ABSTRACT
Introduction
Brain diseases including brain tumor, Alzheimer’s disease, Parkinson’s disease, etc. are difficult to treat. The blood–brain barrier (BBB) is a major obstacle for drug delivery into the brain. Although nano-package and receptor-mediated delivery of nanomedicine markedly increases BBB penetration, it yet did not extensively improve clinical cure rate. Recently, brain extracellular space (ECS) and interstitial fluid (ISF) drainage in ECS have been found to determine whether a drug dissolved in ISF can reach its target cells. Notably, an increase in tortuosity of ECS associated with slower ISF drainage induced by the accumulated harmful substances, such as: amyloid-beta (Aβ), α-synuclein, and metabolic wastes, causes drug delivery failure.
Areas covered
The methods of nano-package and receptor-mediated drug delivery and the penetration efficacy of nanomedicines across BBB and ECS are assessed.
Expert opinion
Invasive delivering drug via ECS and noninvasive near-infrared photo-sensitive nanomedicines may provide a promising benefit to patients with brain disease.
1. Introduction
The blood–brain barrier (BBB) is a physical barrier between the blood system and the brain, which was first discovered by Ehrlich in 1885. BBB contains kinds of cells, including brain capillary endothelial cells, pericytes, astrocytes, and neurons. It can isolate the brain tissues from the external environment, restricts the entry of harmful exogenous factors, but hinders the drugs delivery to the brain at the same time [Citation1]. The specific characteristics of a compound govern the method by which it is transported across the BBB, or whether or not it is transported at all. Compounds that have the surface hydrogen bonds (hydrophilic compounds) are only permissive through tight junctions of the BBB, which ultimately serves to prevent the passage of molecules between cells of the endothelium. These hydrophilic compounds are impermeable through the lipophilic endothelium and require lipid-mediated transport in order to permeate transcellularly. Large molecules, such as insulin and transferrin, require receptors, whereas small molecules require the carrier-mediated transport to move across this barrier. There is also active efflux transport for endogenous BBB transporters [Citation2].
Some serious difficulties are faced in drug development of major brain disease, such as: stroke, brain tumors, Parkinson’s disease (PD), Alzheimer’s disease (AD), multiple sclerosis. Only five drugs have been approved by Food and Drug Administration (FDA) of USA with limited symptomatic treatment properties through alleviating cognitive impairments in AD patients as far. Although there is more than $600 billion invested in new drugs to treat AD, it did not achieve desired clinical efficacy. Approximate of 100 drugs related to dementia are focus currently in clinical research or being reviewed by FDA. These anti-Parkinson’s drugs are mainly used for symptomatic treatment and failed to slow down disease progression with varying degrees of side effects. Regardless of the fact that global companies invest billions of dollars in PD’s research each year, the results of clinical trials are unsatisfactory. Overall, these monumental failures in drug development against encephalopathy force us to re-consider whether the drug actually penetrates into BBB and/or whether it reaches the targeted neurons in the damaged brains.
Recently, brain extracellular space (ECS) and interstitial fluid (ISF) have gradually come into sight. ECS, which is a narrow space surrounds every cell of the central nervous system, occupies approximately 20% of the brain volume [Citation3]. And it is composed of interstitial fluid (ISF) and extracellular matrix (ECM). The width of ECS between cells is about tens of nanometers (38 ~ 64 nm). The narrow ECS prevent exogenous drugs from entering deep neurons, especially macromolecular drugs.
ISF includes water, ion and organic molecules, such as proteins, peptides, enzymes, providing the immediate medium for nutrients supply, waste removal, and intercellular communication [Citation4–6]. The lasted studies have shown that ISF does not distribute or flow in the whole brain but is restricted to certain divisions or territories [Citation7]. On the one hand, it means that most drugs may not reach certain areas of the brain, leading to poor drug efficacy; On the other hand, this discovery also brings new perspective for drug design and improvement of drug delivery.
2. BBB and drug delivery
Over 100 years, the effect of conventional oral or intravenous drugs in the treatment of brain diseases has been unsatisfactory. These drugs have high molecular weight, which not only makes it difficult to break through the BBB, even does immense harm to normal cells. With the rapid progress in nanomaterials in recent 30 years. Nano-drug-loaded particles have gradually realized the characteristics of small particle size, high biocompatibility, and low biotoxicity, which make it possible to treat major brain diseases in order to penetrate through BBB. These new nanomedicines have some advantages than the traditional medicines. First, according to the formula of Noyes-Whitney, there is a positive correlation between drug’s surface area and its solubility. The drug-loaded nanoparticles (NPs) are able to encapsulate the drug which is difficult to dissolve, thus facilitating drug delivery and the uptake of drug in human [Citation8]. Second, there are various materials available for preparing nanomedicines. The combination of different materials makes it possible for the functionalization of drug-loaded nanoparticles. In particular, the drug-loaded NPs, which are easy to couple with the ligands of receptors on cerebral vascular endothelial cells, provide an effective way for brain targeted drug delivery and treatment of brain diseases.
Drug delivery across the BBB is one of the difficulties in the treatment of central nervous system diseases. With the rapid development of nanotechnology, different laboratories around the world have developed a variety of nanomaterials and drug delivery systems with multiple targeted mediators. It is considered to be a breakthrough to cross the BBB. This paper highlights the latest progress of nano-packed methods and receptor-mediated delivery strategies of nanomedicines. The former includes liposomes, poly (lactic-co-glycolic acid) (PLGA), quantum dots, silica and other nanoparticles. The latter comprises transferrin receptor (TfR)-mediated, lipoprotein receptor-related protein (LRP)-mediated, low-density lipoprotein receptor (LDLR)-mediated, insulin receptor (INR)-mediated, insulin-like growth factors receptor (IGFR)-mediated, glucose transporter (GLUT)-mediated drug delivery. It also highlights the latest progress of nanomedicines in the treatment of brain diseases ().
Figure 1. Model diagram of nanomedicines- penetrated across the blood brain barrier for treating brain diseases. BBB: blood-brain barrier; IGFR: insulin-like growth factors receptor; INR: insulin receptor; LRP: lipoprotein receptor-related protein; MSN: mesoporous silica nano-particles; NPs: nanoparticles; PLGA: lactic-co-glycolic acid; QDs: quantum dots.
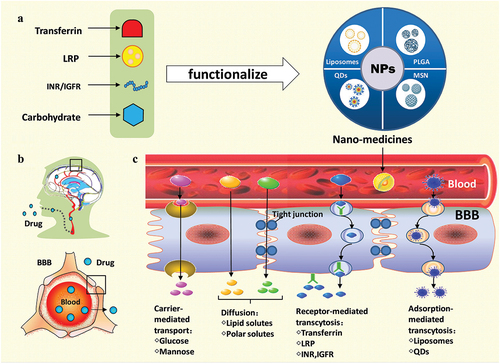
3. Packaging methods of nanomedicines
3.1. Liposome
The concept of liposome was first proposed by Bangham in 1965 [Citation9]. Due to its simple composition and preparation, liposomes are one of the earliest drug-loaded nanoparticles developed by the researchers all over the world. Lipid or modified lipid acts as the basic structure of liposome. The hydrophilic head of the lipids in liposome form the solid-liquid interface, and the hydrophobic tail of the lipids that make up the lipid bilayer are facing in the opposite direction. Under the confocal laser scanning microscopy, the liposome particles are microspheres, and the outer surface is smooth, in which small molecule drugs can be encapsulated. The lipid bilayer of liposome and the basic structure of biological membrane are similar. This similarity indicates that liposomes have excellent biocompatibility, which makes it easy to be ingested by tissue cells, and can be used as a drug delivery platform across BBB. Special pharmacokinetic effects of drug-loaded liposomes can be gained by changing the content and proportion of lipids, drugs and other components in the design of liposomes. In addition, the phospholipid-component of liposome makes it easier to couple with specific molecules, thus exerting its targeted transport function to the brain. Therefore, liposomes are widely synthesized and used in clinical practice. For example, solid lipid NPs loaded with docetaxel (DTX) [Citation10] and the liposomes composed of anti-CD20 functionalized superparamagnetic ferric oxide [Citation11] are prepared for the treatment of brain diseases. shows liposome NPs or their modified counterpart loaded different drugs and their characterizations and applications [Citation12–17]. For brain drug delivery purposes, NPs have to be biocompatible and biodegradable. It is worth noting that compared with NPs that do not degrade or are potentially toxic such as gold and other heavy metal NPs or carbon quantum dots, liposomes show excellent characteristics, such as: high bioavailability, high drug-target binding affinity, low damage and hypersensitivity of normal tissues, sufficient drug-loading capacity and low cytotoxicity [Citation18]. Hence, liposomes are the most widely used and earliest marketed nanomaterials, and liposomes may be one of the most suitable packaging materials for drug delivery to the brain.
Table 1. Liposome NPs-based drug delivery practice.
3.2. Poly (lactic-co-glycolic acid)
Nano-polymer materials have been widely studied since they were applied in the medicinal field. Poly(lactic-co-glycolic acid) (PLGA) occupies a place in many nanomaterials due to its good compatibility and biodegradability, thus becoming the first batch of biodegradable materials approved by the FDA for clinical use [Citation19]. Obtained by co-polymerization of lactic acid and glycolic acid in a defined ratio, PLGA can be metabolized by the human. The intermediate product of PLGA, lactic acid, is one of the normal metabolites in the body, and the final degradation products are H2O and CO2. PLGA is thus widely used in the preparation of microspheres, nanoparticles, implants and film agents [Citation20]. In the field of medicine, PLGA NPs is widely used as drug delivery carriers, which can carry protein [Citation21], polypeptide [Citation22], microbial DNA [Citation23], bacterial or viral DNA [Citation24], various anticancer drugs [Citation25], for example, embedded verteporfin (VP) into PLGA NPs for treating brain tumors [Citation26]. In order to improve the BBB penetration of PLGA NPs, surfactant or polymers are usually used to modify the surface of PLGA NPs, or PLGA NPs is covalent conjugated with the targeting ligands. shows drugs that are carried by PLGA NPs or their modified counterpart loaded different drugs and their characterizations and applications [Citation27–31].
Table 2. PLGA NPs based drug delivery practice.
3.3. Quantum dot
Quantumdot (QDs), known as nanocrystals, are a new type of semiconductor nanomaterials, with a usual size of less than 100 nanometers [Citation32], were first synthesized in 1981 [Citation33]. It was widely used in optical and solar cells and other devices at the end of 20th century [Citation34]. Compared with liposomes, PLGA and other organic materials, quantum dots have many special characteristics, including large absorption spectrum, high photo-bleaching and small size. Therefore, QDs have enormous potential in biomedical imaging and nano-therapy. QDs have made a breakthrough achievement in the fields of sensors, biomedical imaging and nano-targeted drug delivery in recent years due to their excellent optical properties, emission spectrum and good fluorescence characteristics [Citation34–36]. They have excellent biocompatibility and low tissue toxicity. Studies have shown that the specific active groups binding to the surface of QDs can achieve targeted delivery to the brain [Citation37]. Carbon quantum dots (C-QDs) can reduce the deposition of Aβ42 [Citation38], suggesting the potential effect of QDs nanoparticles in AD treatment [Citation39]. It is worth noting that the use of QDs may cause the accumulation of immune cells, resulting in inflammatory reaction [Citation40]. However, the cytotoxicity of QDs has been controversial. QDs may lead to excessive production of reactive oxygen species (ROS) which ultimately induces mitochondrial dysfunction [Citation41]. Recent animal experiments also showed that QDs can be accumulated in the brains in their main exposure routes and causes nerve inflammation, thereby posing a potential threat to brain [Citation42]. A study indicated that carbon quantum dots (CDs) have excellent biocompatibility and low toxicity due to the lack of metal elements [Citation43]. It seems that QDs have enormous potential in biomedical imaging and nano-therapy. Considering the biological characteristics and importance of brain, carbon quantum dots may be a more suitable material.
3.4. Mesoporous silica nanoparticles
A type of Si/Si-Al mesoporous material M41S was first reported in 1992 [Citation44], drawing people’s attention to mesoporous materials [Citation45]. With the rapid development of nanotechnology over 30 years, the research on mesoporous silica nano-particles (MSNs) has become a trend in materials science [Citation46]. MSNs have multiple advantages in targeted drug delivery, due to its strong carrying capacity for low water-solubility drugs. It is worth noting that MSNs, as an inorganic material, is not only easier to modify the surface of special chemical groups, but also easier to form a conjugated system with other inorganic materials and achieving the functional effect. It has been shown that MSN can travel across BBB by preparing it coupled with lactoferrin and modified with polyethylene glycol (PEG) [Citation47]. MSNs have good biocompatibility, and various cell lines are insensitive to oxidative stress caused by MSNs. However, high doses of MSNs may produce cytotoxicity [Citation48]. It is not a long time since MSNs first reported. MSNs show their potential in traveling across BBB, but more research is needed to confirm its effect.
4. New delivery strategies of nanomedicines
4.1. TfR-mediated drug delivery
Transferrin (Tf), a type of glycoprotein, is mostly produced by the liver. Tissue cells can uptake iron by Tf. Firstly, Tf is bound to the extracellular domain of tranferrin receptor (TfR). In this process, a molecule of Tf binds to a subunit of the receptor to form a complex of receptor-Tf-iron. Secondly, this complex is internalized through clathrin-mediated endocytosis into endosome. Then, the ATP-dependent proton pumps (H+ pump) decrease the endosomal pH. This results in the binding force between transferrin and iron to weaken and releasing of iron from transferring [Citation49]. TfR is widely expressed in various cells in the human body; especially, the highest distribution of it is in the liver and brain. TfR is highly expressed in the vascular endothelial cells that constitute BBB, suggesting that TFR can be used to mediate the targeted nanomedicines delivery to the brain [Citation50]. The first nano-silica particles of TfR were prepared in 1987 [Citation51]. TfR has become one of the main solutions to mediate the targeted nanomedicines delivery, particularly in the treatment of brain diseases in recent years. TfR-targeting peptides have recently gained popularity, and about 38% of existing studies used this receptor to achieve targeted drug delivery [Citation52]. Different kinds of TfR-mediated drugs have been researched and developed. For example, Tf-coupled solid lipid nanoparticles of curcumin [Citation53], doxorubicin [Citation54], monoclonal antibody (OX26), or peptides [Citation55,Citation56].
Recently, a series of studies in vivo have indicated that TFR receptor-mediated drug delivery occurs mainly in the post-capillary venules, only little in the capillaries [Citation57]. The penetration of drugs is affected by many factors. First, the penetration is correlated with the density of Tf or antibodies coupled on their surface [Citation58,Citation59]. Another factor is the affinity of antibody. Specifically, antibodies with low affinities mediate a low uptake of intravenously (IV)-injected gold nanoparticles (AuNPs) into the brain, whereas a monovalent (bi-specific) antibody improves the uptake capacity remarkably [Citation58]. Generally speaking, TfR-mediated drug delivery is a promising strategy, and it is necessary to further explore the optimization strategy and in vitro and in vivo evaluation of it, and actively conduct clinical trials, especially in the treatment of gliomas and neurodegenerative diseases.
4.2. LDLR-mediated drug delivery
The low-density lipoprotein receptor (LDLR) superfamily contains low-density lipoprotein receptor-related protein 1 (LRP1), LRP1B, LRP3, LRP4, LRP5, and LRP6 etc [Citation60]. LRP1 is a transmembrane endocytic receptor that mediates the endocytosis of exogenous substances, and consisted of three regions: extracellular region, transmembrane region and intracellular region. It is broadly expressed in human body cells and high levels of distribution in the brain and lungs [Citation61]. This highly expression of LRP1 in BBB suggests that LDL receptor-mediated internalization is a viable route for targeted drug delivery in the brain [Citation62].
Currently, two classes of molecules used for nanoparticle functionalization are Angiopep-2 (Ang, molecular weight 2.4 kDa) and lactoferrin (Lf). Angiopep-2 is 19 amino acids oligopeptide in length that binds to LRP1 with high affinity. These monoclonal antibody-Angiopep-2 complexes can penetrate into BBB for treating intracranial tumors [Citation63,Citation64]. Recently, Angiopep-2 modified lipid nanocomposites show a positive effect of inhibiting Tau phosphorylation in AD mice [Citation65].
Lactoferrin (Lf) is a glycoprotein being owned by the transferrin (Tf) family. LRP participates in the transmembrane transport of Lf, and this targeted transport is a one-way transport from vascular endothelial cells to brain nerve cells and keratinocytes. Thus, the transport efficiency of Lf is greater than that of bidirectional transport of Tf [Citation66]. Recently, Lf dual-modified liposomes loaded with docetaxel (DTX) has been found to enhance targeted delivery in the brain for treating glioma and extend lifespan in mice [Citation67]. Another nanostructured lipid carrier can load with riluzole and functionalize them with Lf [Citation68]. It is noteworthy that a large amount of cholesterol in tumor cells is absorbed to synthesize the cell membrane to satisfy demand of rapid proliferation, thus LDLR is often overexpressed in brain tumor cells. Many studies have proved that nanoparticles can target glioma cells through LDLR-mediated endocytosis [Citation69]. Therefore, LDLR is a potential target for the treatment of brain tumors. However, LDLR still has some disadvantages that need to be paid more attention to. For example, the occurrence of neurodegenerative diseases such as Alzheimer and Parkinson may be accompanied by the down-regulation of LDLR, which is not conducive to LDLR-mediated nanoparticles passing through the BBB [Citation70]. In addition, the expression level of LDLR in the human body (such as liver) in other organs is fluctuating, which may affect the delivery efficiency of LDLR-mediated drug. The curative effect of LDLR-mediated drug remains to be investigated.
4.3. INR or IGFR-mediated drug delivery
Insulin receptor (INR), a member of the receptor protein tyrosine kinase family, is a dimer that is linked by disulfide bonds [Citation71]. Insulin (INS) is the natural ligand of INR in the human body. The insulin-like growth factors receptor (IGFR) is structurally homologous to INR and its ligand is insulin-like growth factors (IGF) which can also bind to INS [Citation72]. INS is essential for memory functions and hippocampal volume correlate with glucose tolerance [Citation73]. The INR/IGFR is highly expressed in BBB; this may provide the possibility for targeted nanoparticles delivery across the BBB. For example, injection of an insulin-targeted gold nanoparticles (INS-GNPs) can improve five-fold levels of INS-GNP in the brains than the controlled non-targeted GNP [Citation74].
It is worth mentioning that in 2006, humanization of anti-human insulin receptor antibody (HIRMAb) was synthesized for the first time. After humanization of HIRMAb radiolabeled with 125-iodine injected into rhesus monkeys, the humanized HIRMAb were rapidly transported into the brain [Citation75]. Since then, the usage of humanized HIRMAb modified nanoparticles via BBB has become popular. It has been reported that IR antibody-iduronidase fusion protein can improve the cognitive impairment and somatic function of patients with mucopolysaccharidosis through the BBB [Citation76]. However, insulin-targeted nanoparticles used in certain IR-sensitive diseases (e.g. AD) may lead to insulin resistance, disturbances in human insulin metabolism or affecting the efficacy of drugs [Citation77]. This kind of drugs are effective in clinical treatment, but they may lead to hypoglycemia and insulin resistance, etc. Compared with other low-risk delivery strategies, this disadvantage will limit the related clinical application in the future.
4.4. GLUT -mediated drug delivery
Glycans play a major role in the recognition of multiple receptor proteins with enzymes, hormones, and antibodies in the human body. Most of these reactions occur through glycoproteins and polysaccharides on the cell surface, so it is believed that glycans can be utilized to mediate targeted drug delivery. Lectin is the most commonly used to as a mediation in the glycan ligand-mediated drug delivery systems [Citation78,Citation79]. Glucose transporter (GLUT) is a group of gating proteins that regulates glucose and other sugars diffusion from the extracellular space into the cell. GLUT1 is highly expressed on the surface of brain capillary endothelial cells (BCECs), and transports sugar molecules, such as: glucose and mannose. This provides a new pathway for drugs delivery into the brain [Citation80].
Notably, some studies have confirmed that only modifying the nanoparticles with glucose does not increase the penetration efficiency of BBB. Controlling the ligand density on the nanoparticles and glycemic concentration can be used to improve the uptake of GLUT-based nanoparticles by brain capillary endothelial cells. Exploiting the rapid glycemic increase after fasting, polymeric micelles decorated with con-figured glucose molecules can penetrate the BBB selectively and efficiently [Citation81]. GLUT1 can migrate from the luminal to the abluminal plasma membrane. This intracellular circulation enables nanomedicines to be remarkably transported by capillary endothelial cell. Glucose and other ligands can be dually modified on the surface of nanoparticles. For example, liposomes dually modified with aminophenyl glucose and cyclic pentapeptide could be used as a therapy for glioblastoma treatment [Citation82]. shows the delivery strategies and beneficial effects of different kinds of NPs in the models of in vitro and in vivo [Citation67,Citation82–95].
Table 3. Delivery strategies nd beneficial effects of different kinds of NPs in the models of in vitro and in vivo.
5. Brain extracellular space and drug delivery
5.1. Unevenness distribution of drug in the brains
Brain tissues have a complex structure for different anisotropic mediators [Citation96]. The distribution of drugs in diverse areas of the brains is different. For example, the cortical area is small, and the diffusion of the drugs is limited; the diffusion along the caudate area is relatively uniform and unevenly; the lateral parenchyma area is spreading rapidly; and the diffusion of the lateral parenchyma area in the lateral ventricle is faster in the upper and lower direction. Recent study indicates that the unevenness distribution of drugs in the brain is closely correlated with the density and structural integrity of the myelin sheath [Citation97] (). Because the flow direction of drug dissolved in the interstitial fluid (ISF) is controlled by the myelin [Citation98]. In addition, the distribution of myelin sheath has been found to be relatively consistent with the flow direction of blood or capillary vessel [Citation99,Citation100]. This suggests that the dynamics of vascular pulsation may drive ISF flow, or may be one of the forces of ISF drainage [Citation101].
Figure 2. Different ISF drainage routes in normal rats and demyelinated rats. Briefly, in healthy rats, the obstruction of the myelinated fiber tracts is compact, and ISF from the caudate nucleus (Cn) could not drain to the ECS of the thalamus (Tha) and vice versa. However, in the demyelinated rats, myelinated fiber tracts are loose, which makes ISF from the caudate nucleus drain to the thalamus and a small quantity of ISF from the thalamus drain to the caudate nucleus [Citation97].
![Figure 2. Different ISF drainage routes in normal rats and demyelinated rats. Briefly, in healthy rats, the obstruction of the myelinated fiber tracts is compact, and ISF from the caudate nucleus (Cn) could not drain to the ECS of the thalamus (Tha) and vice versa. However, in the demyelinated rats, myelinated fiber tracts are loose, which makes ISF from the caudate nucleus drain to the thalamus and a small quantity of ISF from the thalamus drain to the caudate nucleus [Citation97].](/cms/asset/17749842-8ed7-43b0-a952-d0f2b163556e/iedd_a_2139369_f0002_oc.jpg)
Unsurprisingly, the results of the positron emission tomography imaging (PET) and the fluorescent imaging of the small animals have shown that 11C-Befloxatone, a lipid soluble drug [Citation102], 11C-Verapamil, a water-soluble medicine [Citation103], 125I- HIRMAb-IDUA, a receptor-mediated drug [Citation104], and nano-packaged siRNA [Citation105], are inhomogeneous distributed in the brains or other organs (). Until now, there is no argument that no one drug has been found to be evenly distributed in the brains [Citation103]. For example, a kind of carbon-based NPs imaged by near-infrared nanoscopy can exhibit the structure of brain extracellular space (ECS) [Citation106]; meanwhile, it also indicates that if these NPs are looked as drugs, they are not evenly distributed. These data strongly suggest that some more subtle brain structures, such as: ECS, may determine the different distribution of drugs in the different brain regions, besides the factors of water or lipid solubility, or receptor (or ligand)-binding drugs.
Figure 3. Unevenness distribution of drug in the brains of 11C-befloxatone. (a), 11C-verapamil (b), and 125I-HIRMAb-IDUA (c) imaged by the positron emission tomography and nano-packaged siRNA (d) scanned by the fluorescent imaging of the small animals, respectively. Figures were adapted from reference [Citation102–105].
![Figure 3. Unevenness distribution of drug in the brains of 11C-befloxatone. (a), 11C-verapamil (b), and 125I-HIRMAb-IDUA (c) imaged by the positron emission tomography and nano-packaged siRNA (d) scanned by the fluorescent imaging of the small animals, respectively. Figures were adapted from reference [Citation102–105].](/cms/asset/7971b3d8-5381-4337-9c14-ba6e7f1998e4/iedd_a_2139369_f0003_oc.jpg)
5.2. Brain ECS and ISF control drug delivery
5.2.1. Brain ECS structures and ISF drainage
Brain ECS is the narrow microenvironment that surrounds every cell of the central nervous system. It contains a solution that closely resembles interstitial fluid (ISF) with the addition of extracellular matrix (ECM) molecules [Citation107,Citation108]. The space serves as a reservoir for ions essential to the electrical activity of neurons and forms an intercellular chemical communication channel. Brain ECS remains largely unknown territory that is ripe for exploration with new technologies [Citation109–111]. The narrow space between the cells of the central nervous system has been a topic of fascination and frustration to a small number of investigators with different methods, such as: real-time iontophoresis combined with ion-selective electrode detection, microfiber imaging, electron microscopy, magnetic resonance imaging (MRI), intrinsic optical signal imaging, integrative optical imaging, two-photon and confocal microscopy, time-resolved fluorescence anisotropy imaging, super-resolution shadow imaging, a complementary super-resolution microscopy with near-infrared fluorescent nanometer-sized single-walled carbon nanotubes [Citation112].
Having a width estimated in the tens of nanometers, about 38 ~ 64 nm, the space is below the resolution of light microscopy yet they are obliterated by most conventional electron microscopic procedures. Using specialized fixation techniques, electrophysiological methods, and theoretical models, a picture has emerged that shows the narrow intercellular channels to be a complex microenvironment essential to neuronal function, a signaling pathway in its own right, and an important conduit for drug delivery [Citation3]. The diffusion studies both experimental and theoretical tell us how a particular molecule is likely to distribute in the brain. This is useful for exploring some types of signaling between cells and for the important practical application of drug delivery [Citation113].
Most of the research in brain science has been focused on neural networks and vascular systems, which account for 80–85% of the total brain mass. However, the remaining brain ECS systems (15 ~ 20%) have not received much attention over the past 100 years ()). Notably, roles of ‘ECS’ should not be neglected, because it may play a crucial role in brain structure and function, especially in drug delivery. Actually, the nano-scale structures of these ECS, which exist in the narrow gaps between inter-connected and irregular interstitial tissues, are an important link between neurons and glial cells, and also an essential functional unit for maintaining homeostasis of the brain cell living environment [Citation114]. In particular, the ISF in ECS contains neurotransmitters, hormones, nutrient substances, and metabolic wastes; ISF drainage has been proven to affect sleep and cognition [Citation7,Citation109,Citation115]. However, there have been few reports on the roles of ISF drainage in the nano-scale ECS of brains for drug delivery so far.
Figure 4. Brain extracellular space and drug delivery. a. Brain ECS and ISF in the cortex and other cerebral parenchyma. BBB: blood-brain barrier; PA: Pial artery; PPS: Pial perivascular space. b, c. Drugs penetrate out of the capillaries and enter brain ECS. d. Brain ECS systems account for 15–20% of the total brain mass. ECS: extracellular space; ISF: interstitial fluid; N: neuronal cells; V: vessel. e-h. Brain ECS division system in the brains detected by MRI with Gd-DTPA probe. Am: amygdala; CPu: caudate putamen; Mb: midbrain; MRI: magnetic resonance imaging; mPC: medial prefrontal cortex; OB: olfactory bulb; OC: occipital cortex; SC: superior colliculus; T: thalamus. Figure was adapted from reference [Citation97].
![Figure 4. Brain extracellular space and drug delivery. a. Brain ECS and ISF in the cortex and other cerebral parenchyma. BBB: blood-brain barrier; PA: Pial artery; PPS: Pial perivascular space. b, c. Drugs penetrate out of the capillaries and enter brain ECS. d. Brain ECS systems account for 15–20% of the total brain mass. ECS: extracellular space; ISF: interstitial fluid; N: neuronal cells; V: vessel. e-h. Brain ECS division system in the brains detected by MRI with Gd-DTPA probe. Am: amygdala; CPu: caudate putamen; Mb: midbrain; MRI: magnetic resonance imaging; mPC: medial prefrontal cortex; OB: olfactory bulb; OC: occipital cortex; SC: superior colliculus; T: thalamus. Figure was adapted from reference [Citation97].](/cms/asset/489bad7f-5992-45b5-b47c-4c20d051f729/iedd_a_2139369_f0004_oc.jpg)
5.2.2. ISF drainage drives drug to reach the targeted neurons
Drugs that are developed for brain diseases, such as: stroke, AD, PD, brain tumors, etc., firstly need to penetrate the BBB. Of particular note is the case that the drug infiltrated from the capillaries does not directly reach the neurons [Citation3]. This does mean that drugs must pass through the 38 ~ 64-nm diameter ECS to approach the targeted neurons in the deep layer of the cortex along the direction of ISF drainage. Generally, it has been reported that 95% of the developed synthetic drugs, antibodies, and synthetic polypeptides did not pass through the BBB [Citation116,Citation117], and that drugs of diameter greater than 60 nm did not enter the ECS or arrive at the target neurons [Citation118] (), bottom). The vascular pulsation may be one of the forces of ISF drainage [Citation101]. This may be a possible reasonable explanation that the limitations associated with both the BBB and ECS directly or indirectly caused the overall failure of hundreds of billions of dollars of research and development of traditional vascular drugs for encephalopathy [Citation119].
5.2.3. Brain ECS division may control drug distribution
In 2012, a new method of the magnetic resonance imaging (MRI) with a water-soluble tracer gadolinium diethylenetriaminepentacetic acid (Gd-DTPA) was established to image and quantify the parameters (Vdmax: the probe’s maximal volume distribution; t1/2: the time required for the tracer amount to decrease by half) of brain ECS, which is based on that water molecules in ISF are specifically ‘lightened’ on MRI, allowing the dynamic distribution of brain ISF flow to be traced at the whole brain scale [Citation120,Citation121] ()). Knowledge of effective diffusion coefficients and diffusion properties are equally crucial for defining drug delivery in the brain. Proteins and various macromolecules form part of the traffic within the ECS and are increasingly candidates for new drug vectors, so their diffusion behavior is important [Citation96].
Recent studies on the brain ECS by this approach indicate the presence of a new division system in the brain. ISF in brain ECS cannot distribute or flow globally; but is restricted to certain divisions or territories [Citation7]. The ECS division can be characterized by Vdmax and t1/2. For example, the ratio of Vdmax to brain volume for caudate nucleus is greater than that of the thalamus. The t1/2 of the thalamus is less than that in the caudate nucleus [Citation122]. The traced brain ISF in caudate nuclei flows and distributes to the ipsilateral cortex within 2 h, it then reaches the cortex margin and finally drains into the subarachnoid space [Citation123]. The ISF from caudate nuclei flows toward the ipsilateral cortex and finally drains into the subarachnoid space [Citation120,Citation124]. Moreover, the traced brain ISF in the thalamus does not cross the ‘boundary’ between the thalamus and the caudate nucleus [Citation123,Citation125] ()).
Brain ECS as a narrow extracellular channel is a complex microenvironment which controls neuronal function and acts as a conduit for drug delivery [Citation3]. Notably, visualizing the distribution of a drug in ECS and flowing in ISF at the nanoscale will provide strong evidence for the quantitative and qualitative development of drug delivery in the brain parenchyma. A recent study has found that a kind of carbon-based NPs imaged by near-infrared nanoscopy not only exhibits the structure of brain ECS but also a heterogeneous distribution of these NPs in the brain [Citation106]. Another study has shown that intra-cisterna magna (ICM) injection of an epidermal growth factor receptor-specific humanized IgG1 antibody can across BBB and ECS; however, it are not evenly distributed in the brain [Citation126]. Hence, the brain ECS division most likely controls and determines the distribution range or region of drugs in the brains, which is still need to be investigated in the future.
5.3. Changes of brain ECS and ISF in Alzheimer’s disease
Brain ISF in the ECS constitutes the microenvironment of neurons and is a direct and critical site for exchanging nutritional, metabolic, and neurotransmitter signals [Citation7]. The cerebrospinal fluid (CSF) is considered a ‘reservoir’ of ISF because of its extensive interaction with the ISF in the brain. The earliest finding of ECS change in AD was announced in 2005, and it revealed that Aβ deposition in the ECS damaged synaptic transmission and spatial memory in APP23 transgenic AD model mice [Citation127]. Further, AβO have been proven to flow in the direction of ISF drainage, gradually deposit in the ECS, and impair the synapses [Citation128]. Therefore, Aβ deposited in the ECS blocks ISF flow and disrupts the exchange of signals between the ISF and CSF [Citation129,Citation130].
These two important parameters of ECS, namely, volume fraction (α) and tortuosity (λ), can be quantified by the magnetic tracer method. The value of α is the ratio of the ECS volume to the total brain tissue volume. The value of λ is the ratio of the actual distance between the two points and the distance between the straight lines; which describes the geometric characteristics of the space that the ECS can use for diffusion, that is, the size of the factors that hinder molecular diffusion [Citation120,Citation121]. An increase in the value of α and λ value has been observed in APP/PS1 transgenic mice [Citation131] ()).
Figure 5. Changes in brain ECS and ISF drainage in Alzheimer’s disease, Parkinson’s disease, brain tumor, and multiple sclerosis. (a) A smooth flow of ISF in brain ECS of control mice. (b) A larger tortuosity of ECS in AD model mice. (c) Drug delivery failure in the Aβ-deposited ECS in AD model. d. An increase in α and λ values in Lewy body (LB)-induced PD model mice. This figure was edited from Reference [Citation136]. e. Structure of ECS and ISF flow in ECS among neurons. ECM: extracellular matrix. f-h. Changes in ECS volume fraction in normal brain (f), cytotoxic edema (g), and brain tumor/vasogenic edema (h). Cell: black; nucleus: green; extracellular matrix (ECM): yellow. These figures were edited from Reference [Citation141]. i, j. Alteration of ECS volume and ISF drainage in a demyelinated rat model. This figure was adopted from Reference [Citation98].
![Figure 5. Changes in brain ECS and ISF drainage in Alzheimer’s disease, Parkinson’s disease, brain tumor, and multiple sclerosis. (a) A smooth flow of ISF in brain ECS of control mice. (b) A larger tortuosity of ECS in AD model mice. (c) Drug delivery failure in the Aβ-deposited ECS in AD model. d. An increase in α and λ values in Lewy body (LB)-induced PD model mice. This figure was edited from Reference [Citation136]. e. Structure of ECS and ISF flow in ECS among neurons. ECM: extracellular matrix. f-h. Changes in ECS volume fraction in normal brain (f), cytotoxic edema (g), and brain tumor/vasogenic edema (h). Cell: black; nucleus: green; extracellular matrix (ECM): yellow. These figures were edited from Reference [Citation141]. i, j. Alteration of ECS volume and ISF drainage in a demyelinated rat model. This figure was adopted from Reference [Citation98].](/cms/asset/ef7a9fb1-b7bf-401f-9400-3d67958c021f/iedd_a_2139369_f0005_oc.jpg)
Unsurprisingly, the drugs developed for AD treatment targeting Aβ production, aggregation, deposition, or phosphorylation, aggregation of the tau protein, antibodies, compounds, and small molecules have also failed over the past 100 years [Citation132,Citation133]. More importantly, Aβ-mediated SP was shown to block the ECS and disturb ISF drainage and led to the death of deep neurons in the cortex in AD ()). However, smashing SP in ECS by red-light at 630 nm can reverse ISF flow and improve memory functions in AD model mice. This may be the main reason for the failure of the currently developed drugs to enter the nano-scale ECS of the brains of AD patients [Citation131].
5.4. Changes of brain ECS and ISF in Parkinson’s disease
The α-synuclein misfolds and deposition in ECS of substantia nigra and striatum brain are a typical pathological alteration in PD. Micro-injection of antibodies of α-synuclein in the mouse brain parenchyma has been proved to prevent the spread of it [Citation134]. This suggests that α-synuclein can transfer among brain ECS, and plays a major role in a gradual ECS blockage and a slower speed of ISF drainage, which leads to neuronal degeneration [Citation135].
Recently, an increase in ECS width (median = 114 nm), α and λ values,, and the number of reactive microglia was observed in PD model mice by using electron microscopy and nano-tube probes imaging analysis of brain sections [Citation136] ()). Both slower speed and amount of ISF drainage in ECS were detected in PD model than control rats [Citation137]. A possible explanation is that neuronal degeneration can induce changes in the chondroitin sulfate proteoglycans in ECS, which eventually results in the failure of myelin repair [Citation107]. The integrity of the myelin sheath is fundamental to the normal direction of maintaining the regional ISF drainage system [Citation97]; hence, abnormal ISF drainage in PD patients may be related to the destruction of the myelin sheath.
5.5. Changes of ECS and ISF in brain tumors
Solid tumor tissue has a unique pathological characteristic of ECS, such as: gap fluid pressure, dense tumor outer matrix, ECS blockage etc., all these provide a natural barrier for tumor, make large size nano drugs is difficult to solid tumor internal penetration, and small size nano drugs is easier to overcome the tumor internal high gap fluid pressure, through the dense tumor extracellular matrix (ECM) gap in ECS, more advantageous in deep penetration ()). A marked alteration in these two values of ECS has been observed in patients with glioma. Actually, the results of detecting of glioma with different pathological types by real-time ion introduction and pressure introduction have shown that the ECS volume fraction-α has been significantly increased when compared with a normal value of 0.20, for example, diffuse astrocytoma (α = 0.29 ± 0.01), glioblastoma (α = 0.48 ± 0.01), pilocellular astrocytoma (α = 0.37 ± 0.02), ependyma (α = 0.39 ± 0.01), and medulloblastoma (α = 0.38 ± 0.03), respectively [Citation138,Citation139].
The λ value of ECS is increased in brain glioma patients, which is considered as one of the main reasons for the poor efficacy of anti-brain tumor drugs [Citation139]. Disorders of brain ISF circulation in patients with glioma are characterized by a decline in ISF generation and outflow, especially, accumulation of harmful proteins, inflammatory factors, and pigments in mildly slowed (<3-fold slower than in water) in superficial tumor, but is greatly slowed (>10-fold) at a depth of few millimeters as the tumor tissue becomes more compact [Citation140,Citation141] ()). During tumor growth, ISF drainage in the region near the glioma has been changed; glioma in the caudate nucleus can cause a marked decrease in thalamic ISF flow speed. However, after glioma growth within the thalamus, it induces an alteration in the drainage route of ISF flow in the caudate, but not significantly changed in ISF flow speed [Citation142,Citation143].
5.6. Changes of brain ECS and ISF in multiple sclerosis
Multiple sclerosis is characterized by a chronic progressive loss of myelin around the brain and spinal cord axons. Myelin destruction can lead to neuronal degeneration associated with the loss of axons. Brain ECS plays an important role in the regeneration of the myelin sheath. Abnormal changes in the volume and composition of ECS have been found in patients with multiple sclerosis, including an accumulation in inflammatory factors in ECS, and an increased production of hyaluronic acid and collagen fiber protein [Citation144–147]. An increase in α value but a decrease in λ value associated with a disorder of ISF drainage in the brain regionalization has been found in multiple sclerosis, and the ISF may flow into the separated partition of the blood-cerebrospinal fluid barrier [Citation97] ()). This is the same one pathological phenomenon as that is observed in neurodegenerative diseases, such as: PD, and AD [Citation148]. MS patients were often associated with an increase in the α value but a decrease in λ value of brain ECS [Citation96]. The causes of disturbed ISF drainage are controversial. A possible explanation is that the loss of AQP4 expression in the ependymal cells and astrocytes may be involved in this pathological process [Citation149,Citation150].
Above data indicate that there is a common pathological change that occur in AD, PD and brain tumors, which blocks drug delivery. In fact, an increase in tortuosity of ECS and a decline in the speed and amount of ISF drainage induce drug delivery failure.
6. Application of nanomedicines in brain diseases
It is worth pointing out that the currently developed nanomedicines for brain diseases have achieved some promising results in animal models, but none has been successfully registered clinically in the world [Citation151]. The clinical efficacy of these nanomedicines needs further validation.
6.1. Nanomedicines and brain tumors
For a long time, the traditional drugs for the treatment of brain tumors have high molecular weight and low histocompatibility, so it is difficult to pass through BBB and has a significant cytotoxic effect on normal cells. Compared with the BBB, although blood–tumor barrier (BTB) is leaky generally, but its penetration is not well-distributed. Furthermore, cancer cells exhibit the enhancement of oncogenic, pro-survival signaling. All of these reduce the therapeutic effect of tumor drugs. A variety of nanomedicines using the different mediation methods have developed to treat brain tumors and evaluate their effects. For example, the poly (ethylene glycol)-poly (butylene adipate)-poly (ethylene glycol) superparamagnetic iron oxide NPs loaded with temozolomide have the positive antitumor effect on C6 glioma cells in vitro [Citation152]. The TfR-mediated peptide T12 and mannose-functionalized albumin nanoparticles can pass through BBB and be targeted and delivered into glioma [Citation153]. A functionalized gold-iron oxide NPs containing therapeutic microRNA by intranasal administration can improve the survival time of mouse glioma model [Citation154].
6.2. Nanomedicines and Alzheimer’s disease
Until now, the therapeutic effect of traditional medicine in AD is extremely limited or does not approach to the ideal clinical effects. In order to eliminate the accumulation of Aβ in human brain, an iminodiacetic acid-conjugated NPs can inhibit Aβ aggregation in vitro [Citation155]. The super-large mesoporous silica NPs with the antibody of Aβ monomer can reduce Aβ assembly in AD model mice [Citation156]. The peptide 4F fused angiopep-2 (Ang) lipid NPs reduces the levels of p-Tau and significantly improve the ability of learning and memory in AD model mice [Citation157]. It must be noted that above mentioned nanomedicines have not been applied for treating AD patients.
6.3. Nanomedicines and Parkinson’s disease
PD is a neurodegenerative disease with tremor and motor dysfunction as the principal symptoms. At present, levodopa and dopamine receptor agonists are commonly used in the treatment of PD; however, it has clinical side effects. Intranasal administration of the PLGA-NPs encapsulated with levodopa by solvent evaporation exhibit to some extent positive effects in PD model mice [Citation158]. The Ginkgolide B packaged NPs can alleviate dopamine deficiency, increase the levels of dopamine generation, and improve motor behaviors in MPTP-induced PD model mice [Citation159]. The puerarin encapsulated into 6-arm star-shaped PLGA NPs can release lasted for 48 hours in vitro and improve behaviors in PD animal model [Citation160].
6.4. Nanomedicines and multiple sclerosis
Multiple sclerosis is a neurodegenerative disease that is accompanied by inflammatory infiltration and demyelination of nerve fibers. It is considered to be related to an autoimmune response. At present, interferon (IFN) is a common medicine, which is used to treat this disease [Citation161,Citation162]. The PEG-PLGA NPs containing IFNβ-1a by solvent evaporation exhibit high entrapment efficiency (95.9%) and increase the ability of slow release [Citation163]. Proteolipid protein (PLP) is widely used to regulate the immune system for treating multiple sclerosis [Citation164]. Recently, the PLGA NPs loaded with PLP 139–151 by double emulsion solvent evaporation markedly enhance the effect of sustained release of PLP [Citation165].
7. Limitations of nanomedicines
Since large-molecule drugs can hardly cross BBB for treating brain diseases, NPs packaged medicines have been rapidly developed due to their small particle size, high biocompatibility, low biotoxicity and target ability. At present, there are still some problems of NPs that have to be solved. 1) Most reported nanomedicines are still in the development stage. It is primarily due to the heterogeneity of nano-drug carriers in design and synthesis, and their clinical safety needs further evaluation [Citation166]. 2) Their pharmacokinetic aspects, such as: the way to change the particle size, surface area, and shape of NPs, how to make them have better sustained release effect and long-time effect on the brain. 3) Receptor-mediated endocytosis is commonly used in nano-drug delivery. However, this process may cause oxidative stress, tissue inflammation or DNA damage and so on, which results in cytotoxicity [Citation167]. In particular, nanomedicines dissolve collapse easily in vivo, which causes a high local concentration of drug in the tissues. The optimization on the formulation of drug-loaded NPs to improve performance and reduce side effects has yet to be further investigated [Citation168].
8. Invasive administration for brain diseases
Although noninvasive administration, such as: percutaneous permeation, nasal spray, and orally, can reduce the pain of the patient, but drug efficacy is often slow. Invasive administration methods, such as: brain ECS administration, and intraparenchymal, intraventricular, and intrathecal injection, often require precise surgery and bring pain to patients, but the latter is more accurate and often better effect than the former [Citation169].
8.1. Invasive brain ECS administration for drug delivery
Drug delivery via brain ECS is the direct injection of a therapeutic drug or nanomedicine into the damaged brain regions by using a specialized catheter ()). ISF is consists of water, salts, sugars, fatty acids, amino acids, hormones, neurotransmitters, and other nutrients. Hence, ISF drainage can drive medicines along the brain ECS. The microinfusion of drug directly allows it to reach different regions in the brain by traversing this 38 ~ 64 nm interstitial space. Notably, the different left or right damaged brain region needs to deliver drugs via the different brain regions ECS [Citation121,Citation123,Citation170]. Although more studies are still needed to reveal the locations and properties of all the brain divisions, microinjection of drug via brain ECS have been employed to improve interstitial drug delivery efficiency [Citation171]. These studies also suggest that the brain ECS division most likely controls and determines the distribution range or region of drugs in the brains. This innovative technology is a promising strategy for achieving the therapeutic goal of precision medicine [Citation172].
Figure 6. Multiple patterns of invasive and noninvasive administration for treating brain diseases. a. Brain ECS administration. b. Intraventriclular drug delivery. c. Intrathecal administration. D-E. Noninvasive drug delivery for treating brain diseases, including: sublingual administration (d) and Nasal spray (e). f, g. Phototherapy with red light (RL) at 630 ~ 680 nm or Near-infrared light (NIR) at 780 ~ 1100 nm. h. Mechanisms of phototherapy to reduce Aβ toxicity. i. Near-infrared light (NIR)-activated photosensitive drug release.
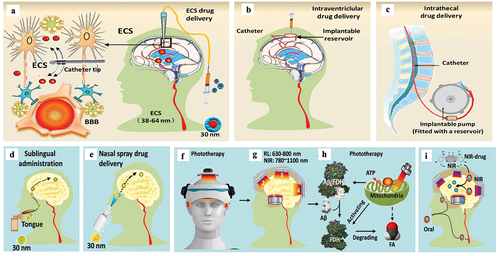
8.2. Intraventricular and intrathecal drug delivery
The therapeutic drug can be administered in one of the ventricles (intraventricular) with an internally implanted reservoir ()), or the spinal canal (intrathecal) with a programmed pump ()). The medicines directly reach the targeted brain region through the cycle of the cerebrospinal fluid (CSF) and ISF. Replenishment of medicines can be obtained by topping up the reservoir or replacing the pump [Citation32–35]. The invasive installation of these two devices needs some complex surgical manipulations by surgeons. Naturally, these processes increase the risk of tissue damage and infection, which are associated with the added recovery time of the patient.
9. Combination of noninvasive therapy and nanomedicine
9.1. Noninvasive sublingual and nasal administrations
Generally, BBB limits the distribution of systemically administered therapeutics to the brain. Sublingual administration involves placing a drug under the tongue, which can deliver drug into the brain [Citation173]. The sublingual route is considered to be a promising alternatives to the traditional oral route for drug delivery [Citation174] ()).
It has found that sublingual levodopa has been implemented in PD patients [Citation175]. Intranasal administration has been noted as a method for noninvasive delivery of a drug to the brain by bypassing the BBB via the ‘nose-to-brain’ route, the olfactory pathway ()). The therapeutic drugs are sprayed into the nasal cavity diffuses through the olfactory sensory nerve and olfactory glomeruli via passive or active transport mechanisms. It then reaches brain tissues via projection neurons, tufted or mitral cells [Citation176]. However, these two pathways are limited by the small amount of curative medicine that can successfully reach the brain tissue, relative to the amount administered [Citation177].
9.2. Noninvasive phototherapy
Substantial studies have demonstrated the efficacy of irradiating tissue with red light (RL: 630 ~ 800 nm) to near-infrared light (NIR: 780 ~ 1100 nm) in mitigating cerebral pathology and degeneration in animal models of stroke, traumatic brain injury [Citation178], PD [Citation179], and AD [Citation180] ()). Although the visible light penetration in the skull is only several millimeters, the near-infrared light (NIR: 780 ~ 1100 nm) can penetrate several centimeters, for example, 3 cm of tissue and 1.2 cm skull [Citation131,Citation181]. There are three possible patterns for the current study of phototherapy in brain disease: 1) Retinal pathway. For example, the 40 Hz white light scintillator via eyes can attenuate the pathological characteristics of AD mice [Citation182]. 2) Non-retinal access, including body surface exposure, endovascular irradiation, nasal exposure, etc. 3) Direct irradiation pathway, including helmet-type transcranial illumination [Citation183]. Among them, low-level laser therapy has positive curative effects on AD, and NIR at ~1000 nm is mostly used [Citation184], but the ‘thermal effect’ of this wavelength is obvious. Its side effects including mild mania, migraine, eye fatigue, nausea, agitation, etc., have been observed in clinical [Citation185]. A phototherapeutic device with RL at shorter wavelength (630 ± 20 nm) has been developed, which has positive clinical effects, less thermal side effects and better security than NIR [Citation186].
9.3. Combination of phototherapy and nano-CoQ10
Recent studies suggest that a combination of phototherapy and nano-Q10 for treating AD may be more therapeutically effective than one of these methods used alone [Citation183,Citation187,Citation188]. Depending on ECS-controlled ISF drainage in the brain, 630-nm red light not only directly removed blockage of the ECS by SP and restored ISF flow, but also activated FDH to degrade FA and reduce Aβ deposition ()). Red light at 630 nm could also directly reduce tau hyperphosphorylation [Citation189]. Moreover, 30-nm Q10 (smaller than the ECS diameter) could enter the ECS and follow the ISF flow into the deep cortical layer to rescue apoptotic neurons [Citation190]. Hence, the combination of these two methods to accelerate ISF drainage will contribute to drug delivery and the recovery of nerve signals.
9.4. Near-infrared photosensitive nanomedicines
During the past three years, NIR as long-wavelength light has been applied to more than 3000 achievements in biological applications due to its deep penetration depth and low phototoxicity. Various materials, especially nanomaterials that can generate reactive oxygen species, ultraviolet/visual light, or thermal energy and so on under NIR illumination achieve great potential for the research of nanomedicine [Citation191] ()). For example, the construction of titanium nitride NPs, with a high photothermal-conversion efficiency and desirable biocompatibility, has been established to act as an alternative theranostic agent for NIR-II laser (1000–1350 nm)-excited photoacoustic imaging-guided photothermal tumor hyperthermia [Citation192]. Another gold-coated mechanoresponsive NPs are consist of liposomes made from the artificial phospholipid Rad-PC-Rad as a tool for the delivery of bioactive molecules into brain tissue. NIR activated the gold-coating on the surface of NPs, creating nanomechanical stress and leading to near-complete vesicle cargo release in sub-seconds in mouse brain [Citation193]. Recently, a novel strategy to target cyclosporine A (CsA) into traumatic brain injury (TBI) site has been developed. The approach is based on TBI treatment with a cyanine dye nano-packed CsA, a known therapeutic agent for TBI that is associated with unacceptable toxicities. In its caged form, CsA remains inactive, while after NIR photoactivation, the resulting fragmentation of the cyanine NPs results in the selective release of CsA at the TBI sites [Citation194,Citation195]. Above studies suggest that the NIR photosensitive nanomedicines are a noninvasive and low toxicity for treating brain disease.
10. Expert opinion
There is no doubt that advantages of nanomedicines are abundant, such as high permeability, targeting distribution, ultimately delivering drug more efficiently. For these reasons, there are 15 types of nanomedicines approved for cancer treatment by regulatory agencies, such as: FDA in USA or the European Medicines Agency (EMA). More than 50 types of nanomedicines are in clinical trials [Citation196].
In general, the special structures of BBB and ECS in the brains are the critical barriers for delivering drugs into the brain. With the increasing of further studies of the molecular biological mechanism of brain diseases, people’s understanding of the brain will be more comprehensive. The risk of tissue damage and infection of invasive administration methods makes people tend to choose noninvasive treatments. Although many drugs for brain diseases have been developed, there will be a more urgent need for brain-targeted nano-drugs. None of the developed traditional drugs for these brain diseases, such as: brain tumors, AD, PD, and multiple sclerosis, has achieved the desired clinical efficacy up to the present.
The development of effective strategies for enhancing drug delivery to the brain has become a topic of great interest in both the clinical and pharmaceutical fields. They have some potential advantages than the traditional drugs. 1) Nanomedicines can pass through BBB and achieve target drug delivery to the desired brain regions. For example, PEG-modified liposomes can pass through BBB and bind to Aβ specifically to diagnose and treat AD [Citation154]. 2) Nanomedicines increase the accumulation of the drug concentrations in the brain by 75% when compared with traditional drug aqueous solution [Citation197]. 3) Nanomedicines can ensure the integrity and biological activity of bioactive substances by avoiding enzyme degradation prematurely, thus improving the stability and bioavailability of drugs. 4) Nanomedicines can reduce the ineffective interaction between drugs and the body, and extend the circulation time of drugs in vivo [Citation198]. 5) Invasive delivering 30 ~ 50 nm nanomedicines via ECS may contribute to the treatment of brain disease. For example, 30 nm coenzyme Q10 (less than the diameter of ECS, 38 ~ 64 nm) shows better curative effects in AD model mice than non-nanoscale-packaged drugs [Citation190].
However, there are still many challenges in the real clinical transformation of nanomedicines-crossing BBB. To address the above questions, developed nanomedicines targeting require further research. First of all, the target mechanism of new nanomedicines is still not clear enough, and there is not enough research on the side effects of many drugs. For example, INR-mediated drug delivery may lead to hypoglycemia and insulin resistance [Citation199], which is conducive to the treatment of brain diseases. The shortcomings of these drugs may make it more difficult to enter clinical research. Secondly, as we mentioned earlier, complex structure for different anisotropic mediators leads unevenness distribution of drugs in the brain. Drugs can’t reach a specific region of the brain. More research is needed on the mechanism of uneven drug distribution. Moreover, some nanomedicines with large diameter are difficult to reach the lesions because of nano-scale structures of ECS. Nanomedicines with smaller diameters need to be developed. Besides, abnormalities in the ECS tortuosity and the speed/amount of ISF drainage have been proven in the incurable brain diseases. It is also a problem that increasing ECS tortuosity and the slow speed of ISF reduce drug transport efficiency.
It is clear that studying the ECS holds great promise for fundamental neuroscience as well as translational research aimed at identifying new drug targets and diagnostic read-outs for brain diseases [Citation200].
In addition, clinical translation requires production at large scale and with GMP manufacturing for reproducible batches of the nanomedicine [Citation201]. However, the composition of many brain targeted nano drugs is complex, leading to the complex preparation process. If these drugs cannot be produced in large quantities, it means that there is a long distance from clinical use of drugs. Simplifying the composition and preparation process of nano-drugs must be put in the first place.
However, some hope with the development of nano-drugs still can be seen. There are many researches on drug transport mediated by liposome NPs and TfR and their safety and efficacy were universally confirmed. More efforts are needed for nano drugs to enter clinical research. In the future, these two kinds of nanomedicines will be more likely to take the lead in clinical applications. Moreover, some studies about improving the efficiency of GULT-mediated drug transport by changing the blood glucose suggest that it is also a good research direction to search for a special way of improving the efficiency of receptor transport. Also, some novel manner of noninvasive phototherapy with RL or NIR has exhibited a positive therapeutic effect in brain disease than the pharmacotherapy. The noninvasive NIR photo-sensitive nanomedicines are a promising strategy for treating brain diseases [Citation194].
In general, with storage and preparation technology improvement, if we choose more suitable packaging methods and drug delivery strategies and make the best of other noninvasive methods, nanomedicines-crossing BBB will enter clinical treatment in the future.
Article highlights
Treatment failures of brain diseases are largely related with BBB, a major obstacle for drug delivery.
Nano-package and receptor-mediated delivery of drug improves the penetration rate of BBB.
A smooth drainage of interstitial fluid (ISF) in brain extracellular space (ECS) determines whether a drug reaches its target cells.
Invasive delivering drug via ECS or noninvasive near-infrared photo-sensitive nanomedicines may be a promising strategy.
Abbreviations
Alzheimer’s disease (AD); BBB: | = | Blood-brain barrier; |
CsA: | = | Cyclosporine A; |
GLUT: | = | Glucose transporter; |
IGFR: | = | Insulin-like growth factors receptor; |
INR: | = | Insulin receptor; |
LRP: | = | Lipoprotein receptor-related protein; |
MSN: | = | Mesoporous silica nano-particles; |
NIR: | = | Near-infrared light; |
NPs: | = | Nanoparticles; |
PLGA: | = | Lactic-co-glycolic acid; |
QDs: | = | Quantum dots; |
Parkinson’s disease (PD) | = | Positron emission tomography imaging (PET); |
RL: | = | Red light |
Declaration of interest
The authors have no relevant affiliations or financial involvement with any organization or entity with a financial interest in or financial conflict with the subject matter or materials discussed in the manuscript. This includes employment, consultancies, honoraria, stock ownership or options, expert testimony, grants or patents received or pending, or royalties.
Reviewer disclosures
Peer reviewers on this manuscript have no other relevant financial relationships or otherwise to disclose.
Author contribution
Ziqi Gu, Haishu Chen, Han Zhao, Wanting Yang, Yilan Song, Xiang Li, Haikang
Liao, Wenhao Pan, and Xi Li: Data curation, writing and original draft preparation. Yang Wang and Dan Du: Data collection, obtained the permission. Yajuan Gao, Hongbin Han, and Zhiqian Tong: Supervision. Ziqi Gu, Haishu Chen, and Zhiqian Tong: Writing, reviewing and editing. All the authors participated in the conception and design, or analysis and interpretation of the data; the drafting of the paper or revising it critically for intellectual content; and the final approval of the version to be published; and that all authors agree to be accountable for all aspects of the work.
Additional information
Funding
References
- Abbott NJ, Patabendige AA, Dolman DE, et al. Structure and function of the blood-brain barrier. Neurobiol Dis. 2010 Jan;37(1):13–25.
- Sweeney MD, Sagare AP, Zlokovic BV. Blood-brain barrier breakdown in Alzheimer disease and other neurodegenerative disorders. Nat Rev Neurol. 2018 Mar;14(3):133–150.
- Nicholson C, Hrabětová S. Brain extracellular space: the final frontier of neuroscience. Biophys J. 2017 Nov 21;113(10):2133–2142.
- Edwards TN, Meinertzhagen IA. The functional organisation of glia in the adult brain of drosophila and other insects. Prog Neurobiol. 2010 Apr;90(4):471–497.
- Deco G, Rolls ET, Albantakis L, et al. Brain mechanisms for perceptual and reward-related decision-making. Prog Neurobiol. 2013 Apr;103:194–213.
- Kastellakis G, Cai DJ, Mednick SC, et al. Synaptic clustering within dendrites: an emerging theory of memory formation. Prog Neurobiol. 2015 Mar;126:19–35.
- Lei Y, Han H, Yuan F, et al. The brain interstitial system: anatomy, modeling, in vivo measurement, and applications. Prog Neurobiol. 2017 Oct;157:230–246.
- Zhang C, Zhou Z, Qian Q, et al. Glutathione-capped fluorescent gold nanoclusters for dual-modal fluorescence/X-ray computed tomography imaging. J Mater Chem B. 2013 Oct 14;1(38):5045–5053.
- Agrawal M, Ajazuddin, Tripathi DK, et al. Recent advancements in liposomes targeting strategies to cross blood-brain barrier (BBB) for the treatment of Alzheimer’s disease. J Control Release. 2017 Aug 28;260:61–77.
- Singh I, Swami R, Pooja D, et al. Lactoferrin bioconjugated solid lipid nanoparticles: a new drug delivery system for potential brain targeting. J Drug Target. 2016;24(3):212–223.
- Saesoo S, Sathornsumetee S, Anekwiang P, et al. Characterization of liposome-containing SPIONs conjugated with anti-CD20 developed as a novel theranostic agent for central nervous system lymphoma. Colloids Surf B Biointerfaces. 2018 Jan 1;161:497–507.
- Kong L, Li XT, Ni YN, et al. Transferrin-modified osthole pegylated liposomes travel the blood-brain barrier and mitigate Alzheimer’s disease-related pathology in APP/PS-1 mice. Int J Nanomedicine. 2020;15:2841–2858.
- Lin CY, Lin YC, Huang CY, et al. Ultrasound-responsive neurotrophic factor-loaded microbubble- liposome complex: preclinical investigation for Parkinson’s disease treatment. J Control Release. 2020 May 10;321:519–528. DOI:10.1016/j.jconrel.2020.02.044.
- Moon H, Hwang K, Nam KM, et al. Enhanced delivery to brain using sonosensitive liposome and microbubble with focused ultrasound. Biomater Adv. 2022 Sep 6;141:213102. DOI:10.1016/j.bioadv.2022.213102.
- Saha S, Yakati V, Shankar G, et al. Amphetamine decorated cationic lipid nanoparticles cross the blood-brain barrier: therapeutic promise for combating glioblastoma. J Mater Chem B. 2020 May 21;8(19):4318–4330.
- Salvati E, Re F, Sesana S, et al. Liposomes functionalized to overcome the blood-brain barrier and to target amyloid-β peptide: the chemical design affects the permeability across an in vitro model. Int J Nanomedicine. 2013;8:1749–1758.
- Wu S, Yang X. OEA loaded liposomes with the neuroprotective effect for stroke therapy. Front Chem. 2022;10:1014208.
- Almeida B, Nag OK, Rogers KE, et al. Recent progress in bioconjugation strategies for liposome-mediated drug delivery. Molecules. 2020;25(23):5672.
- Kapoor DN, Bhatia A, Kaur R, et al. PLGA: a unique polymer for drug delivery. Ther Deliv. 2015 Jan;6(1):41–58.
- Tabatabaei Mirakabad F S, Nejati-Koshki K, Akbarzadeh A, et al. PLGA-based nanoparticles as cancer drug delivery systems. Asian Pac J Cancer Prev. 2014;15(2):517–535.
- Xu K, An N, Zhang H, et al. Sustained-release of PDGF from PLGA microsphere embedded thermo-sensitive hydrogel promoting wound healing by inhibiting autophagy. J Drug Delivery Sci Technol. 2020;55:101405.
- Cai Q, Qiao C, Ning J, et al. A polysaccharide-based hydrogel and PLGA microspheres for sustained P24 peptide delivery: an in vitro and in vivo study based on osteogenic capability. Chem Res Chin Univ. 2019;35(5):908–915.
- Lu Y, Wu F, Duan W, et al. Engineering a “PEG-g-PEI/DNA nanoparticle-in-PLGA microsphere” hybrid controlled release system to enhance immunogenicity of DNA vaccine. Mater Sci Eng C. 2020;106:110294.
- Su Y, Zhang B, Sun R, et al. PLGA-based biodegradable microspheres in drug delivery: recent advances in research and application. Drug Deliv. 2021;28(1):1397–1418.
- Shakeri S, Ashrafizadeh M, Zarrabi A, et al. Multifunctional polymeric nanoplatforms for brain diseases diagnosis, therapy and theranostics. Biomedicines. 2020;8(1):13.
- Shah SR, Kim J, Schiapparelli P, et al. Verteporfin-loaded polymeric microparticles for intratumoral treatment of brain cancer. Mol Pharm. 2019 Apr 1;16(4):1433–1443.
- Cunha A, Gaubert A, Verget J, et al. Trehalose-based nucleolipids as nanocarriers for autophagy modulation: an in vitro study. Pharmaceutics. 2022 Apr 13;14(4).
- Gajbhiye KR, Gajbhiye V, Siddiqui IA, et al. Ascorbic acid tethered polymeric nanoparticles enable efficient brain delivery of galantamine: an in vitro-in vivo study. Sci Rep. 2017 Sep 11;7(1):11086.
- Gholamzad M, Baharlooi H, Shafiee Ardestani M, et al. Prophylactic and therapeutic effects of MOG-Conjugated PLGA nanoparticles in C57Bl/6 mouse model of multiple sclerosis. Adv Pharm Bull. 2021 May;11(3):505–513.
- Loureiro JA, Gomes B, Fricker G, et al. Cellular uptake of PLGA nanoparticles targeted with anti-amyloid and anti-transferrin receptor antibodies for Alzheimer’s disease treatment. Colloids Surf B Biointerfaces. 2016 Sep 1;145:8–13.
- Smiley SB, Yun Y, Ayyagari P, et al. Development of CD133 targeting multi-drug polymer micellar nanoparticles for glioblastoma - in vitro evaluation in glioblastoma stem cells. Pharm Res. 2021 Jun;38(6):1067–1079.
- Mansur HS. Quantum dots and nanocomposites. Wiley Interdiscip Rev Nanomed Nanobiotechnol. 2010;2(2):113–129.
- Ekimov AI, Onushchenko AA. Quantum size effect in three-dimensional microscopic semiconductor crystals. ZhETF Pisma Redaktsiiu. 1981;34:363.
- Perini G, Palmieri V, Ciasca G, et al. Unravelling the potential of graphene quantum dots in biomedicine and neuroscience. Int J Mol Sci. 2020;21(10):3712.
- Edis Z, Wang J, Waqas MK, et al. Nanocarriers-mediated drug delivery systems for anticancer agents: an overview and perspectives. Int J Nanomedicine. 2021;16:1313–1330.
- Matea CT, Mocan T, Tabaran F, et al. Quantum dots in imaging, drug delivery and sensor applications. Int J Nanomedicine. 2017;12:5421.
- Díaz-González M, de la Escosura-Muñiz A, Fernandez-Argüelles MT, et al. Quantum dot bioconjugates for diagnostic applications. Top Curr Chem (Cham). 2020 Mar 26;378(2):35.
- Li H, Zhang Y, Ding J, et al. Synthesis of carbon quantum dots for application of alleviating amyloid-β mediated neurotoxicity. Colloids Surf B Biointerfaces. 2022 Apr;212:112373.
- Y-P H, Leong KW. Quantum dot-based theranostics. Nanoscale. 2010;2(1):60–68.
- Sukhanova A, Bozrova S, Gerasimovich E, et al. Dependence of quantum dot toxicity in vitro on their size, chemical composition, and surface charge. Nanomaterials (Basel). 2022 Aug 9;12(16). 10.3390/nano12162734
- Liu N, Tang M. Toxicity of different types of quantum dots to mammalian cells in vitro: an update review. J Hazard Mater. 2020 Nov 15;399:122606.10.1016/j.jhazmat.2020.122606
- Liang Y, Zhang T, Tang M. Toxicity of quantum dots on target organs and immune system. J Appl Toxicol. 2022 Jan;42(1):17–40.
- Li S, Skromne I, Peng Z, et al. “Dark” carbon dots specifically “light-up” calcified zebrafish bones. J Mater Chem B. 2016 Dec 14;4(46):7398–7405.
- Kresge A, Leonowicz M, Roth WJ, et al. Ordered mesoporous molecular sieves synthesized by a liquid-crystal template mechanism. Nature. 1992;359(6397):710–712.
- Singh P, Srivastava S, Singh SK. Nanosilica: recent progress in synthesis, functionalization, biocompatibility, and biomedical applications. ACS Biomater Sci Eng. 2019;5(10):4882–4898.
- IUPAC. Manual of symbols and terminology ApPCaSCPaAC. 1972;31:579–638.
- Song Y, Du D, Li L, et al. In vitro study of receptor-mediated silica nanoparticles delivery across blood-brain barrier. ACS Appl Mater Interfaces. 2017 Jun 21;9(24):20410–20416.
- Pellen-Mussi P, Tricot-Doleux S, Neaime C, et al. Evaluation of functional SiO2 nanoparticles toxicity by a 3D culture model. J Nanosci Nanotechnol. 2018;18(5):3148–3157.
- Qian ZM, Tang PL. Mechanisms of iron uptake by mammalian cells. Biochim Biophys Acta Mol Cell Res. 1995;1269(3):205–214.
- Z-q Y, Wu Q, Zhou X-M, et al. Receptor-mediated delivery of Astaxanthin-loaded nanoparticles to neurons: an enhanced potential for subarachnoid hemorrhage treatment. Front Neurosci. 2019;13:989.
- Nilsson KG. Preparation of nanoparticles conjugated with enzyme and antibody and their use in heterogeneous enzyme immunoassays. J Immunol Methods. 1989;122(2):273–277.
- Ramalho MJ, Loureiro JA, Coelho MAN, et al. Transferrin receptor-targeted nanocarriers: overcoming barriers to treat glioblastoma. Pharmaceutics. 2022 Jan 25;14(2). DOI:10.3390/pharmaceutics14020279.
- Neves A, van der Putten L, Queiroz J, et al. Transferrin-functionalized lipid nanoparticles for curcumin brain delivery. J Biotechnol. 2021;331:108–117.
- Lan W, Zhang H, Yang B. Preliminary study on the therapeutic effect of doxorubicin-loaded targeting nanoparticles on glioma. Appl Bionics Biomech. 2022;2022. DOI:10.1155/2022/6405400.
- Loureiro JA, Gomes B, Coelho MA, et al. Targeting nanoparticles across the blood–brain barrier with monoclonal antibodies. Nanomedicine. 2014;9(5):709–722.
- Kuang Y, An S, Guo Y, et al. T7 peptide-functionalized nanoparticles utilizing RNA interference for glioma dual targeting. Int J Pharm. 2013;454(1):11–20.
- Kucharz K, Kristensen K, Johnsen KB, et al. Post-capillary venules are the key locus for transcytosis-mediated brain delivery of therapeutic nanoparticles. Nat Commun. 2021 Jul 5;12(1):4121.
- Johnsen KB, Bak M, Kempen PJ, et al. Antibody affinity and valency impact brain uptake of transferrin receptor-targeted gold nanoparticles. Theranostics. 2018;8(12):3416–3436.
- Johnsen KB, Bak M, Melander F, et al. Modulating the antibody density changes the uptake and transport at the blood-brain barrier of both transferrin receptor-targeted gold nanoparticles and liposomal cargo. J Control Release. 2019 Feb 10;295:237–249.
- Herz J, Hamann U, Rogne S, et al. Surface location and high affinity for calcium of a 500‐kd liver membrane protein closely related to the LDL‐receptor suggest a physiological role as lipoprotein receptor. EMBO J. 1988;7(13):4119–4127.
- Lillis AP, Van Duyn LB, Murphy-Ullrich JE, et al. LDL receptor-related protein 1: unique tissue-specific functions revealed by selective gene knockout studies. Physiol Rev. 2008;88(3):887–918.
- Maletínská L, Blakely EA, Bjornstad KA, et al. Human glioblastoma cell lines: levels of low-density lipoprotein receptor and low-density lipoprotein receptor-related protein. Cancer Res. 2000;60(8):2300–2303.
- Anami Y, Xiong W, Yamaguchi A, et al. Homogeneous antibody–angiopep 2 conjugates for effective brain targeting. RSC Adv. 2022;12(6):3359–3364.
- Regina A, Demeule M, Tripathy S, et al. ANG4043, a novel brain-penetrant peptide–mAb conjugate, is efficacious against HER2-positive intracranial tumors in mice. Mol Cancer Ther. 2015;14(1):129–140.
- Zhang B, Xue R, Sun C. Rational design of ROS-responsive nanocarriers for targeted X-ray-induced photodynamic therapy and cascaded chemotherapy of intracranial glioblastoma. Nanoscale. 2022;14:5054–5067.
- Fillebeen C, Descamps L, Dehouck MP, et al. Receptor-mediated transcytosis of lactoferrin through the blood-brain barrier. J Biol Chem. 1999 Mar 12;274(11):7011–7017.
- Qi N, Zhang S, Zhou X, et al. Combined integrin α(v)β(3) and lactoferrin receptor targeted docetaxel liposomes enhance the brain targeting effect and anti-glioma effect. J Nanobiotechnology. 2021 Dec 23;19(1):446.
- Teixeira MI, Lopes CM, Gonçalves H, et al. Formulation, characterization, and cytotoxicity evaluation of lactoferrin functionalized lipid nanoparticles for riluzole delivery to the brain. Pharmaceutics. 2022;14(1):185.
- Ahmad F, Sun Q, Patel D, et al. Cholesterol metabolism: a potential therapeutic target in glioblastoma. Cancers (Basel). 2019 Jan 26;11(2). DOI:10.3390/cancers11020146.
- Hong DY, Lee DH, Lee JY, et al. Relationship between brain metabolic disorders and cognitive impairment: LDL receptor defect. Int J Mol Sci. 2022 Jul 29;23(15). DOI:10.3390/ijms23158384.
- Lee J, Pilch PF. The insulin receptor: structure, function, and signaling. Am J Physiol Cell Physiol. 1994;266(2):C319–C334.
- Rechler MM, Nissley SP. Insulin-like growth factor (IGF)/somatomedin receptor subtypes: structure, function, and relationships to insulin receptors and IGF carrier proteins. Horm Res. 1986;24(2–3):152–159.
- Messier C, Teutenberg K. The role of insulin, insulin growth factor, and insulin-degrading enzyme in brain aging and Alzheimer’s disease. Neural Plast. 2005;12(4):311–328.
- Shilo M, Motiei M, Hana P, et al. Transport of nanoparticles through the blood–brain barrier for imaging and therapeutic applications. Nanoscale. 2014;6(4):2146–2152.
- Boado RJ, Zhang Y, Zhang Y, et al. Humanization of anti-human insulin receptor antibody for drug targeting across the human blood-brain barrier. Biotechnol Bioeng. 2007 Feb 1;96(2):381–391.
- Giugliani R, Giugliani L, de Oliveira Poswar F, et al. Neurocognitive and somatic stabilization in pediatric patients with severe mucopolysaccharidosis type I after 52 weeks of intravenous brain-penetrating insulin receptor antibody-iduronidase fusion protein (valanafusp alpha): an open label phase 1-2 trial. Orphanet J Rare Dis. 2018 Jul 5;13(1):110.
- Rip J, Schenk G, De Boer A. Differential receptor-mediated drug targeting to the diseased brain. Expert Opin Drug Deliv. 2009;6(3):227–237.
- Shukla RK, Tiwari A. Carbohydrate molecules: an expanding horizon in drug delivery and biomedicine. Critical Reviews™ in Therapeutic Drug Carrier Systems. 2011;28:255–292.
- Chen F, Huang G, Huang H. Sugar ligand-mediated drug delivery. Future Med Chem. 2020;12(2):161–171.
- Holman GD. Chemical biology probes of mammalian GLUT structure and function. Biochem J. 2018;475(22):3511–3534.
- Anraku Y, Kuwahara H, Fukusato Y, et al. Glycaemic control boosts glucosylated nanocarrier crossing the BBB into the brain. Nat Commun. 2017 Oct 17;8(1):1001.
- Zhang CX, Zhao WY, Liu L, et al. A nanostructure of functional targeting epirubicin liposomes dually modified with aminophenyl glucose and cyclic pentapeptide used for brain glioblastoma treatment. Oncotarget. 2015 Oct 20;6(32):32681–32700.
- Agrawal P, Singh RP, Sonali, et al. TPGS-chitosan cross-linked targeted nanoparticles for effective brain cancer therapy. Mater Sci Eng C Mater Biol Appl. 2017 May 1;74:167–176. 10.1016/j.msec.2017.02.008
- Bao Q, Hu P, Xu Y, et al. Simultaneous blood-brain barrier crossing and protection for stroke treatment based on edaravone-loaded ceria nanoparticles. ACS Nano. 2018 Jul 24;12(7):6794–6805.
- Cui Y, Xu Q, Chow PK, et al. Transferrin-conjugated magnetic silica PLGA nanoparticles loaded with doxorubicin and paclitaxel for brain glioma treatment. Biomaterials. 2013 Nov;34(33):8511–8520.
- Di Mauro PP, Cascante A, Brugada Vilà P, et al. Peptide-functionalized and high drug loaded novel nanoparticles as dual-targeting drug delivery system for modulated and controlled release of paclitaxel to brain glioma. Int J Pharm. 2018 Dec 20;553(1–2):169–185.
- Fu S, Liang M, Wang Y, et al. Dual-modified novel biomimetic nanocarriers improve targeting and therapeutic efficacy in glioma. ACS Appl Mater Interfaces. 2019 Jan 16;11(2):1841–1854.
- Jiang X, Xin H, Ren Q, et al. Nanoparticles of 2-deoxy-D-glucose functionalized poly(ethylene glycol)-co-poly(trimethylene carbonate) for dual-targeted drug delivery in glioma treatment. Biomaterials. 2014 Jan;35(1):518–529.
- Pardridge WM, Boado RJ, Giugliani R, et al. Plasma pharmacokinetics of valanafusp alpha, a human insulin receptor antibody-iduronidase fusion protein, in patients with mucopolysaccharidosis type I. BioDrugs. 2018 Apr;32(2):169–176.
- Patil R, Portilla-Arias J, Ding H, et al. Temozolomide delivery to tumor cells by a multifunctional nano vehicle based on poly(β-L-malic acid). Pharm Res. 2010 Nov;27(11):2317–2329.
- Porru M, Zappavigna S, Salzano G, et al. Medical treatment of orthotopic glioblastoma with transferrin-conjugated nanoparticles encapsulating zoledronic acid. Oncotarget. 2014 Nov 15;5(21):10446–10459.
- Shen J, Zhao Z, Shang W, et al. Fabrication and evaluation a transferrin receptor targeting nano-drug carrier for cerebral infarction treatment. Artif Cells Nanomed Biotechnol. 2019 Dec;47(1):192–200.
- Shen Y, Cao B, Snyder NR, et al. ROS responsive resveratrol delivery from LDLR peptide conjugated PLA-coated mesoporous silica nanoparticles across the blood-brain barrier. J Nanobiotechnology. 2018 Feb 13;16(1):13.
- Wang W, Lin X, Dong X, et al. A multi-target theranostic nano-composite against Alzheimer’s disease fabricated by conjugating carbon dots and triple-functionalized human serum albumin. Acta Biomater. 2022 Aug;148:298–309.
- Xie J, Gonzalez-Carter D, Tockary TA, et al. Dual-sensitive nanomicelles enhancing systemic delivery of therapeutically active antibodies specifically into the brain. ACS Nano. 2020 Jun 23;14(6):6729–6742.
- Syková E, Nicholson C. Diffusion in brain extracellular space. Physiol Rev. 2008 Oct;88(4):1277–1340.
- Wang A, Wang R, Cui D, et al. The drainage of interstitial fluid in the deep brain is controlled by the integrity of myelination. Aging Dis. 2019 Oct;10(5):937–948.
- Wang R, Han H, Shi K, et al. The alteration of brain interstitial fluid drainage with myelination development. Aging Dis. 2021 Oct;12(7):1729–1740.
- Gitler AD, Lu MM, Epstein JA. PlexinD1 and semaphorin signaling are required in endothelial cells for cardiovascular development. Dev Cell. 2004 Jul;7(1):107–116.
- Torres-Vazquez J, Gitler AD, Fraser SD, et al. Semaphorin-plexin signaling guides patterning of the developing vasculature. Dev Cell. 2004 Jul;7(1):117–123.
- Xianjie Cai QH, Wang W, Chunlin L, et al. Epidural pulsation accelerates the drainage of brain interstitial fluid. Aging Dis. 2022. DOI:10.14336/AD.2022.0609
- Zanotti-Fregonara P, Leroy C, Roumenov D, et al. Kinetic analysis of [11C]befloxatone in the human brain, a selective radioligand to image monoamine oxidase A. EJNMMI Res. 2013 Nov 25;3(1):78.
- Bourasset F, Auvity S, Thorne RG, et al. Brain distribution of drugs: brain morphology, delivery routes, and species differences. Handb Exp Pharmacol. 2022;273:97–120.
- Pardridge WM. Drug transport across the blood-brain barrier. J Cereb Blood Flow Metab. 2012 Nov;32(11):1959–1972.
- Liu Y, Zou Y, Feng C, et al. Charge conversional biomimetic nanocomplexes as a multifunctional platform for boosting orthotopic glioblastoma RNAi therapy. Nano Lett. 2020 Mar 11;20(3):1637–1646.
- Paviolo C, Cognet L. Near-infrared nanoscopy with carbon-based nanoparticles for the exploration of the brain extracellular space. Neurobiol Dis. 2021 Jun;153:105328.
- Lau LW, Cua R, Keough MB, et al. Pathophysiology of the brain extracellular matrix: a new target for remyelination. Nat Rev Neurosci. 2013 Oct;14(10):722–729.
- Hrabetova S, Nicholson C. Contribution of dead-space microdomains to tortuosity of brain extracellular space. Neurochem Int. 2004 Sep;45(4):467–477.
- KT FJ. Drug “diffusion” within the brain . Ann N Y Acad Sci. 1988;531:29–39.
- Godin AG, Varela JA, Gao Z, et al. Single-nanotube tracking reveals the nanoscale organization of the extracellular space in the live brain. Nat Nanotechnol. 2017 Mar;12(3):238–243.
- Tonnesen J, Inavalli V, Nagerl UV. Super-resolution imaging of the extracellular space in living brain tissue. Cell. 2018 Feb 22;172(5):1108–1121 e15.
- Soria FN, Miguelez C, Penagarikano O, et al. Current techniques for investigating the brain extracellular space. Front Neurosci. 2020;14:570750.
- Tao CNPK-ZL. Brain extracellular space as a diffusion barrier. Comput Visual Sci. 2011;14(7):309–325.
- Stern P. Tracking extracellular space in the brain. Science. 2016 Dec 23;354(6319):1547–1548.
- Xie L, Kang H, Xu Q, et al. Sleep drives metabolite clearance from the adult brain. Science. 2013 Oct 18;342(6156):373–377.
- Mansor NI, Nordin N, Mohamed F, et al. Crossing the blood-brain barrier: a review on drug delivery strategies for treatment of the central nervous system diseases. Curr Drug Deliv. 2019;16(8):698–711.
- Misra A, Ganesh S, Shahiwala A, et al. Drug delivery to the central nervous system: a review. J Pharm Pharm Sci. 2003 May-Aug;6(2):252–273.
- Hoshyar N, Gray S, Han H, et al. The effect of nanoparticle size on in vivo pharmacokinetics and cellular interaction. Nanomedicine (Lond). 2016 Mar;11(6):673–692.
- Fisher M, Feuerstein G, Howells DW, et al. Update of the stroke therapy academic industry roundtable preclinical recommendations. Stroke. 2009 Jun;40(6):2244–2250.
- Han H, Li K, Yan J, et al. An in vivo study with an MRI tracer method reveals the biophysical properties of interstitial fluid in the rat brain. Sci China Life Sci. 2012 Sep;55(9):782–787.
- Han HB. In vivo quantitative measurement of diffusion parameters in brain extracellular space of rat by using magnetic resonance imaging. Beijing Da Xue Xue Bao Yi Xue Ban. 2012 Oct 18;44(5):770–775.
- Zuo LLK, Han H. Comparative analysis by magnetic resonance imaging of extracellular space diffusion and interstitial fluid flow in the rat striatum and thalamus. Appl Magn Reson. 2015;46(6):623–632.
- Han H, Shi C, Fu Y, et al. A novel MRI tracer-based method for measuring water diffusion in the extracellular space of the rat brain. IEEE J Biomed Health Inform. 2014 May;18(3):978–983.
- Pardridge WM. Drug transport in brain via the cerebrospinal fluid. Fluids Barriers CNS. 2011 Jan 18;8(1):7.
- Shi CLY, Han H. Transportation in the interstitial space of the brain can be regulated by neuronal excitation. Sci Rep. 2015;3(5):17673.
- Naseri Kouzehgarani PK G, Bolin SE, Reilly EB, et al. Biodistribution analysis of an anti-egfr antibody in the rat brain: validation of CSF microcirculation as a viable pathway to circumvent the blood-brain barrier for drug delivery. Pharmaceutics. 2022;14(7):1441.
- Sykova E, Vorisek I, Antonova T, et al. Changes in extracellular space size and geometry in APP23 transgenic mice: a model of Alzheimer’s disease. Proc Natl Acad Sci U S A. 2005 Jan 11;102(2):479–484.
- Hong S, Ostaszewski BL, Yang T, et al. Soluble Abeta oligomers are rapidly sequestered from brain ISF in vivo and bind GM1 ganglioside on cellular membranes. Neuron. 2014 Apr 16;82(2):308–319.
- Jessen NA, Munk AS, Lundgaard I, et al. The glymphatic system: a beginner’s guide. Neurochem Res. 2015 Dec;40(12):2583–2599.
- Thrane AS, Rangroo Thrane V, Nedergaard M. Drowning stars: reassessing the role of astrocytes in brain edema. Trends Neurosci. 2014 Nov;37(11):620–628.
- Yue X, Mei Y, Zhang Y, et al. New insight into Alzheimer’s disease: light reverses Abeta-obstructed interstitial fluid flow and ameliorates memory decline in APP/PS1 mice. Alzheimers Dement (N Y). 2019;5(1):671–684.
- Cummings J, Lee G, Ritter A, et al. Alzheimer’s disease drug development pipeline: 2018. Alzheimers Dement (N Y). 2018;4:195–214. DOI:10.1016/j.trci.2018.03.009.
- Mangialasche F, Solomon A, Winblad B, et al. Alzheimer’s disease: clinical trials and drug development. Lancet Neurol. 2010 Jul;9(7):702–716.
- Tran HT, Chung CH, Iba M, et al. Α-synuclein immunotherapy blocks uptake and templated propagation of misfolded α-synuclein and neurodegeneration. Cell Rep. 2014 Jun 26;7(6):2054–2065.
- Moretto E, Stuart S, Surana S, et al The role of extracellular matrix components in the spreading of pathological protein aggregates. Front Cell Neurosci. 2022;16:844211.
- Soria FN, Paviolo C, Doudnikoff E, et al. Synucleinopathy alters nanoscale organization and diffusion in the brain extracellular space through hyaluronan remodeling. Nat Commun. 2020 Jul 10;11(1):3440.
- Lv D, Li J, Li H, et al. Imaging and quantitative analysis of the interstitial space in the caudate nucleus in a rotenone-induced rat model of Parkinson’s disease using tracer-based MRI. Aging Dis. 2017 Feb;8(1):1–6.
- Zámecník J, Vargová L, Homola A, et al. Extracellular matrix glycoproteins and diffusion barriers in human astrocytic tumours. Neuropathol Appl Neurobiol. 2004 Aug;30(4):338–350.
- Syková E, Vargová L. Extrasynaptic transmission and the diffusion parameters of the extracellular space. Neurochem Int. 2008 Jan;52(1–2):5–13.
- Verkman AS. Diffusion in the extracellular space in brain and tumors. Phys Biol. 2013 Aug;10(4):045003.
- Papadopoulos MC, Binder DK, Verkman AS. Enhanced macromolecular diffusion in brain extracellular space in mouse models of vasogenic edema measured by cortical surface photobleaching. FASEB J. 2005 Mar;19(3):425–427.
- Guan X, Wang W, Wang A, et al. Brain interstitial fluid drainage alterations in glioma-bearing rats. Aging Dis. 2018;9(2):228–234.
- Li K, Han H, Zhu K, et al. Real-time magnetic resonance imaging visualization and quantitative assessment of diffusion in the cerebral extracellular space of C6 glioma-bearing rats. Neurosci Lett. 2013 May 24;543:84–89. 10.1016/j.neulet.2013.02.071
- Davalos D, Akassoglou K. Fibrinogen as a key regulator of inflammation in disease. Semin Immunopathol. 2012 Jan;34(1):43–62.
- Ghorbani S, Yong VW. The extracellular matrix as modifier of neuroinflammation and remyelination in multiple sclerosis. Brain. 2021 Aug 17;144(7):1958–1973.
- Back SA, Tuohy TM, Chen H, et al. Hyaluronan accumulates in demyelinated lesions and inhibits oligodendrocyte progenitor maturation. Nat Med. 2005 Sep;11(9):966–972.
- Mohan H, Krumbholz M, Sharma R, et al. Extracellular matrix in multiple sclerosis lesions: fibrillar collagens, biglycan and decorin are upregulated and associated with infiltrating immune cells. Brain Pathol. 2010 Sep;20(5):966–975.
- Simon MJ, Iliff JJ. Regulation of cerebrospinal fluid (CSF) flow in neurodegenerative, neurovascular and neuroinflammatory disease. Biochim Biophys Acta. 2016 Mar;1862(3):442–451.
- Simon M, Wang MX, Ismail O, et al. Loss of perivascular aquaporin-4 localization impairs glymphatic exchange and promotes amyloid beta plaque formation in mice. Alzheimers Res Ther. 2022 Apr 26;14(1):59.
- Teng Z, Wang A, Wang P, et al. The effect of aquaporin-4 knockout on interstitial fluid flow and the structure of the extracellular space in the deep brain. Aging Dis. 2018 Oct;9(5):808–816.
- Tosi G, Thomas Duskey J, Angela Vandelli M, et al. Nanomedicines for brain diseases: where we are and where we are going. Ther Deliv. 2021 Sep;12(9):631–635.
- Emamgholizadeh Minaei S, Khoei S, Khoee S, et al. Tri-block copolymer nanoparticles modified with folic acid for temozolomide delivery in glioblastoma. Int J Biochem Cell Biol. 2019 Mar;108:72–83.
- Zhao P, Wang Y, Kang X, et al. Dual-targeting biomimetic delivery for anti-glioma activity via remodeling the tumor microenvironment and directing macrophage-mediated immunotherapy. Chem Sci. 2018 Mar 14;9(10):2674–2689.
- Sukumar UK, Bose RJC, Malhotra M, et al. Intranasal delivery of targeted polyfunctional gold-iron oxide nanoparticles loaded with therapeutic microRNAs for combined theranostic multimodality imaging and presensitization of glioblastoma to temozolomide. Biomaterials. 2019 Oct;218:119342.
- Liu H, Dong X, Liu F, et al. Iminodiacetic acid-conjugated nanoparticles as a bifunctional modulator against Zn(2+)-mediated amyloid β-protein aggregation and cytotoxicity. J Colloid Interface Sci. 2017 Nov 1;505:973–982. DOI:10.1016/j.jcis.2017.06.093.
- Jung H, Chung YJ, Wilton R, et al. Silica nanodepletors: targeting and clearing Alzheimer’s β‐amyloid plaques. Adv Funct Mater. 2020;30(15):1910475.
- Han G, Bai K, Yang X, et al. “Drug-Carrier” synergy therapy for Amyloid- β clearance and inhibition of tau phosphorylation via biomimetic lipid nanocomposite assembly. Adv Sci. 2022;9(14):2106072.
- Arisoy S, Sayiner O, Comoglu T, et al. In vitro and in vivo evaluation of levodopa-loaded nanoparticles for nose to brain delivery. Pharm Dev Technol. 2020 Jul;25(6):735–747.
- Liu Y, Liu W, Xiong S, et al. Highly stabilized nanocrystals delivering ginkgolide B in protecting against the Parkinson’s disease. Int J Pharm. 2020 Mar 15;577:119053. 10.1016/j.ijpharm.2020.119053
- Chen T, Liu W, Xiong S, et al. Nanoparticles mediating the sustained puerarin release facilitate improved brain delivery to treat Parkinson’s disease. ACS Appl Mater Interfaces. 2019 Dec 4;11(48):45276–45289.
- Baumhefner RW, Leng M. Standard dose weekly intramuscular beta interferon-1a may be inadequate for some patients with multiple sclerosis: a 19-year clinical experience using twice a week dosage. Neurol Ther. 2022 Jul 7;113. 10.1007/s40120-022-00377-1
- van Ballegooijen H, van der Hiele K, Enzinger C, et al. The longitudinal relationship between fatigue, depression, anxiety, disability, and adherence with cognitive status in patients with early multiple sclerosis treated with interferon beta-1a. eNeurologicalSci. 2022 Sep;28:100409.
- Fodor-Kardos A, Kiss ÁF, Monostory K, et al. Sustained in vitro interferon-beta release and in vivo toxicity of PLGA and PEG-PLGA nanoparticles. RSC Adv. 2020;10(27):15893–15900.
- Greer JM, Trifilieff E, Pender MP. Correlation between anti-myelin proteolipid protein (PLP) antibodies and disease severity in multiple sclerosis patients with PLP response-permissive HLA types. Front Immunol. 2020;11:1891.
- Lima AF, Amado IR, Pires LR. Poly(d,l-lactide-co-glycolide) (PLGA) nanoparticles loaded with proteolipid protein (PLP)-exploring a new administration route. Polymers (Basel). 2020 Dec 21;12(12). DOI:10.3390/polym12123063.
- Wong XY, Sena-Torralba A, Alvarez-Diduk R, et al. Nanomaterials for nanotheranostics: tuning their properties according to disease needs. ACS nano. 2020;14(3):2585–2627.
- Najahi-Missaoui W, Arnold RD, Cummings BS. Safe nanoparticles: are we there yet?. Int J Mol Sci. 2020 Dec 31;221. DOI:10.3390/ijms22010385.
- Awasthi R, Roseblade A, Hansbro PM, et al. Nanoparticles in cancer treatment: opportunities and obstacles. Curr Drug Targets. 2018;19(14):1696–1709.
- Chen KS, Chen R. Invasive and noninvasive brain stimulation in Parkinson’s disease: clinical effects and future perspectives. Clin Pharmacol Ther. 2019 Oct;106(4):763–775.
- He QY, Han HB, Xu FJ, et al. Imaging and quantitative measurement of brain extracellular space using MRI Gd-DTPA tracer method. Beijing Da Xue Xue Bao Yi Xue Ban. 2010 Apr 18;42(2):188–191.
- Xu F, Hongbin H, Yan J, et al. Greatly improved neuroprotective efficiency of citicoline by stereotactic delivery in treatment of ischemic injury. Drug Deliv. 2011 Sep-Oct;18(7):461–467.
- Fan C, Tian F, Zhao X, et al. The effect of thymoquinone on the characteristics of the brain extracellular space in transient middle cerebral artery occlusion rats. Biol Pharm Bull. 2020;43(9):1306–1314.
- Deplanque D, Lavallee PC, Labreuche J, et al. Cerebral and extracerebral vasoreactivity in symptomatic lacunar stroke patients: a case-control study. Int J Stroke. 2013 Aug;8(6):413–421.
- Hua S. Advances in nanoparticulate drug delivery approaches for sublingual and buccal administration. Front Pharmacol. 2019;10:1328.
- Bhirud PH, Kate JA. Perioperative sublingual levodopa in Parkisnon’s Disease: a useful alternative!. Indian J Anaesth. 2017 May;61(5):432–434.
- Kanazawa T. Development of noninvasive drug delivery systems to the brain for the treatment of brain/central nervous system diseases. Yakugaku Zasshi. 2018;138(4):443–450.
- Abdul Razzak R, Florence GJ, Gunn-Moore FJ. Approaches to CNS drug delivery with a focus on transporter-mediated transcytosis. Int J Mol Sci. 2019 Jun 25;20 12. DOI:10.3390/ijms20123108.
- Hennessy M, Hamblin MR. Photobiomodulation and the brain: a new paradigm. J Opt. 2017 Jan;19(1):013003.
- Stepanov YV, Golovynska I, Zhang R, et al. Near-infrared light reduces beta-amyloid-stimulated microglial toxicity and enhances survival of neurons: mechanisms of light therapy for Alzheimer’s disease. Alzheimers Res Ther. 2022 Jun 18;14(1):84.
- Johnstone DM, Moro C, Stone J, et al. Turning on lights to stop neurodegeneration: the potential of near infrared light therapy in Alzheimer’s and Parkinson’s disease. Front Neurosci. 2015;9:500.
- Henderson TA, Morries LD. Near-infrared photonic energy penetration: can infrared phototherapy effectively reach the human brain?. Neuropsychiatr Dis Treat. 2015;11:2191–2208.
- Iaccarino HF, et al. Gamma frequency entrainment attenuates amyloid load and modifies microglia. Nature. 2016;540(7632):230–235.
- Hamblin MR. Photobiomodulation for Alzheimer’s disease: has the light dawned?. Photonics. 2019 Sep;6(3).
- Enengl J, Hamblin MR, Dungel P. Photobiomodulation for Alzheimer’s disease: translating basic research to clinical application. J Alzheimers Dis. 2020;75(4):1073–1082.
- Terman M. Bright light therapy: side effects and benefits across the symptom spectrum. J Clin Psychiatry. 1999;60(11):799–808.
- Huang N, Yao D, Jiang W, et al. Safety and efficacy of 630-nm red light on cognitive function in older adults with mild to moderate Alzheimer’s disease: protocol for a randomized controlled study. Front Aging Neurosci. 2020;12:143.
- Salehpour F, Farajdokht F, Mahmoudi J, et al. Photobiomodulation and coenzyme Q10 treatments attenuate cognitive impairment associated with model of transient global brain ischemia in artificially aged mice. Front Cell Neurosci. 2019;13:74.
- Salehpour F, Hamblin MR, DiDuro JO. Rapid reversal of cognitive decline, olfactory dysfunction, and quality of life using multi-modality photobiomodulation therapy: case report. Photobiomodul Photomed Laser Surg. 2019;37(3):159–167.
- Zhang J, Yue X, Luo H, et al. Illumination with 630 nm red light reduces oxidative stress and restores memory by photo-activating catalase and formaldehyde dehydrogenase in SAMP8 mice. Antioxid Redox Signal. 2019 Apr 10;30(11):1432–1449.
- Fei X, Zhang Y, Mei Y, et al. Degradation of FA reduces abeta neurotoxicity and Alzheimer-related phenotypes. Mol Psychiatry. 2020 Dec 16;26(10). 10.1038/s41380-020-00929-7
- Luan X, Pan Y, Gao Y, et al. Recent near-infrared light-activated nanomedicine toward precision cancer therapy. J Mater Chem B. 2021 Sep 15;9(35):7076–7099.
- Wang C, Dai C, Hu Z, et al. Photonic cancer nanomedicine using the near infrared-II biowindow enabled by biocompatible titanium nitride nanoplatforms. Nanoscale Horiz. 2019 Mar 1;4(2):415–425.
- Xiong H, Li X, Kang P, et al. Near-infrared light triggered-release in deep brain regions using ultra-photosensitive nanovesicles. Angew Chem Int Ed Engl. 2020 May 25;59(22):8608–8615.
- Black CE, Zhou E, DeAngelo C, et al. Cyanine nanocage activated by near-IR light for the targeted delivery of cyclosporine A to traumatic brain injury sites. Mol Pharm. 2020 Dec 7;17(12):4499–4509.
- Farokhi M, Mottaghitalab F, Saeb MR, et al. Functionalized theranostic nanocarriers with bio-inspired polydopamine for tumor imaging and chemo-photothermal therapy. J Control Release. 2019 Sep 10;309:203–219.
- Ragelle H, Danhier F, Préat V, et al. Nanoparticle-based drug delivery systems: a commercial and regulatory outlook as the field matures. Expert Opin Drug Deliv. 2017;14(7):851–864.
- Bhavna MS, Ali M, Baboota S, et al. Preparation, characterization, in vivo biodistribution and pharmacokinetic studies of donepezil-loaded PLGA nanoparticles for brain targeting. Drug Dev Ind Pharm. 2014;40(2):278–287.
- Peer D, Karp JM, Hong S, et al. Nanocarriers as an emerging platform for cancer therapy. Nat Nanotechnol. 2007;2(12):751–760.
- Rip J, Schenk GJ, de Boer AG. Differential receptor-mediated drug targeting to the diseased brain. Expert Opin Drug Deliv. 2009 Mar;6(3):227–237.
- Hrabetova S, Cognet L, Rusakov DA, et al. Unveiling the extracellular space of the brain: from super-resolved microstructure to in vivo function. J Neurosci. 2018 Oct 31;38(44):9355–9363.
- Salamanna F, Gambardella A, Contartese D, et al. Nano-based biomaterials as drug delivery systems against osteoporosis: a systematic review of preclinical and clinical evidence. Nanomaterials (Basel). 2021 Feb 19;11(2). 10.3390/nano11020530