.
- Usmani OS, Biddiscombe MF, Barnes PJ. Regional lung deposition and bronchodilator response as a function of beta2-agonist particle size. Am J Respir Crit Care Med. 2005;172(12):1497–1504.
- Warren S, Taylor G, Smith J, et al. Gamma scintigraphic evaluation of a novel budesonide dry powder inhaler using a validated radiolabeling technique. J Aerosol Med. 2002;15(1):15–25.
- Saari SM, Vidgren MT, Turjanmaa VM, et al. No impairment of peripheral deposition in novel asthmatics treated with an MDI corticosteroid with spacer. Respir Med. 2003;97(2):152–158.
- Newman SP, Sutton DJ, Segarra R, et al. Lung deposition of Aclidinium bromide from Genuair, a multidose dry powder inhaler. Respiration. 2009;78(3):322–328.
- Usmani O, Roche N, Wahab E, et al. A scintigraphy study of budesonide/glycopyrrolate/formoterol fumarate metered dose inhaler in patients with moderate-to-very severe chronic obstructive pulmonary disease. Respir Res. 2021;22(1):261.
- Kappeler D, Sommerer K, Kietzig C, et al. Pulmonary deposition of fluticasone propionate/formoterol in healthy volunteers, asthmatics and COPD patients with a novel breath-triggered inhaler. Respir Med. 2018;138:107–114.
- Galindo-Filho VC, Alcoforado L, Rattes C, et al. A mesh nebulizer is more effective than jet nebulizer to nebulize bronchodilators during non-invasive ventilation of subjects with COPD: a randomized controlled trial with radiolabeled aerosols. Respir Med. 2019;153:60–67.
- Brand P, Hederer B, Austen G, et al. Higher lung deposition with Respimat Soft Mist inhaler than HFA-MDI in COPD patients with poor technique. Int J Chron Obstruct Pulmon Dis. 2008;3(4):763–770.
- Weers J, Metzheiser B, Taylor G, et al. A gamma scintigraphy study to investigate lung deposition and clearance of inhaled amikacin-loaded liposomes in healthy male volunteers. J Aerosol Med Pulm Drug Deliv. 2009;22(2):131–138.
- Coates AL, Green M, Leung K, et al. Rapid pulmonary delivery of inhaled tobramycin for Pseudomonas infection in cystic fibrosis: a pilot project. Pediatr Pulmonol. 2008;43(8):753–759.
- Cassidy JP, Amin N, Marino M, et al. Insulin lung deposition and clearance following Technosphere(R) insulin inhalation powder administration. Pharm Res. 2011;28(9):2157–2164.
- Newman S, Salmon A, Nave R, et al. High lung deposition of 99mTc-labeled ciclesonide administered via HFA-MDI to patients with asthma. Respir Med. 2006;100(3):375–384.
- Leach CL, Bethke TD, Boudreau RJ, et al. Two-dimensional and three-dimensional imaging show ciclesonide has high lung deposition and peripheral distribution: a nonrandomized study in healthy volunteers. J Aerosol Med. 2006;19(2):117–126.
- Mason NS, Mathis CA. Positron emission tomography radiochemistry. Neuroimaging Clin N Am. 2003;13(4):671–687.
- Li Z, Conti PS. Radiopharmaceutical chemistry for positron emission tomography. Adv Drug Deliv Rev. 2010;62(11):1031–1051.
- Miller PW, Long NJ, Vilar R, et al. Synthesis of 11C, 18F, 15O, and 13N radiolabels for positron emission tomography. Angew Chem Int Ed Engl. 2008;47(47):8998–9033.
- Jacobson O, Kiesewetter DO, Chen X. Fluorine-18 radiochemistry, labeling strategies and synthetic routes. Bioconjug Chem. 2015;26(1):1–18.
- Wolf W. Imaging can be much more than pretty pictures. Pharm Res. 1995;12(12):1821–1822.
- Fischman AJ, Alpert NM, Rubin RH. Pharmacokinetic imaging: a noninvasive method for determining drug distribution and action. Clin Pharmacokinet. 2002;41(8):581–602.
- Burt T, Young G, Lee W, et al. Phase 0/microdosing approaches: time for mainstream application in drug development? Nat Rev Drug Discov. 2020;19(11):801–818.
- Van der Veldt AAM, Lubberink M, Bahce I, et al. Rapid decrease in delivery of chemotherapy to tumors after anti-VEGF therapy: implications for scheduling of anti-angiogenic drugs. Cancer Cell. 2012;21(1):82–91.
- Sundelin EI, Gormsen LC, Jensen JB, et al. Genetic polymorphisms in organic cation transporter 1 attenuates hepatic metformin exposure in humans. Clin Pharmacol Ther. 2017;102(5):841–848.
- Ordonez AA, Wang H, Magombedze G, et al. Dynamic imaging in patients with tuberculosis reveals heterogeneous drug exposures in pulmonary lesions. Nat Med. 2020;26(4):529–534.
- Jucaite A, Stenkrona P, Cselenyi Z, et al. Brain exposure of the ATM inhibitor AZD1390 in humans-a positron emission tomography study. Neuro Oncol. 2021;23(4):687–696.
- Brown NF, Williams M, Arkenau HT, et al. A study of the focal adhesion kinase inhibitor GSK2256098 in patients with recurrent glioblastoma with evaluation of tumor penetration of [11C]GSK2256098. Neuro Oncol. 2018;20(12):1634–1642.
- Heuveling DA, de Bree R, Vugts DJ, et al. Phase 0 microdosing PET study using the human mini antibody F16SIP in head and neck cancer patients. J Nucl Med. 2013;54(3):397–401.
- Thorneloe KS, Sepp A, Zhang S, et al. The biodistribution and clearance of AlbudAb, a novel biopharmaceutical medicine platform, assessed via PET imaging in humans. EJNMMI Res. 2019;9(1):45.
- Wollmer P, Pride NB, Rhodes CG, et al. Measurement of pulmonary erythromycin concentration in patients with lobar pneumonia by means of positron tomography. Lancet. 1982;2(8312):1361–1364.
- Bajc M, Neilly JB, Miniati M, et al. EANM guidelines for ventilation/perfusion scintigraphy: part 1. Pulmonary imaging with ventilation/perfusion single photon emission tomography. Eur J Nucl Med Mol Imaging. 2009;36(8):1356–1370.
- Bäckström E, Lundqvist A, Boger E, et al. Development of a novel lung slice methodology for profiling of inhaled compounds. J Pharm Sci. 2016;105(2):838–845.
- Luptakova D, Vallianatou T, Nilsson A, et al. Neuropharmacokinetic visualization of regional and subregional unbound antipsychotic drug transport across the blood-brain barrier. Mol Psychiatry. 2021;26(12):7732–7745.
- Berridge MS, Lee Z, Heald DL. Pulmonary distribution and kinetics of inhaled [11C]triamcinolone acetonide. J Nucl Med. 2000;41(10):1603–1611.
- Berridge MS, Cassidy EH, Bordeaux KG. Preparation of [C-11] triamcinolone acetonide. Appl Radiat Isot. 1994;45(1):91–95.
- Lee Z, Heald D, Berridge MS. Comparison of PET and gamma scintigraphy: validation studies. J Aerosol Med. 1999;12:134.
- Berridge MS, Heald DL. In vivo characterization of inhaled pharmaceuticals using quantitative positron emission tomography. J Clin Pharmacol. 1999;39(S1):25S–29S.
- Berridge MS, Heald DL, Muswick GJ, et al. Biodistribution and kinetics of nasal carbon-11-triamcinolone acetonide. J Nucl Med. 1998;39(11):1972–1977.
- Spiro SG, Singh CA, Tolfree SE, et al. Direct labelling of ipratropium bromide aerosol and its deposition pattern in normal subjects and patients with chronic bronchitis. Thorax. 1984;39(6):432–435.
- Virgolini I, Kurtaran A, Leimer M, et al. Inhalation scintigraphy with iodine-123-labeled interferon gamma-1b: pulmonary deposition and dose escalation study in healthy volunteers. J Nucl Med. 1997;38(9):1475–1481.
- Issa F, Kassiou M, Chan HK, et al. Synthesis and radiolabelling of ipratropium and tiotropium for use as PET ligands in the study of inhaled drug deposition. Aust J Chem. 2006;59(1):53–58.
- Badawi RD, Shi H, Hu P, et al. First human imaging studies with the EXPLORER total-body PET scanner. J Nucl Med. 2019;60(3):299–303.
- Constantino M, Waters SL, Steel CJ, et al. FlixotideTM-pressurized metered-dose inhalers loaded with [18F]fluticasone propionate particles for drug deposition studies in humans with PET–formulation and analysis. J Label Compd Radiopharm. 2004;47:55–70.
- Bergström M, Nordberg A, Lunell E, et al. Regional deposition of inhaled 11C-nicotine vapor in the human airway as visualized by positron emission tomography. Clin Pharmacol Ther. 1995;57(3):309–317.
- Lunell E, Bergström M, Antoni G, et al. Nicotine deposition and body distribution from a nicotine inhaler and a cigarette studied with positron emission tomography. Clin Pharmacol Ther. 1996;59(5):593–594.
- Berridge MS, Apana SM, Nagano KK, et al. Smoking produces rapid rise of [11C]nicotine in human brain. Psychopharmacology (Berl). 2010;209(4): 383–394.
- Apana SM, Berridge MS. Cigarettes labeled with [C-11]nicotine: formulation and administration for PET inhalation. J Labelled Compd Rad. 2010;53(1–2):6–10.
- Rose JE, Mukhin AG, Lokitz SJ, et al. Kinetics of brain nicotine accumulation in dependent and nondependent smokers assessed with PET and cigarettes containing 11C-nicotine. Proc Natl Acad Sci U S A. 2010;107(11):5190–5195.
- Solingapuram Sai KK, Zuo Y, Rose JE, et al. Rapid brain nicotine uptake from electronic cigarettes. J Nucl Med. 2020;61(6):928–930.
- Wall A, Roslin S, Borg B, et al. E-cigarette aerosol deposition and disposition of [11C]nicotine using positron emission tomography: a comparison of nicotine uptake in lungs and brain using two different nicotine formulations. Pharmaceuticals. 2022;15(3).
- Elsinga PH, van Waarde A, Vaalburg W. Receptor imaging in the thorax with PET. Eur J Pharmacol. 2004;499(1–2):1–13.
- Wang X, Zhang ZY, Powers D, et al. Rolapitant absolute bioavailability and PET imaging studies in healthy adult volunteers. Clin Pharmacol Ther. 2017;102(2):332–339.
- Raje S, Patat AA, Parks V, et al. A positron emission tomography study to assess binding of lecozotan, a novel 5-hydroxytryptamine-1A silent antagonist, to brain 5-HT1A receptors in healthy young and elderly subjects, and in patients with Alzheimer’s disease. Clin Pharmacol Ther. 2008;83(1):86–96.
- Schou M, Ewing P, Cselenyi Z, et al. Pulmonary PET imaging confirms preferential lung target occupancy of an inhaled bronchodilator. EJNMMI Res. 2019;9(1): 9.
- Maher TM, Simpson JK, Porter JC, et al. A positron emission tomography imaging study to confirm target engagement in the lungs of patients with idiopathic pulmonary fibrosis following a single dose of a novel inhaled alphavbeta6 integrin inhibitor. Respir Res. 2020;21(1):75.
- Hayes MJ, Qing F, Rhodes CG, et al. In vivo quantification of human pulmonary beta-adrenoceptors: effect of beta-agonist therapy. Am J Respir Crit Care Med. 1996;154(5):1277–1283.
- van Waarde A, Maas B, Doze P, et al. Positron emission tomography studies of human airways using an inhaled beta-adrenoceptor antagonist, S-11C-CGP 12388. Chest. 2005;128(4): 3020–3027.
- Cselenyi Z, Jucaite A, Kristensson C, et al. Quantification and reliability of [11C]VC - 002 binding to muscarinic acetylcholine receptors in the human lung - a test-retest PET study in control subjects. EJNMMI Res. 2020;10(1):59.
- Tronde A, Nordén B, Marchner H, et al. Pulmonary absorption rate and bioavailability of drugs in vivo in rats: structure-absorption relationships and physicochemical profiling of inhaled drugs. J Pharm Sci. 2003;92(6):1216–1233.
- Selo MA, Sake JA, Ehrhardt C, et al. Organic cation transporters in the lung-current and emerging (patho)physiological and pharmacological concepts. Int J Mol Sci. 2020;21(23):9168.
- Nickel S, Clerkin CG, Selo MA, et al. Transport mechanisms at the pulmonary mucosa: implications for drug delivery. Expert Opin Drug Deliv. 2016;13(5): 667–690.
- Gumbleton M, Al-Jayyoussi G, Crandon-Lewis A, et al. Spatial expression and functionality of drug transporters in the intact lung: objectives for further research. Adv Drug Deliv Rev. 2011;63(1–2):110–118.
- Sakamoto A, Matsumaru T, Yamamura N, et al. Quantitative expression of human drug transporter proteins in lung tissues: analysis of regional, gender, and interindividual differences by liquid chromatography-tandem mass spectrometry. J Pharm Sci. 2013;102(9):3395–3406.
- Al-Jayyoussi G, Price DF, Kreitmeyr K, et al. Absorption of ipratropium and l-carnitine into the pulmonary circulation of the ex-vivo rat lung is driven by passive processes rather than active uptake by OCT/OCTN transporters. Int J Pharm. 2015;496(2):834–841.
- Al-Jayyoussi G, Price DF, Francombe D, et al. Selectivity in the impact of P-glycoprotein upon pulmonary absorption of airway-dosed substrates: a study in ex vivo lung models using chemical inhibition and genetic knockout. J Pharm Sci. 2013;102(9):3382–3394.
- Zamek-Gliszczynski MJ, Bedwell DW, Bao JQ, et al. Characterization of SAGE Mdr1a (P-gp), Bcrp, and Mrp2 knockout rats using loperamide, paclitaxel, sulfasalazine, and carboxydichlorofluorescein pharmacokinetics. Drug Metab Dispos. 2012;40(9):1825–1833.
- Cossio U, Gomez-Vallejo V, Flores M, et al. Preclinical evaluation of aerosol administration systems using Positron Emission Tomography. Eur J Pharm Biopharm. 2018;130:59–65.
- Bivas-Benita M, Zwier R, Junginger HE, et al. Non-invasive pulmonary aerosol delivery in mice by the endotracheal route. Eur J Pharm Biopharm. 2005;61(3):214–218.
- Meyerholz DK, Suarez CJ, Dintzis SM, et al. Respiratory System. In: Treuting PM, Dintzis SM, Montine KS, editors. Comparative anatomy and histology (Second edition). A mouse, rat, and human atlas. Academic Press; 2017. p. 147–162.
- Okamura T, Kikuchi T, Okada M, et al. Noninvasive and quantitative assessment of the function of multidrug resistance-associated protein 1 in the living brain. J Cereb Blood Flow Metab. 2009;29(3):504–511.
- Okamura T, Kikuchi T, Okada M, et al. Imaging of activity of multidrug resistance-associated protein 1 in the lungs. Am J Respir Cell Mol Biol. 2013;49(3):335–340.
- Zoufal V, Mairinger S, Krohn M, et al. Influence of multidrug resistance-associated proteins on the excretion of the ABCC1 imaging probe 6-bromo-7-[11C]methylpurine in mice. Mol Imaging Biol. 2019;21(2):306–316.
- Mairinger S, Sake JA, Hernández Lozano I, et al. Assessing the activity of multidrug resistance-associated protein 1 at the lung epithelial barrier. J Nucl Med. 2020;61(11): 1650–1657.
- Hernández-Lozano I, Mairinger S, Filip T, et al. PET imaging to assess the impact of P-glycoprotein on pulmonary drug delivery in rats. J Control Release. 2022;342:44–52.
- Wanek T, Mairinger S, Langer O. Radioligands targeting P-glycoprotein and other drug efflux proteins at the blood–brain barrier. J Labelled Comp Radiopharm. 2013;56(3–4):68–77.
- Mairinger S, Hernandez-Lozano I, Filip T, et al. Impact of P-gp and BCRP on pulmonary drug disposition assessed by PET imaging in rats. J Control Release. 2022;349:109–117.
- Ruparelia P, Cheow HK, Evans JW, et al. Pulmonary elimination rate of inhaled 99mTc-sestamibi radioaerosol is delayed in healthy cigarette smokers. Br J Clin Pharmacol. 2008;65(4):611–614.
- Mohan HK, Routledge T, Cane P, et al. Does the clearance of inhaled 99mTc-sestamibi correlate with multidrug resistance protein 1 expression in the human lung? Radiology. 2016;280(3):924–930.
- Mohan HK, Livieratos L, Peters AM. Lung clearance of inhaled aerosol of Tc-99m-methoxyisobutyl isonitrile: relationships with cigarette smoking, age and gender. Clin Physiol Funct I. 2019;39(4):236–239
- Hendrikse NH, Franssen EJ, van der Graaf WT, et al. 99mTc-sestamibi is a substrate for P-glycoprotein and the multidrug resistance-associated protein. Br J Cancer. 1998;77(3):353–358.
- Chiu ML, Kronauge JF, Piwnica-Worms D. Effect of mitochondrial and plasma membrane potentials on accumulation of hexakis (2-methoxyisobutylisonitrile) technetium(I) in cultured mouse fibroblasts. J Nucl Med. 1990;31(10):1646–1653.
- Burt T, Roffel AF, Langer O, et al. Strategic, feasibility, economic, and cultural aspects of phase 0 approaches: is it time to change the drug development process in order to increase productivity? Clin Transl Sci. 2022;15(6):1355–1379.
- Moek KL, Giesen D, Kok IC, et al. Theranostics using antibodies and antibody-related therapeutics. J Nucl Med. 2017;58(Suppl 2):83S–90S.
- Chen DL, Cheriyan J, Chilvers ER, et al. Quantification of lung PET images: challenges and opportunities. J Nucl Med. 2017;58(2):201–207.
- Lukey PT, Coello C, Gunn R, et al. Clinical quantification of the integrin alphavbeta6 by [18F]FB-A20FMDV2 positron emission tomography in healthy and fibrotic human lung (PETAL Study). Eur J Nucl Med Mol Imaging. 2020;47(4):967–979.
- Innis RB, Cunningham VJ, Delforge J, et al. Consensus nomenclature for in vivo imaging of reversibly binding radioligands. J Cereb Blood Flow Metab. 2007;27(9):1533–1539.
- Gunn RN, Gunn SR, Cunningham VJ. Positron emission tomography compartmental models [Review]. J Cereb Blood Flow Metab. 2001;21(6):635–652.
- Grootjans W, Rietbergen DD, van Velden FH. Added value of respiratory gating in positron emission tomography for the clinical management of lung cancer patients. Semin Nucl Med. 2022 May; 25 S0001-2998(22)00037-X. 10.1053/j.semnuclmed.2022.04.006. Online ahead of print.
- Lee Z, Berridge MS. PET imaging-based evaluation of aerosol drugs and their delivery devices: nasal and pulmonary studies. IEEE Trans Med Imaging. 2002;21(10):1324–1331.
- Pike VW. PET radiotracers: crossing the blood-brain barrier and surviving metabolism. Trends Pharmacol Sci. 2009;30(8):431–440.