ABSTRACT
Introduction
Cancer-related drug expenses are rising with the increasing cancer incidence and cost may represent a severe challenge for drug access for patients with cancer. Consequently, strategies for increasing therapeutic efficacy of already available drugs may be essential for the future health-care system.
Areas covered
In this review, we have investigated the potential for the use of platelets as drug-delivery systems. We searched PubMed and Google Scholar to identify relevant papers written in English and published up to January 2023. Papers were included at the authors’ discretion to reflect an overview of state of the art.
Expert opinion
It is known that cancer cells interact with platelets to gain functional advantages including immune evasion and metastasis development. This platelet-cancer interaction has been the inspiration for numerous platelet-based drug delivery systems using either drug-loaded or drug-bound platelets, or platelet membrane-containing hybrid vesicles combining platelet membranes with synthetic nanocarriers. Compared to treatment with free drug or synthetic drug vectors, these strategies may improve pharmacokinetics and selective cancer cell targeting. There are multiple studies showing improved therapeutic efficacy using animal models, however, no platelet-based drug delivery systems have been tested in humans, meaning the clinical relevance of this technology remains uncertain.
1. Introduction
Cancer is a major public health burden, with global death rates parallel to cardiovascular disease [Citation1]. However, the incidence of cancer is rapidly increasing, with a predicted doubling in 2070 from 2020 [Citation2]. Although improved strategies for cancer prevention and the development of new methods for early detection are essential, more efficient medical treatments for advanced cancer are necessary to keep mortality rates low. However, as the pre-launch research and development costs for new anticancer drugs may reach several billion US dollars [Citation3], it is unsurprising that the rising cost of cancer drugs leads to treatment abandonment due to affordability, especially in low-income countries [Citation4]. Thus, a low-cost strategy to increase the efficiency of already available anticancer drugs is intriguing and necessary for future healthcare systems. In this review, we discuss the potential of platelets as a drug delivery system to serve this purpose.
2. Platelet biology
Platelets were first recognized as critical contributors to hemostasis in the late 19th century [Citation5]. Later, that platelets exhibit many other functions, with essential roles in tissue regeneration, immunology, and cancer biology became apparent [Citation6–11]. Platelets are anucleated membrane cell fragments derived from megakaryocytes (MKs). Thus, platelets are considerably smaller than other nucleated blood cells, typically with a diameter of 2–3 µm and a height of 350–800 nm [Citation12]. MKs originate partly from a shared progenitor with erythroid cells; however, earlier restricted progenitors have also been identified [Citation13–16]. MK development is primed by thrombopoietin (TPO) [Citation17]. However, at least some TPO-independent platelet production exists, as double knockout of the Tpo gene and TPO-receptor gene Mpl does not entirely abrogate thrombopoiesis in mice, which can also be salvaged with different chemokines [Citation18]. MKs usually reside close to blood vessels [Citation19], and shear stress promotes MK maturation [Citation20]. Under normal conditions, platelets are produced by membrane budding or proplatelet formation [Citation21,Citation22]. However, upon greater need, MK rupture induced by interleukin-1α may be an emergency mechanism to restore the platelet pool [Citation22].
Although platelets are anucleate cell fragments with a rapid mRNA time decay [Citation23], limited protein synthesis persists [Citation24]. However, most platelet proteins are inherited by parental MKs, and a recent analysis of the platelet proteome identified over 5,000 unique proteins [Citation25]. Some proteins are packed in granules, mainly alpha granules and dense granules, which are released upon platelet activation, yielding a complex releasate [Citation26]. Furthermore, the composition of the platelet releasate differs depending on the activation stimuli [Citation27,Citation28]. Platelet alpha granules contain several hundred proteins, including growth factors and chemokines [Citation29,Citation30]. Conversely, dense granules primarily contain small coagulation-active substances such as ADP, serotonin, and calcium. However, mass spectrometry analysis has also revealed a minor selection of cell signaling proteins [Citation31].
As discovered by Heijnen et al., platelets secrete different microvesicles up to 1 µm in diameter via plasma membrane shedding or multivesicular body and alpha granule exocytosis following thrombin receptor agonist peptide or thrombin stimulation [Citation32]. Nowadays, the terms ‘microvesicles’ or ‘microparticles’ are generally replaced with ‘extracellular vesicles’ (EVs) and denominated small, medium, or large, depending on size [Citation33]. Platelet extracellular vesicles (PEVs) are the most abundant EVs in plasma, with substantial contributions from other blood and endothelial cells [Citation34,Citation35]. Although PEVs are generally ‘small,’ with a diameter <200 nm depending on origin [Citation36,Citation37], larger PEVs exist and can contain large organelles such as mitochondria and proteasomes [Citation38]. PEVs are shed by platelets upon storage or activation by different stimuli [Citation36,Citation37,Citation39]. Regarding coagulation, PEV generation follows membrane ballooning, which both increase the total procoagulant surface area [Citation40,Citation41]. Different platelet activators yield quantitative and qualitative differences in the PEV composition, suggesting that formation and packing involve active rather than stochastic processes [Citation42]. Consequently, PEVs generated in cancer may have specific qualities, as the proteome of PEVs generated by the co-incubation of platelets with the breast cancer cell line MDA-MB-231 differs from that of thrombin-generated PEVs [Citation43].
3. The role of platelets in cancer
Patients with cancer have an increased risk of venous thromboembolism (VTE), which varies with cancer type and stage [Citation44,Citation45]. Additionally, an elevated platelet count is often observed and is generally indicative of poor prognosis [Citation46–48]. However, the increased VTE risk in patients with cancer is not merely a result of elevated platelet counts, as there is no association between VTE and elevated platelet counts in the general population [Citation49]. This phenomenona is surely multifactorial and likely involves a complex interplay between platelets and cancer cells [Citation50]. It is known that the tumor microenvironment attracts platelets, as immunohistochemical investigations of tumor biopsies reveal that platelets can infiltrate tumors [Citation51,Citation52] (). However, platelets are generally absent in normal tissues. Based on findings from murine knockout models, platelet tumor infiltration may be dependent on select G proteins [Citation53], meaning extravasation is not a random event. In addition, platelet tumor infiltration leads to worse overall survival in patients with colorectal or pancreatic cancer [Citation51,Citation54,Citation55]. Thus, one can surmise a critical role of platelet-cancer interactions in cancer biology.
Figure 1. Platelet-cancer interactions. The tumor microenvironment attracts platelets followed by binding and activation of the platelets by cancer cells. Platelets may be activated through multiple pathways including secretion of soluble mediators or direct receptor stimulation yielding functional advantages for the cancer cells.
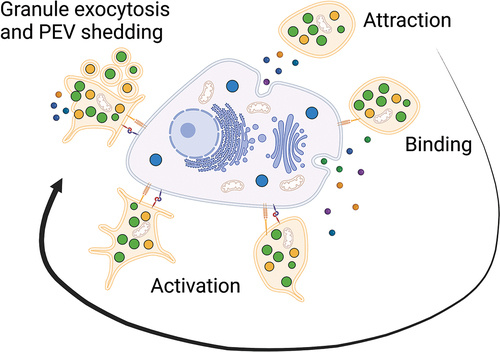
3.1. Platelet-cancer interactions
Cancer cells activate platelets via multiple pathways. For instance, the high mobility group box 1 protein is released by cancer cells and activates platelets via Toll-like receptor 4 (TLR4) [Citation56]. Multiple cell lines likely generate the strong platelet activator thrombin via different mechanisms, with varying dependency on tissue factor [Citation57]. Cancer cells can also activate platelets directly via binding to receptors and adhesion molecules such as Clec-2, P-selectin, glycoprotein (GP) VI, integrin α6β1, and platelet FcγRIIa [Citation58–63]. Furthermore, cancer cells secrete ADP, a weak platelet activator [Citation64]. Treatment with the commonly used platelet inhibitor ticagrelor, which inhibits the ADP receptors P2Y12 and P2Y1, reduced tumor growth in ovarian cancer-bearing mice by 60% compared to aspirin and 75% compared to placebo [Citation65]. Similar results have been obtained using ADP receptor inhibitors in melanoma, breast cancer, and pancreatic cancer models, underscoring the importance of platelet ADP stimulation by cancer cells [Citation66,Citation67].
There was early enthusiasm about the potential of aspirin treatment to break the platelet-cancer axis, and observational studies have shown a reduced risk of multiple cancer diseases and better cancer-specific survival in patients with colorectal cancer [Citation68–71]. However, a recent systematic review including more randomized controlled trials (RCTs) for prophylactic aspirin against colorectal cancer did not confirm these previous conclusions [Citation72]. Nonetheless, the authors reported high statistical heterogeneity due to variable study designs, including aspirin dosage and treatment duration. Multiple platelet inhibitors are in clinical use, but the studies on the relationship between platelet inhibition and cancer risk or mortality generally includes only aspirin. Although some data on the effects of other platelet inhibitors, usually in combination with aspirin, are available, RCTs are lacking [Citation73].
Platelets interact with circulating tumor cells (CTCs) not only by binding to single cells, but also by formation of larger aggregates and both mechanisms may confer functional advantages to cancer cells, including protection against mechanical forces and CTC arrest [Citation74–76]. This interaction also facilitates epithelial-to-mesenchymal transition (EMT) through transforming growth factor (TGF)-β1 secretion [Citation58,Citation77]. In SK-OV-3 and OVCAR-3 ovarian cancer cells, inhibiting the TGF-β1 receptor reversed the effect of platelets on EMT, thereby reducing metastatic potential [Citation78].
3.2. Platelets in cancer immunology
Cancer cells can evade natural killer (NK) and T cells by interacting with platelets (). Platelets express MHC class 1 molecules (MHC-1), which exhibit time-dependent decay [Citation79]; however, MHC-1 expression is upregulated upon activation [Citation38]. Thus, platelet binding can lead to pseudo-expression in CTCs, disrupting recognition by NK cells, and directly impairing their cytotoxic function [Citation80]. However, platelet binding may also exert MHC-1-independent inhibitory functions [Citation81]. The use of platelet releasate weakened NK cytotoxicity against tumor cells by downregulating the NK group 2D (NKG2D) receptor expression, and this effect was abolished when neutralizing TGF-β1 [Citation82]. Similar findings have been reported using PEVs co-cultured with NK cells, where activation receptors were downregulated in a TGF-β1-dependent manner [Citation83]. Platelet binding may also cause the shedding of NKG2D receptor ligands on cancer cells, thus impairing NK cell-mediated lysis [Citation84].
Figure 2. Platelet-assisted immune evasion. Platelet-cancer interactions assist cancer cells in evading NK and T cell cytotoxicity partially through TGF-ß-mediated mechanisms. In addition, binding of platelets also leads to pseudo-expression of MHC-1 and PD-L1, thus cancer cells may avoid recognition by NK cells through expression of platelet self-antigens and impair T cell cytotoxicity through checkpoint inhibition.
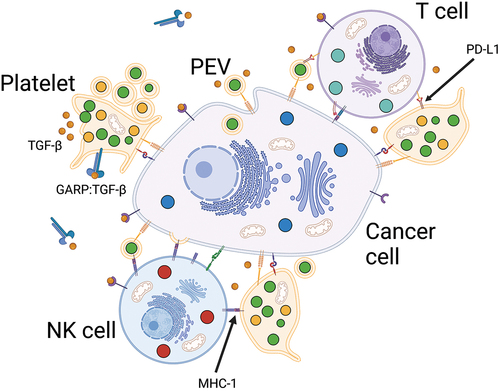
T cells activate platelets through the CD40-CD40L axis, which may increase T cell recruitment through C-C motif chemokine ligand 5 secretion [Citation85]. Furthermore, in lung cancer, the frequency of platelet-T cell aggregates increases [Citation86]. Although platelets can also activate cytotoxic T cells through antigen presentation [Citation38,Citation87], platelet-T cell interactions are likely detrimental in cancer because the surface-bound GARP-TGF-β complex on platelets is a crucial mediator of platelet-associated T cell suppression in murine cancer models [Citation88,Citation89]. In addition, similar to the pseudo-transfer of MHC-1, platelet binding may lead to the pseudo-expression of programmed death-ligand 1 (PD-L1), thus impairing anticancer T-cell activity through checkpoint inhibition [Citation90]. Riesenberg et al. studied the potential interplay between platelets and the tumor microenvironment in heat shock protein gp96 knockout mice, which exhibit thrombocytopenia and severe platelet dysfunction [Citation91]. SMAD3 phosphorylation was lower in tumor cells and tumor-infiltrating lymphocytes (TILs), indicating less stimulation by TGF-β. Moreover, the relative CD4+ or CD8+ TIL frequencies, PD-1 expression, and tumor necrosis factor (TNF)-α and interferon (INF)-γ production upon stimulation were higher. The tumor growth-inhibitory effect of platelet inhibition described in the previous sections was also confirmed and it was abrogated when combined with an antibody against CD8.
Bispecific T cell-recruiting antibodies are a novel modality of immunotherapy in which monoclonal antibodies (mAbs) connect cancer cells with T cells. Although only a few drugs have reached clinical use, this technology has shown promising therapeutic efficacy, and multiple drugs are under clinical investigation [Citation92]. However, recent evidence indicates that activated platelets may reduce bispecific antibody-mediated CD4+ and CD8+ T cell reactivity, which is restored with TGF-ß blockade [Citation93]. Platelets may also play a role in the resistance to conventional anti-PD-1-based immunotherapy. The combination of dual platelet inhibition and anti-PD-1 antibody was more effective than anti-PD-1 antibody treatment alone in a murine MC-38 colon cancer model [Citation91]. Although the platelet-mediated anticancer effect of aspirin in humans is controversial, one prospective study showed that aspirin only reduced the risk of colorectal cancer with a low TIL count, suggesting a possible immune-mediated pro-tumor effect of platelets [Citation94]. Thus, our knowledge of the interactions between platelets and cytotoxic NK and T cells supports new studies on platelet inhibition to overcome resistance to various immunotherapies.
4. Drug delivery systems
Drug delivery systems exist in many forms, including novel techniques using drug-loaded nanoparticles [Citation95]. Liposomes are likely the best-known and most used of these platforms, and several drugs with liposomal formulations have been approved since the 1990s [Citation96]. Liposomes are membrane-permeable lipid bilayer vesicles with aqueous cores that can be loaded with drugs to alter their pharmacokinetics and pharmacodynamics [Citation96]. Other notable platforms include poly(lactic-co-glycolic acid) (PLGA) and protein-based nanoparticles. PLGA nanoparticles are manufactured from polymers comprising lactic and glycolic acid in different ratios to obtain specific physicochemical properties [Citation97]. Protein-based nanoparticles have huge potential as they can be manufactured with various functionalities and responsiveness depending on the protein composition [Citation98]. However, their clinical use remains limited. Despite their early promise, nanopharmaceuticals have disadvantages, most notably cost and certain immunological phenomena [Citation99]. Liposomes are cleared by macrophages in the reticuloendothelial system [Citation100], and macrophage uptake of liposomes depends on their size and lipid composition [Citation101]. Pegylation is used to alter the physicochemical properties of liposomes and increase their stability [Citation102]. However, this leads to the risk of hypersensitivity reactions, possibly through interactions with anti-PEG IgM and complement activation [Citation103].
Generally, drug delivery systems are used to optimize the pharmacokinetics and pharmacodynamics of a drug, thus improving its therapeutic efficacy and safety. However, promising preclinical data on liposome-formulated chemotherapeutics usually do not translate into substantially better therapeutic efficacy in clinical studies [Citation104]. However, a liposomal formulation may be more beneficial than the free drug if it reduces the frequency or severity of adverse events. Doxorubicin (DOX) is an anthracycline frequently used to treat solid and hematological malignancies as a liposomal formulation and a free drug. Thus, as much clinical data are available, DOX is ideal for comparing toxicity. However, systematic reviews generally conclude that conventional and liposomal formulations have similar toxicity profiles. Although the risk of cardiotoxicity may be reduced in liposomal formulations, the risk of hand-foot syndrome increases [Citation105–107].
One example of the cost issue in developing advanced drug delivery systems for existing drugs is CPX-351, now registered as a Vyxeos liposomal. CPX-351 is a relatively new liposome-encapsulated formulation of cytarabine and daunorubicin that maintains a favorable molar ratio of 5:1 in the plasma and bone marrow (BM) for a prolonged time [Citation108]. In addition to its favorable pharmacokinetics, preclinical data suggest that it may selectively target leukemic cells over normal hematopoietic progenitors [Citation109,Citation110]. A phase III trial published in 2018 showed that CPX-351 markedly improved overall survival and remission rates compared with conventional treatment, with similar toxicity in older patients with newly diagnosed high-risk secondary acute myeloid leukemia [Citation111]. Post hoc analysis also showed improved quality-adjusted time without symptoms of disease or toxicity (Q-TWiST) [Citation112]. However, a 2021 analysis concluded that it was far from cost-effective at the current price, yielding an incremental cost of $115,000 per patient [Citation113]. Thus, even if drug delivery platforms have been in clinical use for a long time, they still have several shortcomings, including the failure to increase therapeutic efficacy or to substantially reduce toxic side effects, at least in a cost-effective manner.
5. Platelets as a drug delivery system
Cancer cells interact with platelets to gain functional advantages. Thus, using platelets for drug delivery could provide a platform for selectively targeting cancer cells, at least to a greater degree than free drugs or existing drug delivery systems. Some apparent advantages of a platelet-based versus a synthetic drug delivery system are that platelet concentrates are readily available, well tolerated, and have relatively low direct production costs [Citation114]. Although it would increase the hospital stay for patients with cancer, platelets for drug delivery can also potentially be harvested from the patients themselves, thus eliminating potential issues with alloimmunization and further minimizing the risk of transfusion reactions.
Platelets or PEVs as drug delivery systems also share the theoretical benefits of nanopharmaceuticals in terms of changes in pharmacokinetics, as the drugs are encapsulated and protected from degradation processes in the blood, phagocytosis by macrophages, and renal clearance. PEVs are also known to infiltrate tumors, lymphoid organs, BM, and inflamed tissue [Citation38,Citation115–117]. Platelets can transfer content to various malignant and normal cells via PEV shedding and subsequent internalization by the target cells [Citation115,Citation118–122]. Thus, PEVs shed from drug-loaded platelets may transfer molecules independent of specific transporter proteins, thereby circumventing limiting drug uptake, which is a suggested drug-resistance mechanism [Citation123].
Strategies for using platelets as drug delivery systems can be summarized into three main categories: drug-loaded, drug-bound, and platelet-based hybrid vesicles ().
Figure 3. Platelets as drug-delivery systems. Platelets can be used as drug-delivery systems mainly through three different strategies. a) by drug-loading where platelets encapsulate the drug, then accumulate in the tumor microenvironment followed by activation and drug release. b) by drug-binding where large molecules such as antibodies or cytotoxic complexes are conjugated to the surface and kill cancer cells by cell-to-cell contact or by shedding of PEVs. c) by implementing platelet membranes in hybrid vesicles through fusing of PEVs with synthetic drug-loaded nanocarriers or other cell membranes to slow macrophage clearance and increase accumulation in the tumor microenvironment.
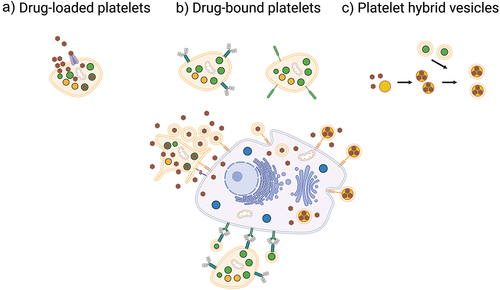
5.1. Drug-loaded platelets
Several studies have used platelets as a delivery system by loading cytotoxic compounds and the most commonly used drug is DOX [Citation124–129]. However, other chemotherapeutics have also been tested in use with platelet hybrid vesicles [Citation130,Citation131]. Because anthracyclines passively permeate lipid membranes, drug loading is uncomplicated [Citation132]; however, human organic cation transporter 1 (OCT1; SLC22A1)-mediated transport has also been reported [Citation133]. Administering DOX-loaded platelets increased drug uptake and apoptosis induction in Raji cells compared with free DOX [Citation124]. Furthermore, toxicity in cardiomyocytes was reduced, which may have clinical relevance, as cardiomyopathy is a feared consequence of DOX treatment. Platelets have migratory capabilities [Citation134], which were further enhanced by coating DOX-loaded platelets with polydopamine to increase tumor infiltration and drug transfer in MCF-7 breast cancer-bearing mice upon irradiation with near-infrared light [Citation129]. One possible challenge of using platelets as drug vectors is their limited storage potential. Wu et al. used cryopreserved DOX-loaded platelets from clinical-grade platelet concentrates to study apoptosis induction in cell-lines from different solid cancers, creating an ‘off-the-shelf product’ [Citation126]. Cryopreservation of DOX-loaded platelets did not markedly impair platelet function or the cytotoxic effect, which was generally in line with that of free DOX, although it was higher than that of liposomal DOX. Moreover, drug release, although partially a time-dependent and pH-sensitive process, was stimulated by tissue factor-expressing cancer-derived EVs.
Platelets loaded with the tyrosine kinase inhibitors sorafenib and lenvatinib led to increased drug uptake and necrosis in tumors in hepatocellular carcinoma-bearing rats compared to treatment with free drugs [Citation135]. Furthermore, drug release increased when the platelets were exposed to ADP, indicating the importance of platelet activation. The contribution of platelet activation to loaded drug release has also been corroborated in other studies [Citation127,Citation129]. Li et al. suggested in their study that the polydopamine-coated platelets could bind DOX through π–π stacking, which was released in weak acidic environments [Citation129]. In addition, the transfer of drugs from DOX-loaded platelets decreased by inhibiting endocytosis in the target cancer cells. Thus, drugs are partially transferred from drug-loaded platelets by shedding PEVs, followed by internalization by cancer cells.
Photothermal therapy (PTT) is a treatment modality closely related to the better-known photodynamic therapy. In PTT, photothermal agents (PTAs) may be administered via intratumoral or intravenous routes before laser treatment, which induces heating, followed by apoptosis or necroptosis [Citation136]. Thus, tumor-selective uptake is necessary to avoid excessive damage to the surrounding tissue, which currently limits its application outside preclinical research. Platelets were used as vectors for gold nanostar (AuNS)-directed PTT in a murine head and neck squamous cell carcinoma model [Citation137]. Platelet cloaking protected the AuNSs against macrophage clearance, yielded better therapeutic efficacy, and led to less weight loss than treatment with uncoated AuNSs. Combining PTT with the loading of cytotoxic compounds may also be prudent. By loading platelets with the PTA IR-820 and DOX before laser irradiation, Zhang et al. obtained synergistic effects, as heat induction increased DOX release, thus improving anticancer efficacy in breast cancer-bearing mice [Citation138].
5.2. Drug-bound platelets
Other studies have investigated platelets as drug delivery systems with antibody conjugates or binding of other large molecules. This may also be useful for increasing cancer cell targeting because low platelet binding to cancer cells may be a potential limitation of using platelets as drug vectors. Transferrin conjugation increased platelet adherence to myeloma cells with high transferrin receptor expression [Citation139]. Furthermore, the anticancer effect of DOX-loaded platelets was optimized by conjugating anti-CD22 to the platelets, leading to more selective drug transfer and increased DOX uptake and apoptosis of CD22+ lymphoma cells [Citation140]. Fan et al. conjugated platelets with a tumor microenvironment-responsive nanogel comprising the pore-forming peptide GALA and serine protease granzyme B (GrB) [Citation141]. Platelets accumulated at the tumor site, where the GALA/GrB complex was cleaved under acidic conditions and internalized by cancer cells. GALA was essential for lysosomal escape by GrB for optimal apoptosis induction in a murine melanoma model. Yap et al. used single-chain antibodies against activated GP IIb/IIIa linked to the microtubule inhibitor monomethyl auristatin E (MMAE) [Citation142]. Thus, the activated platelets were selectively targeted for drug delivery in vivo, and following tumor infiltration, MMAE was released via linker cleavage by cathepsin B. This system effectively treated primary breast cancer tumors and metastasis in murine models and showed excellent selectivity with no drug uptake in the BM or spleen. Li et al. engineered TNF-related apoptosis-inducing ligand (TRAIL)-expressing platelets by lentiviral transduction and transplantation of murine hematopoietic stem cells (HSCs) [Citation143]. These platelets produced an anticancer effect in a murine metastasis model using the human prostate cancer cell line, PC3. Likewise, TRAIL- and von Willebrand factor-coated liposomes have effectively killed CTCs isolated from patients with cancer with metastatic disease through interconnection with cancer cells via platelets [Citation144]. Zhao et al. used paclitaxel and IR-780-containing P-selectin-targeting nanoparticles and adopted a similar strategy [Citation145]. The therapeutic efficacy of the drug-containing nanoparticles was further enhanced by PTT, which increased platelet accumulation at the tumor site. This ‘hitchhiking’ strategy was also exploited by Li et al., who used P-selectin-binding nanoparticles loaded with ticagrelor and the anti-inflammatory agent celecoxib to inhibit metastasis in breast cancer-bearing mice through modulation of the tumor microenvironment [Citation146].
Using platelets as a drug delivery system may be a double-edged sword because platelets also have protumoral properties. This effect can be reduced by adding the sulfated polysaccharide fucoidan to the system. Guo et al. used micelles containing fucoidan and DOX that could bind to platelets through P-selectin, thus targeting platelets in vivo that are further connected to cancer cells [Citation147]. The addition of fucoidan, when compared to dextran containing (control) micelles or free DOX, further decreased tumor volume in a murine 4T1 breast cancer metastasis model, possibly through reduction of tumor TGF-ß levels leading to a favorable immune response with increased levels of pro-inflammatory cells and decreased levels of anti-inflammatory cells.
5.2.1. Platelet-based immunotherapy
The introduction of checkpoint inhibitors represents a paradigm shift in treating solid cancers, resulting in durable remission in previously untreatable diseases. Briefly, this treatment modality seeks to disrupt checkpoint signaling in T cells to stimulate an anticancer immune response. Recently, many studies have used platelets as mAb carriers to optimize this technology further.
Wang et al. developed a drug delivery system in which antibodies against programmed death-1 protein (PD-1) were conjugated to platelets for use in melanoma and breast cancer models [Citation148]. This system uses the ability of platelets to infiltrate tumors and then release the drug in situ by shedding PEVs. Anti-PD-1-conjugated platelets were superior to free anti-PD-1 treatment, increasing the circulation half-life and yielding a stronger local anticancer immune response and tumor reduction. Lu et al. used a different approach to link tenfold the amount of anti-PD-1 antibody per platelet compared to the previous study [Citation149]. This was managed by conjugating antibody-crosslinked nanogel backpacks that dissolved in response to the reduction activity of the platelet surface upon platelet activation. Furthermore, Hu et al. used anti-PD-1-conjugated platelets to treat acute myeloid leukemia (AML)-bearing mice by conjugating platelets to HSCs [Citation150]. HSC-conjugation was necessary for sufficient BM infiltration; however, adding platelets to the system substantially increased the anti-leukemic inflammatory response and survival. This study thus illustrates insufficient BM infiltration as a potential weakness of unmodified platelets as a drug delivery system. The same group combined anti-PD-1-conjugated platelets with chimeric antigen receptor (CAR)-T cells in a biodegradable hydrogel for implantation into the tumor resection cavity of melanoma-bearing mice [Citation151]. Anti-PD-1 antibodies are released upon activation in situ, increasing the efficacy of CAR-T cell treatment. Zhang et al. engineered murine PD-1 expressing platelets from MK progenitors that accumulated in partially resected malignant melanoma tumor beds [Citation152]. The authors observed an anticancer effect by increasing tumor-infiltrating cytotoxic CD8+ T cells, as observed in the previous studies with anti-PD-1-conjugated platelets. Moreover, the anticancer effect was increased when PD-1-expressing platelets were loaded with cyclophosphamide to deplete the tumor microenvironment of regulatory T cells.
The inflammatory anticancer effect of anti-PD-1-conjugated platelets was also potentiated by incorporating them into a hydrogel loaded with the tyrosine kinase inhibitor pexidartinib [Citation153]. This incorporation depleted the resection sites of tumor-associated macrophages in tumor-bearing mice with different cancer types by blocking CSF1R signaling. Furthermore, concomitant treatment with vadimezan increases the recruitment of anti-PD-1-conjugated platelets through tumor vascular disruption, resulting in substantially improved survival in several murine cancer models [Citation154]. Lastly, PTT has been combined with anti-PD-1-conjugated platelets to enhance the immune stimulatory effect through ‘wounding’ of the tumor to increase platelet infiltration and tumor antigen release [Citation155,Citation156].
Thus, platelets may not only play a role in developing resistance to different immunotherapies, they can also be engineered into potent immunomodulatory anticancer cell fragments using existing drugs.
5.3. Platelet hybrid vesicles
Several researchers have developed platelet-inspired synthetic hybrid vesicles. Possible benefits of implementing platelet proteins or membrane elements in nanocarriers include improved cancer-specific targeting and slowed clearance by macrophages. PLGA nanoparticles cloaked with platelet membranes mimic several aspects of platelets, including platelet antigen expression, collagen binding, and endothelial cell binding [Citation157]. Macrophage phagocytosis in vitro was also inhibited in a CD47-specific manner. Wang et al. used this method by coating chitosan-modified PLGA nanoparticles loaded with the anticancer drug bufalin with PEVs to increase cancer cell apoptosis without excessive off-target effects [Citation158]. The tumor infiltration and anticancer effects of platelet-coated hybrid vesicles loaded with docetaxel were further enhanced by incorporating them with recombinant VAR2CSA [Citation159]. This peptide can attach to chondroitin sulfate expressed on melanoma cells with different phenotypes after EMT and mesenchymal-to-epithelial transition, thus increasing platelet recruitment in both primary tumors and metastases. Combining the vascular-disrupting agent vadimezan with anti-PD-1-conjugated platelets may increase tumor platelet accumulation and therapeutic efficacy [Citation154], as described in the previous sections. Similar effects were observed when vadimezan was combined with paclitaxel-loaded platelet membrane-coated nanocrystals in breast cancer-bearing mice [Citation160]. Pan et al. coated DOX-loaded liposomes with ligands to mimic platelet-tumor binding and increased apoptosis induction in co-cultures with breast cancer cell lines [Citation161]. In addition to the drug-loading strategy, conjugating platelet membrane-coated silica particles with the death receptor ligand TRAIL has shown potential for treating murine breast cancer metastatic disease [Citation162]. Consequently, combining TRAIL expression and DOX loading in platelet hybrid vesicles may provide additional therapeutic benefits [Citation163].
Kim et al. used red blood cells and platelet membranes to coat AuNSs with hybrid vesicles, which were then loaded with curcumin [Citation164]. Thus, AuNS-directed PTT was combined with a cytotoxic drug. Platelet membranes were integrated for the selective targeting of cancer cells, and adding red blood cell membranes proved crucial for further evasion of macrophage phagocytosis. Coating PTA-containing hybrid vesicles with platelet membranes may increase tumor uptake and penetration [Citation165]. Multiple studies have shown an increased in vivo anticancer efficacy in breast cancer-bearing mice [Citation165–167]. Similar to the beneficial effects of platelet coating in PTT systems, coating gold nanocages with PEVs and loading them with cisplatin has been shown to sensitize breast cancer-bearing mice to low-dose radiotherapy [Citation168].
5.3.1. Platelet hybrid vesicle-based immunotherapy
Platelet hybrid vesicles have been manufactured to induce favorable immune responses in the tumor microenvironment. A variation of this method was developed by Yu et al. in which vesicles from genetically engineered HEK-293T cells and platelets were fused and loaded with oxaliplatin [Citation130]. HEK-293T cells were infected with the gene encoding the T cell immunoreceptor with immunoglobulin and the ITIM domain (TIGIT), which binds to the CD155 antigen on cancer cells. Thus, the hybrid vesicles blocked TIGIT-mediated immune evasion and restored CD8+ T cell activity. In a recent study, Bahmani et al. coated nanoparticles containing the TLR agonist resiquimod with platelet membranes, which yielded promising efficacy, especially in MC-38 tumor-bearing mice, where all tumors were eradicated even after two tumor rechallenges compared to the 28.6% survival rate of treatment with free and naked nanoparticle-encapsulated drugs [Citation169]. This superior therapeutic efficacy was attributed to enhanced immune activation and increased T-cell infiltration of the tumor.
5.3.2. Combinatory platelet hybrid vesicle platforms
Platelets or platelet hybrid vesicles have been used in drug delivery systems to increase selective uptake by cancer cells. However, there are numerous strategies for further optimizing tumor infiltration, thereby increasing the therapeutic efficacy of platelet-inspired drug delivery. Hu et al. developed a double-hit relay system [Citation131]. First, a nanogel encapsulating TNF-α, decorated with an Arg-Gly-Asp peptide, was administered to increase tumor vascular inflammation in breast cancer-bearing mice. Then, platelet-membrane-coated dextran nanocarriers loaded with paclitaxel were administered to induce apoptosis. Paclitaxel uptake and therapeutic efficacy considerably increased when tumor vascular inflammation was concomitantly induced. Multiple myeloma (MM) is a hematological malignancy usually confined to the BM. Thus, linking the bisphosphonate alendronate, which can bind to hydroxyapatite in bones, to platelet membrane-cloaked bortezomib-loaded nanocarriers increases tumor uptake and improves the survival of MM-bearing mice [Citation170]. Zhou et al. used metal-organic nanostructures loaded with α-methyl-DL-tryptophan to block glutamine uptake in the breast cancer cell line ZR-75-1 [Citation171]. After drug loading, the nanocarriers were coated with MnO2 to prevent drug leakage and increase their glutathione scavenging properties, followed by loading into platelets. This combination resulted in glutamine deprivation and increased reactive oxygen species levels in cancer cells owing to reduced glutathione levels. Treatment was supplemented with ultrasonography of the tumors, causing the drug-loaded platelets to release the nanocarriers and to aggregate and form thrombi, yielding synergistic anticancer effects.
In addition, multiple studies have investigated combinations of cytotoxic drugs, death ligands, adhesion molecules, antibodies, and some variants of tumor disruption in either platelet or platelet hybrid vesicle-based drug delivery systems to optimize these platforms, as described in the previous sections and summarized in .
Figure 4. Strategies to optimize platelet-based drug-delivery systems. In order to enhance therapeutic efficacy, drug-loaded platelets, drug-bound platelets, or platelet hybrid vesicles can be conjugated with attachment molecules to increase retention in tumors a). Platelet infiltration of tumors may also be increased through combination with PTT b), tumor vascular-disrupting agents c), and tumor-inflammation increasing agents d). NIR, near infrared.
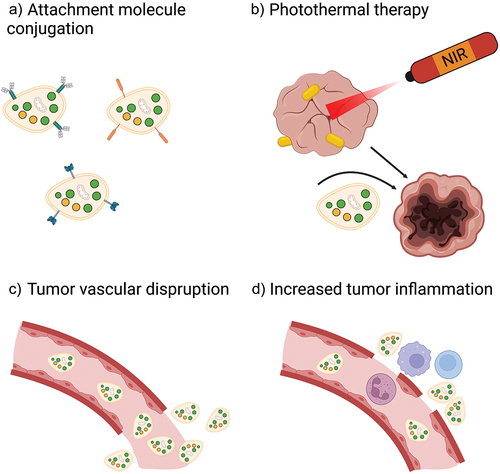
6. Challenges and future perspectives
Using platelets for drug delivery faces several challenges. First, platelets must be loaded with drugs. Larger molecules that cannot cross the lipid bilayer plasma membrane, such as antibodies or complex receptor ligands, generally need to be attached to the plasma membrane, as described in the previous sections. However, some evidence indicates that platelets can internalize mAbs and release them upon activation [Citation172]. Smaller molecules can permeate the platelet membrane if they are sufficiently lipophilic. DOX is a relatively small drug with a theoretical diameter of 1.5 nm [Citation173], equal to the inner diameter of the large aquaporin 4 [Citation174]. Thus, diffusion across channels and pores for drugs is not likely an important mechanism; however, this field has been poorly investigated. Consequently, the influx of hydrophilic drugs might require transporter proteins or endocytosis. Girardi et al. used a systematic solute carrier (SLC)-focused CRISPR/Cas9 library to screen the cellular uptake of 60 commonly used cytotoxic drugs and found that 47 were functionally dependent on one or more SLC transporters [Citation175]. Nucleoside analogs such as decitabine and azacitidine are frequently used to treat myelodysplastic syndrome and AML. These hydrophilic drugs are transported by human equilibrative nucleoside transporters (hENTs) or human concentrative nucleoside transporters (hCNTs) [Citation176–178]. Although hCNTs have not been identified in platelets, hENT1 (SLC29A1) has been [Citation25]. hENT1 also is an important transporter for the nucleoside analog cytarabine, a mainstay chemotherapeutic agent in AML, and its expression levels correlate with the survival of patients with AML receiving intensive chemotherapy [Citation179]. Various single-nucleotide polymorphisms in the gene encoding the hENT1 protein may also affect the treatment response [Citation180]. Likewise, protein expression correlates with survival after treatment with gemcitabine, another nucleoside analog, in biliary tract and pancreatic cancers [Citation181,Citation182]. Moreover, recent research has identified another SLC-transporter, OCTN1 (SLC22A4), as a potent transporter for several nucleoside analogs, and its expression levels, which are dependent on DNA methylation [Citation183], correlated independently with survival in patients with AML receiving intensive chemotherapy [Citation184]. Numerous potential drug transporters exist within the SLC family of proteins, such as OCT1 and OCTN1, which are not found in the platelet proteome [Citation25,Citation185]. Therefore, the ability of platelets to load hydrophilic drugs may be limited.
Although sonication, electroporation, and extrusion can facilitate drug loading of PEVs [Citation157,Citation158,Citation166,Citation168,Citation186], these strategies may not be feasible for whole platelets. Using electroporation, Rao et al. loaded platelets with relatively large AuNSs, approximately 12 nm in diameter and 50 nm in length [Citation137]. The electroporated platelets could hold the AuNSs for up to 48 h in a buffer; however, platelet function, viability, and circulation time were not tested. Although the non-immunological mechanisms for platelet clearance are not entirely understood, platelets are prone to apoptosis through the BCL2 antagonist/killer (BAK) and BCL2-associated X and apoptosis regulator (BAX)-dependent pathways [Citation187]. Furthermore, loss-of-function mutations in B-cell lymphoma extra-large (BCL-XL) or treatment with ABT-737, which antagonizes BCL-XL, shortens the platelet half-life, and ABT-737 even causes acute thrombocytopenia [Citation188]. Thus, the loading procedure should not affect the viability of platelets to function as a drug delivery system.
Platelets have scavenging properties as they can engulf large molecules such as fibrinogen, or even whole bacteria, which then localize in invaginations of the open canalicular system [Citation134]. However, platelets can also phagocytose proteins, process them into peptides, and present them to naïve T cells via MHC-1 [Citation87,Citation189]. Although limited data support the scavenging of nanoparticles for drug-loading, MnO2-coated nanocarriers with an average diameter of 146 nm could be loaded into unperturbed platelets, suggestively through endocytosis, but the exact mechanism was not investigated in detail [Citation171].
Following drug loading or binding, platelets transfer the drugs to target cancer cells, which depends on the ability of platelets or shed PEVs to infiltrate the tumor microenvironment. After loading into platelets or platelet hybrid vesicles, different drugs are released into the cell culture medium or buffer over time, and this release depends on pH [Citation124,Citation126,Citation131,Citation161,Citation170]. However, if a drug is only released passively in the plasma swiftly after infusion, drug carriers will have little specificity, meaning that this should preferably happen close to the target cells. Wu et al. suggested that cancer cell-derived EVs could activate platelets and stimulate the PEV shedding leading to drug release [Citation126]. However, transfused platelets will regardless shed PEVs, but this will comprise only a small fraction of the circulating PEVs. Thus, they may be outcompeted by non-loaded PEVs in vivo unless this mainly occurs in the tumor microenvironment.
Furthermore, much of the present in vivo data on platelets as a drug delivery system are derived from solitary tumor models, quantitative analysis of small metastatic foci, or CTC models. If close contact is necessary for drug-loaded or drug-bound platelets to induce apoptosis, one therapeutic dose should preferably contain more than one platelet per cancer cell, as many transfused platelets will likely never encounter or connect with cancer cells. This may be an unrealistic number in cancer with disseminated disease. Thus, platelets may not be effective as a drug delivery system for treating cancer types with typically high tumor loads, such as leukemia and lymphoma. This obstacle may be overcome by using drug-loaded PEVs or platelet-coated nanoparticles, which are infused in much larger numbers and thus have a greater chance of interconnecting with cancer cells. However, using drug-loaded PEVs or platelet-coated nanoparticles may raise safety concerns because very high numbers of transfused PEVs may theoretically be perilous, as they are generally believed to be thrombogenic [Citation190]. Nonetheless, this function depends on the origin of PEVs [Citation191]. In addition, the exact role of PEVs in hemostasis is controversial, as newer, more sensitive methods reveal a more complex contribution to hemostasis, as they also have fibrinolytic properties [Citation34].
All platelet concentrates contain PEVs, and the number is affected by the production method, storage, and handling [Citation192]. Thus, some amount of PEVs is safe for transfusion, but there is very little data on the infusion of large amounts in humans other than some small clinical trials from the 1990s where ‘infusible platelet membranes,’ i.e. PEVs, were tested as a substitute for platelet transfusion [Citation193]. However, there is evidence suggesting that a high number of PEVs in platelet concentrates is associated with the risk of transfusion reactions [Citation194]. Another aspect of whether PEVs are better suited for drug delivery than whole platelets is their circulation time. Transfused murine PEVs bind almost completely to blood cells or extravasate within minutes [Citation38]. A study on platelet transfusion in patients with different hematological diseases showed that the half-life of transfused PEVs from one apheresis-derived platelet concentrate was 5.3 h – considerably shorter than the platelet half-life of 24 h [Citation195]. Thus, platelets may have critical advantages over PEVs as drug vectors, including beneficial clearance kinetics.
There is also a question regarding platelet preparation. The reviewed papers calculated the efficiency of drug loading or binding using different techniques, including liquid chromatography, mass spectrometry, fluorescence spectrophotometry, and enzyme-linked immunosorbent assay [Citation125,Citation126,Citation135,Citation149]. The amount of drug per platelet can then be calculated, and individual treatment doses with the correct number of platelets equivalent to the desired concentration of free drug can be prepared by washing the platelets and resuspending them in a buffer or plasma before treatment. This method can be referred to as the ‘wash’ strategy (). However, as every product in clinical use needs to be quality controlled, post-quantitation handling and subsequent storage could potentially decay the product, as washing will lead to platelet loss and potentially platelet activation with subsequent drug release. In contrast, platelets will be transfused directly after incubation when implementing a ‘no wash’ strategy. Thus, doses are not calculated by the platelet number but by the drug concentration and suspension volume. Excellent encapsulation efficiency of 86.6% for DOX at 100 µM has been reported [Citation124], meaning only a small fraction of the suspension will be free drug if adapting a ‘no wash’ strategy. This method simplifies the workflow, but it may not be feasible for drug-bound platelets or for loading hydrophilic drugs, which may yield lower encapsulation efficiency.
Figure 5. Clinical implementation of platelet-based drug-delivery systems. Platelets may be harvested from the patients themselves by thrombapheresis or supplied from blood bank storage. Following washing and resuspension in drug solution for loading or binding of drug, platelets are incubated and either transfused immediately a) or after washing and quantitation to calculate equivalent doses of free drug b). PAS, platelet additive solution.
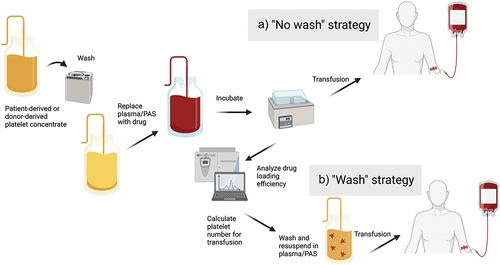
Generally, strategies using platelets for drug delivery presuppose tumor infiltration, although circulating platelets loaded or bound to cytotoxic compounds will in most cases also target CTCs as long as normal platelet functionality is preserved. The only exceptions will be the strategies that depend on the tumor microenvironment for drug release or killing. However, there are strategies for selectively targeting of CTCs, which include platelet [Citation196] and platelet hybrid vesicle decoys [Citation197]. These decoys compete with normal platelets for CTC binding. Otherwise, they have no platelet functionality that may aid immune evasion or CTC extravasation. As there are no cytotoxic elements, these strategies may have good tolerability; however, more studies are necessary to assess their efficacy, which will be limited to metastatic potential.
7. Conclusion
The interplay between platelets and cancer cells is essential in cancer biology. Thus, using platelets or other platelet-engineered hybrid technologies as drug delivery systems is an increasingly interesting strategy to improve drug efficacy and reduce harmful adverse events in cancer. Preclinical data are promising, and this technology may provide therapeutic benefits through selective targeting of cancer cells, improved cancer cell drug uptake, and slowed drug elimination. Platelet concentrates are also readily available from blood banks, relatively cheap to manufacture, and safe for transfusion. Thus, platelets have many advantages as drug delivery systems over synthetic drug carriers. However, unlike synthetic formulations, there is no clinical data on platelet- or platelet-inspired drug delivery systems in humans. Thus, the clinical relevance of this technology remains to be determined.
8. Expert opinion
Our understanding of platelet function beyond its role in hemostasis, especially in cancer biology, has changed profoundly in recent years. This knowledge has inspired platelet-based drug delivery systems to use the exceptional features of platelets to target the tumor microenvironment. The drug delivery systems reviewed in this paper represent some of the great potentials of this platform. Although clinical data in humans are lacking, plenty of studies using animal models show good tolerability and have generally implemented well-proven and safe drugs such as DOX or other cytotoxic compounds. Thus, clinical trials in humans will likely follow, and there is reason to believe that using platelets as drug vectors will improve the therapeutic efficacy of existing therapies for many cancers, possibly with only minor additional financial costs. Although this may be limited to cancer types with a high degree of platelet-cancer interaction, using platelet-based drug delivery systems are promising for prevalent cancer types such as breast, pancreatic, and colorectal cancer. However, as with any novel treatment, the initial clinical trials in humans will likely include heavily treated patients with refractory diseases. In this context, profound drug resistance may mask the primary benefits of using a platelet-based drug delivery system, i.e. selective targeting of cancer cells and optimized pharmacokinetics. Therefore, further development of more complex platelet-based drug delivery systems using a combination of drugs, strategies to improve platelet infiltration, and immunotherapeutic components for optimum anticancer efficacy are required.
This combined approach shows promise in preclinical trials, which underlines the greatest of a number of advantages of platelet-based drug delivery systems over synthetic nanocarriers, namely the dynamic properties of platelets. Not only can platelets be modified, but they can also respond to modifications in the tumor microenvironment, substantially increasing the complexity and potential of the treatment platform. Thus, although multiple studies using platelet-based drug delivery systems have been published recently, the optimal platform is probably not yet developed.
Drug modifications of platelets in platelet concentrates will likely not be performed by blood bank services, at least not more complex modifications such as the conjugation of different molecules to the plasma membrane or manufacturing of platelet hybrid vesicles. Clinical studies, and eventually clinical implementation, will require the availability of advanced good manufacturing practice (GMP) cell-therapy laboratories, which may be challenging in the near future, because this capacity is generally limited. However, these services are likely to become more available with the emergence of advanced cellular therapies, such as CAR-T cells, dendritic cell cancer vaccines, and tissue engineering techniques.
Medical cancer treatment generally requires multiple treatments over extended periods, regardless if the aim is disease stabilization or curation. Thus, treatment centralization is generally limited to rare diseases or complex protocols such as allogeneic hematopoietic stem cell transplantation. In a scenario with insufficient accessibility to GMP cell therapy laboratories and therefore the need for centralized manufacturing, some technical obstacles must be overcome for widespread application. The evidence for the release of drugs from drug-loaded platelets suggests that storage longer than a couple of hours is not advisable, at least for lipophilic drugs. However, loading of hydrophilic drugs and especially the use of membrane-conjugated cytotoxic compounds, may theoretically yield better stability. Still, this may render centralized manufacturing and treatment at local hospitals impossible in many settings owing to logistical challenges. In contrast, as described earlier, there is some evidence for making ‘off-the-shelf products’ of platelet-based drug delivery systems through cryopreservation, meaning production facilities could still be localized far from the patient. However, cryopreservation may introduce challenges with altered platelet functionality [Citation198]; therefore, this remains to be studied in more detail in the setting of platelets as drug carriers.
Another strategy for developing platelet-inspired drug delivery systems and circumventing storage and improper drug loading issues is to use platelet-targeting nanoparticles, which may have superior ex vivo stability. Platelets are then ‘loaded’ or drug bound in vivo before infiltration of the tumor microenvironment, as described in the previous sections [Citation142,Citation144–147]. Although this confers several benefits, including selective nanoparticle cancer cell targeting, it will increase complexity and thus financial cost and may not elude the issues with the immunogenicity of synthetic drug carriers.
In a scenario where the necessary cell therapy facilities are readily available, apheresis-derived autologous platelets will be the likely source for platelet-based drug delivery systems. Subsequently, harvesting, manufacturing, and treatment can be performed in local hospitals without draining blood donor resources. This treatment strategy may be feasible for most cancer patients because medical cancer treatment is generally not commenced with concomitant thrombocytopenia, which impedes platelet harvesting. However, some patients with hematological malignancies may require treatment despite of being thrombocytopenic, and thus there is need for allogenic platelets for manufacturing of the drug delivery systems. Alloimmunization is not uncommon in multi-line treated hematological patients, who generally have received numerous platelet transfusions, and accordingly will require the use of human leukocyte antigen-matched single-donor apheresis-derived platelets. Otherwise, the more available pooled buffy coat-derived platelet concentrates may be supplied from blood banks.
In conclusion, platelet-based drug delivery systems will likely represent a future treatment platform in hematology and oncology, which are in the later stages of preclinical testing and thus may be available for patients within the next decade. This treatment strategy can potentially improve the survival of patients with various cancers without developing new and expensive drugs. However, there still are technical and logistical challenges that has to be overcome before platelet-based drug delivery systems, if proven to be an effective platform in human clinical trials, can be available for the general population.
Article highlights
Platelets interact with cancer cells in the blood and the tumor microenvironment, which confers survival advantages in the cancer cells.
Platelets can transfer molecules to cancer cells by shedding platelet extracellular vesicles.
Platelets can be loaded or conjugated with multiple drugs, thereby slowing drug elimination and increasing selective targeting because of platelet-cancer interactions.
Many of the advantages of platelet drug vectors can be transferred to synthetic nanocarriers by coating them with platelet membranes.
Although multiple animal studies confirm the potential of platelet-based drug delivery, currently there are no human studies.
Declaration of interest
Daniel Cacic’s current employment is financed by Folke Hermansen fond. The authors have no other relevant affiliations or financial involvement with any organization or entity with a financial interest in or financial conflict with the subject matter or materials discussed in the manuscript apart from those disclosed.
Reviewer disclosures
Peer reviewers on this manuscript have no relevant financial or other relationships to disclose.
Additional information
Funding
References
- ReFaey K, Tripathi S, Grewal SS, et al. Cancer mortality rates increasing vs cardiovascular disease mortality decreasing in the world: future implications. Mayo Clin Proc Innov Qual Outcomes. 2021 Jun;5(3):645–653.
- Soerjomataram I, Bray F. Planning for tomorrow: global cancer incidence and the role of prevention 2020-2070. Nat Rev Clin Oncol. 2021 Oct;18(10):663–672.
- Schlander M, Hernandez-Villafuerte K, Cheng CY, et al. How much does it cost to research and develop a new drug? A systematic review and assessment. Pharmacoeconomics. 2021 Nov;39(11):1243–1269.
- Ocran Mattila P, Ahmad R, Hasan SS, et al. Availability, affordability, access, and pricing of anti-cancer medicines in low- and middle-income countries: a systematic review of literature. Front Public Health. 2021;9:628744.
- de Gaetano G. Historical overview of the role of platelets in hemostasis and thrombosis. Haematologica. 2001 Apr;86(4):349–356.
- Varon D, Shai E. Platelets and their microparticles as key players in pathophysiological responses. J Thromb Haemost. 2015 Jun;13(Suppl 1):S40–6.
- Walsh TG, Metharom P, Berndt MC. The functional role of platelets in the regulation of angiogenesis. Platelets. 2015;26(3):199–211.
- Gawaz M, Vogel S. Platelets in tissue repair: control of apoptosis and interactions with regenerative cells. Blood. 2013 Oct 10;122(15):2550–2554.
- Kurokawa T, Zheng YW, Ohkohchi N. Novel functions of platelets in the liver. J Gastroenterol Hepatol. 2016 Apr;31(4):745–751.
- Dovizio M, Bruno A, Contursi A, et al. Platelets and extracellular vesicles in cancer: diagnostic and therapeutic implications. Cancer Metastasis Rev. 2018 Sep;37(2–3):455–467.
- Xu XR, Yousef GM, Ni H. Cancer and platelet crosstalk: opportunities and challenges for aspirin and other anti-platelet agents. Blood. 2018 Mar 8;131(16):1777–1789.
- Li A, Chen J, Liang ZH, et al. Comparison of ultrastructural and nanomechanical signature of platelets from acute myocardial infarction and platelet activation. Biochem Biophys Res Commun. 2017 Apr 29;486(2):245–251.
- Sanada C, Xavier-Ferrucio J, Lu YC, et al. Adult human megakaryocyte-erythroid progenitors are in the CD34+CD38mid fraction. Blood. 2016 Aug 18;128(7):923–933.
- Miyawaki K, Iwasaki H, Jiromaru T, et al. Identification of unipotent megakaryocyte progenitors in human hematopoiesis. Blood. 2017 Jun 22;129(25):3332–3343.
- Notta F, Zandi S, Takayama N, et al. Distinct routes of lineage development reshape the human blood hierarchy across ontogeny. Science. 2016 Jan 8;351(6269):aab2116.
- Rodriguez-Fraticelli AE, Wolock SL, Weinreb CS, et al. Clonal analysis of lineage fate in native haematopoiesis. Nature. 2018 Jan 11;553(7687):212–216.
- Nakamura-Ishizu A, Matsumura T, Stumpf PS, et al. Thrombopoietin metabolically primes hematopoietic stem cells to megakaryocyte-lineage differentiation. Cell Rep. 2018 Nov 13;25(7):1772–1785.e6.
- Avecilla ST, Hattori K, Heissig B, et al. Chemokine-mediated interaction of hematopoietic progenitors with the bone marrow vascular niche is required for thrombopoiesis. Nature Med. 2004;10(1):64–71. DOI:10.1038/nm973
- Stegner D, vanEeuwijk JMM, Angay O, et al. Thrombopoiesis is spatially regulated by the bone marrow vasculature. Nat Commun. 2017 Jul 25;8(1):127.
- Jiang J, Woulfe DS, Papoutsakis ET. Shear enhances thrombopoiesis and formation of microparticles that induce megakaryocytic differentiation of stem cells. Blood. 2014 Sep 25;124(13):2094–2103.
- Potts KS, Farley A, Dawson CA, et al. Membrane budding is a major mechanism of in vivo platelet biogenesis. J Exp Med. 2020 Sep 7;217(9). DOI:10.1084/jem.20191206
- Nishimura S, Nagasaki M, Kunishima S, et al. IL-1alpha induces thrombopoiesis through megakaryocyte rupture in response to acute platelet needs. J Cell Bio. 2015 May 11;209(3):453–466.
- Angénieux C, Maître B, Eckly A, et al. Time-dependent decay of mRNA and ribosomal RNA during platelet aging and its correlation with translation activity. PLoS ONE. 2016;11(1):e0148064–e0148064. DOI:10.1371/journal.pone.0148064
- Mills EW, Green R, Ingolia NT. Slowed decay of mRnas enhances platelet specific translation. Blood. 2017 Apr 27;129(17):e38–48.
- Huang J, Swieringa F, Solari FA, et al. Assessment of a complete and classified platelet proteome from genome-wide transcripts of human platelets and megakaryocytes covering platelet functions. Sci Rep. 2021 Jun 11;11(1):12358.
- Parsons MEM, Szklanna PB, Guererro JA, et al. Platelet releasate proteome profiling reveals a core set of proteins with low variance between healthy adults. Proteomics. 2018 Jun 22;18(15):e1800219.
- Velez P, Izquierdo I, Rosa I, et al. A 2D-DIGE-based proteomic analysis reveals differences in the platelet releasate composition when comparing thrombin and collagen stimulations. Sci Rep. 2015;5(1):8198. DOI:10.1038/srep08198
- Jonnalagadda D, Izu LT, Whiteheart SW. Platelet secretion is kinetically heterogeneous in an agonist-responsive manner. Blood. 2012 Dec 20;120(26):5209–5216.
- Maynard DM, Heijnen HF, Horne MK, et al. Proteomic analysis of platelet alpha-granules using mass spectrometry. J Thromb Haemost. 2007 Sep;5(9):1945–1955.
- Zufferey A, Schvartz D, Nolli S, et al. Characterization of the platelet granule proteome: evidence of the presence of MHC1 in alpha-granules. J Proteomics. 2014;101:130–140.
- Hernandez-Ruiz L, Valverde F, Jimenez-Nunez MD, et al. Organellar proteomics of human platelet dense granules reveals that 14-3-3zeta is a granule protein related to atherosclerosis. J Proteome Res. 2007 Nov;6(11):4449–4457.
- Heijnen HF, Schiel AE, Fijnheer R, et al. Activated platelets release two types of membrane vesicles: microvesicles by surface shedding and exosomes derived from exocytosis of multivesicular bodies and alpha-granules. Blood. 1999 Dec 1;94(11):3791–3799.
- Théry C, Witwer KW, Aikawa E, et al. Minimal information for studies of extracellular vesicles 2018 (MISEV2018): a position statement of the international society for extracellular vesicles and update of the MISEV2014 guidelines. J Extracell Vesicles. 2018;7(1):1535750. DOI:10.1080/20013078.2018.1535750
- Berckmans RJ, Lacroix R, Hau CM, et al. Extracellular vesicles and coagulation in blood from healthy humans revisited. J Extracell Vesicles. 2019;8(1):1688936. DOI:10.1080/20013078.2019.1688936
- Arraud N, Linares R, Tan S, et al. Extracellular vesicles from blood plasma: determination of their morphology, size, phenotype and concentration. J Thromb Haemost. 2014 May;12(5):614–627.
- Ponomareva AA, Nevzorova TA, Mordakhanova ER, et al. Intracellular origin and ultrastructure of platelet-derived microparticles. J Thromb Haemost. 2017 Aug;15(8):1655–1667.
- De Paoli SH, Tegegn TZ, Elhelu OK, et al. Dissecting the biochemical architecture and morphological release pathways of the human platelet extracellular vesiculome. Cell Mol Life Sci. 2018 Oct;75(20):3781–3801.
- Marcoux G, Laroche A, Hasse S, et al. Platelet EVs contain an active proteasome involved in protein processing for antigen presentation via MHC-I molecules. Blood. 2021 Dec 23;138(25):2607–2620.
- Cauwenberghs S, Feijge MA, Harper AG, et al. Shedding of procoagulant microparticles from unstimulated platelets by integrin-mediated destabilization of actin cytoskeleton. FEBS Lett. 2006 Oct 2;580(22):5313–5320.
- Agbani EO, van den Bosch MT, Brown E, et al. Coordinated membrane ballooning and procoagulant spreading in human platelets. Circulation. 2015 Oct 13;132(15):1414–1424.
- Agbani EO, Williams CM, Hers I, et al. Membrane ballooning in aggregated platelets is synchronised and mediates a surge in microvesiculation. Sci Rep. 2017 Jun 5;7(1):2770.
- Aatonen MT, Ohman T, Nyman TA, et al. Isolation and characterization of platelet-derived extracellular vesicles. J Extracell Vesicles. 2014;3(1):3. DOI:10.3402/jev.v3.24692
- Vismara M, Manfredi M, Zarà M, et al. Proteomic and functional profiling of platelet-derived extracellular vesicles released under physiological or tumor-associated conditions. Cell Death Discov. 2022 Nov 26;8(1):467.
- Mahajan A, Brunson A, Adesina O, et al. The incidence of cancer-associated thrombosis is increasing over time. Blood Adv. 2022 Jan 11;6(1):307–320.
- Li A, La J, May SB, et al. Derivation and validation of a clinical risk assessment model for cancer-associated thrombosis in two unique us health care systems. J Clin Oncol. 2023 Jan 10:Jco2201542. DOI:10.1200/JCO.22.01542
- Zhang X, Lv Z, Yu H, et al. The clinicopathological and prognostic role of thrombocytosis in patients with cancer: a meta-analysis. Oncol Lett. 2017 Jun;13(6):5002–5008.
- Rao XD, Zhang H, Xu ZS, et al. Poor prognostic role of the pretreatment platelet counts in colorectal cancer: a meta-analysis. Medicine (Baltimore). 2018 Jun;97(23):e10831.
- Wang YH, Kang JK, Zhi YF, et al. The pretreatment thrombocytosis as one of prognostic factors for gastric cancer: a systematic review and meta-analysis. Int J Surg. 2018 May;53:304–311.
- Warny M, Helby J, Birgens HS, et al. Arterial and venous thrombosis by high platelet count and high hematocrit: 108 521 individuals from the copenhagen general population study. J Thromb Haemost. 2019 Nov;17(11):1898–1911.
- Palacios-Acedo AL, Langiu M, Crescence L, et al. Platelet and cancer-cell interactions modulate cancer-associated thrombosis risk in different cancer types. Cancers (Basel). 2022 Jan 30;14(3):730.
- Zhang SR, Yao L, Wang WQ, et al. Tumor-infiltrating platelets predict postsurgical survival in patients with pancreatic ductal adenocarcinoma. Ann Surg Oncol. 2018 Dec;25(13):3984–3993.
- Wang J, Zhang M, Zhou T, et al. Role of platelet infiltration as independent prognostic marker for gastric adenocarcinomas. J Clin Lab Anal. 2020 Aug;34(8):e23320.
- Cho MS, Li J, Gonzalez-Delgado R, et al. The effect of platelet G proteins on platelet extravasation and tumor growth in the murine model of ovarian cancer. Blood Adv. 2021;5(7):1947–1951. DOI:10.1182/bloodadvances.2020003410
- Miao Y, Xu Z, Feng W, et al. Platelet infiltration predicts survival in postsurgical colorectal cancer patients. Int J Cancer. 2022 Feb 1;150(3):509–520.
- Xu SS, Xu HX, Wang WQ, et al. Tumor-infiltrating platelets predict postoperative recurrence and survival in resectable pancreatic neuroendocrine tumor. World J Gastroenterol. 2019 Nov 7;25(41):6248–6257.
- Yu LX, Yan L, Yang W, et al. Platelets promote tumour metastasis via interaction between TLR4 and tumour cell-released high-mobility group box1 protein. Nat Commun. 2014 Oct 28;5(1):5256.
- Adesanya MA, Maraveyas A, Madden L. Differing mechanisms of thrombin generation in live haematological and solid cancer cells determined by calibrated automated thrombography. Blood Coagul Fibrinolysis. 2017 Dec;28(8):602–611.
- Takemoto A, Okitaka M, Takagi S, et al. A critical role of platelet TGF-β release in podoplanin-mediated tumour invasion and metastasis. Sci Rep. 2017 Feb 8;7(1):42186.
- Qi C, Wei B, Zhou W, et al. P-selectin-mediated platelet adhesion promotes tumor growth. Oncotarget. 2015 Mar 30;6(9):6584–6596.
- Thomas GM, Panicot-Dubois L, Lacroix R, et al. Cancer cell-derived microparticles bearing P-selectin glycoprotein ligand 1 accelerate thrombus formation in vivo. J Exp Med. 2009 Aug 31;206(9):1913–1927.
- Mammadova-Bach E, Gil-Pulido J, Sarukhanyan E, et al. Platelet glycoprotein VI promotes metastasis through interaction with cancer cell-derived galectin-3. Blood. 2020 Apr 2;135(14):1146–1160.
- Mammadova-Bach E, Zigrino P, Brucker C, et al. Platelet integrin α6β1 controls lung metastasis through direct binding to cancer cell-derived ADAM9. JCI Insight. 2016 Sep 8;1(14):e88245.
- Mitrugno A, Williams D, Kerrigan SW, et al. A novel and essential role for FcγRIIa in cancer cell-induced platelet activation. Blood. 2014 Jan 9;123(2):249–260.
- Haemmerle M, Bottsford-Miller J, Pradeep S, et al. FAK regulates platelet extravasation and tumor growth after antiangiogenic therapy withdrawal. J Clin Invest. 2016 May 2;126(5):1885–1896.
- Cho MS, Noh K, Haemmerle M, et al. Role of ADP receptors on platelets in the growth of ovarian cancer. Blood. 2017 Sep 7;130(10):1235–1242.
- Gebremeskel S, LeVatte T, Liwski RS, et al. The reversible P2Y12 inhibitor ticagrelor inhibits metastasis and improves survival in mouse models of cancer. Int J Cancer. 2015 Jan 1;136(1):234–240.
- Palacios-Acedo AL, Mezouar S, Mège D, et al. P2RY12-inhibitors reduce cancer-associated thrombosis and tumor growth in pancreatic cancers. Front Oncol. 2021;11:704945.
- Chan AT, Ogino S, Fuchs CS. Aspirin use and survival after diagnosis of colorectal cancer. JAMA. 2009 Aug 12;302(6):649–658.
- Bains SJ, Mahic M, Myklebust TA, et al. Aspirin as secondary prevention in patients with colorectal cancer: an unselected population-based study. J Clin Oncol. 2016 Jul 20;34(21):2501–2508.
- Cao Y, Nishihara R, Wu K, et al. Population-wide impact of long-term use of aspirin and the risk for cancer. JAMA Oncol. 2016 Jun 1;2(6):762–769.
- Qiao Y, Yang T, Gan Y, et al. Associations between aspirin use and the risk of cancers: a meta-analysis of observational studies. BMC Cancer. 2018 Mar 13;18(1):288.
- Guirguis-Blake JM, Evans CV, Perdue LA, et al. Aspirin use to prevent cardiovascular disease and colorectal cancer: updated evidence report and systematic review for the us preventive services task force. JAMA. 2022 Apr 26;327(16):1585–1597.
- Kaufmann CC, Lyon AR, Wojta J, et al. Is P2Y12 inhibitor therapy associated with an increased risk of cancer? Eur Heart J Cardiovasc Pharmacother. 2019 Apr 1;5(2):100–104.
- Anvari S, Osei E, Maftoon N. Interactions of platelets with circulating tumor cells contribute to cancer metastasis. Sci Rep. 2021 Jul 29;11(1):15477.
- Egan K, Cooke N, Kenny D. Living in shear: platelets protect cancer cells from shear induced damage. Clin Exp Metastasis. 2014 Aug;31(6):697–704.
- Echtler K, Konrad I, Lorenz M, et al. Platelet GPIIb supports initial pulmonary retention but inhibits subsequent proliferation of melanoma cells during hematogenic metastasis. PLoS ONE. 2017;12(3):e0172788. DOI:10.1371/journal.pone.0172788
- Yu M, Bardia A, Wittner BS, et al. Circulating breast tumor cells exhibit dynamic changes in epithelial and mesenchymal composition. Science. 2013 Feb 1;339(6119):580–584.
- Guo Y, Cui W, Pei Y, et al. Platelets promote invasion and induce epithelial to mesenchymal transition in ovarian cancer cells by TGF-beta signaling pathway. Gynecol Oncol. 2019 Jun;153(3):639–650.
- Angénieux C, Dupuis A, Gachet C, et al. Cell surface expression of HLA I molecules as a marker of young platelets. J Thromb Haemost. 2019 Sep;17(9):1511–1521.
- Placke T, Örgel M, Schaller M, et al. Platelet-derived MHC class I confers a pseudonormal phenotype to cancer cells that subverts the antitumor reactivity of natural killer immune cells. Cancer Res. 2012 Jan 15;72(2):440–448.
- Nieswandt B, Hafner M, Echtenacher B, et al. Lysis of tumor cells by natural killer cells in mice is impeded by platelets. Cancer Res. 1999 Mar 15;59(6):1295–1300.
- Kopp HG, Placke T, Salih HR. Platelet-derived transforming growth factor-beta down-regulates NKG2D thereby inhibiting natural killer cell antitumor reactivity. Cancer Res. 2009 Oct 1;69(19):7775–7783.
- Sadallah S, Schmied L, Eken C, et al. Platelet-derived ectosomes reduce NK cell function. J Immunol (Baltimore, Md : 1950). 2016 Sep 1;197(5):1663–1671. DOI:10.4049/jimmunol.1502658
- Maurer S, Kropp KN, Klein G, et al. Platelet-mediated shedding of NKG2D ligands impairs NK cell immune-surveillance of tumor cells. Oncoimmunology. 2018;7(2):e1364827. DOI:10.1080/2162402X.2017.1364827
- Danese S, de la Motte C, Reyes BM, et al. Cutting edge: t cells trigger CD40-dependent platelet activation and granular RANTES release: a novel pathway for immune response amplification. J Immunol. 2004 Feb 15;172(4):2011–2015.
- Meikle CK, Meisler AJ, Bird CM, et al. Platelet-T cell aggregates in lung cancer patients: implications for thrombosis. PLoS ONE. 2020;15(8):e0236966. DOI:10.1371/journal.pone.0236966
- Chapman LM, Aggrey AA, Field DJ, et al. Platelets present antigen in the context of MHC class I. J Immunol. 2012 Jul 15;189(2):916–923.
- Rachidi S, Metelli A, Riesenberg B, et al. Platelets subvert T cell immunity against cancer via GARP-TGFβ axis. Sci Immunol. 2017 May 5;2(11). DOI:10.1126/sciimmunol.aai7911
- Metelli A, Wu BX, Riesenberg B, et al. Thrombin contributes to cancer immune evasion via proteolysis of platelet-bound GARP to activate LTGF-β. Sci Transl Med. 2020 Jan 8;12(525). DOI:10.1126/scitranslmed.aay4860
- Zaslavsky AB, Adams MP, Cao X, et al. Platelet PD-L1 suppresses anti-cancer immune cell activity in PD-L1 negative tumors. Sci Rep. 2020 Nov 9;10(1):19296.
- Riesenberg BP, Ansa-Addo EA, Gutierrez J, et al. Cutting edge: targeting thrombocytes to rewire anticancer immunity in the tumor microenvironment and potentiate efficacy of PD-1 blockade. J Immunol. 2019 Sep 1;203(5):1105–1110.
- Zhou S, Liu M, Ren F, et al. The landscape of bispecific T cell engager in cancer treatment. Biomark Res. 2021 May 26;9(1):38.
- Lutz MS, Klimovich B, Maurer S, et al. Platelets subvert antitumor efficacy of T cell-recruiting bispecific antibodies. J Immunother Cancer. 2022 Feb;10(2):e003655.
- Cao Y, Nishihara R, Qian ZR, et al. Regular aspirin use associates with lower risk of colorectal cancers with low numbers of tumor-infiltrating lymphocytes. Gastroenterology. 2016 Nov;151(5):879–892.e4.
- Vargason AM, Anselmo AC, Mitragotri S. The evolution of commercial drug delivery technologies. Nat Biomed Eng. 2021 Sep;5(9):951–967.
- Bulbake U, Doppalapudi S, Kommineni N, et al. Liposomal formulations in clinical use: an updated review. Pharmaceutics. 2017 Mar 27;9(4):12.
- Alvi M, Yaqoob A, Rehman K, et al. PLGA-based nanoparticles for the treatment of cancer: current strategies and perspectives. AAPS Open. 2022 Aug 01;8(1):12.
- Miao Y, Yang T, Yang S, et al. Protein nanoparticles directed cancer imaging and therapy. Nano Convergence. 2022 Jan 08;9(1):2.
- Sharma S, Parveen R, Chatterji BP. Toxicology of nanoparticles in drug delivery. Curr Pathobiol Rep. 2021;9(4):133–144.
- Sieber S, Grossen P, Uhl P, et al. Zebrafish as a predictive screening model to assess macrophage clearance of liposomes in vivo. Nanomedicine. 2019 Apr;17:82–93.
- Weber F, Ivan DC, Proulx ST, et al. Beyond trial and error: a systematic development of liposomes targeting primary macrophages. Adv NanoBiomed Res. 2021;1(3):2000098. DOI:10.1002/anbr.202000098
- Levchenko TS, Rammohan R, Lukyanov AN, et al. Liposome clearance in mice: the effect of a separate and combined presence of surface charge and polymer coating. Int J Pharm. 2002 Jun 20;240(1–2):95–102.
- Kozma GT, Mészáros T, Vashegyi I, et al. Pseudo-anaphylaxis to Polyethylene Glycol (PEG)-coated liposomes: roles of anti-PEG IgM and complement activation in a porcine model of human infusion reactions. ACS Nano. 2019 Aug 27;13(8):9315–9324.
- Petersen GH, Alzghari SK, Chee W, et al. Meta-analysis of clinical and preclinical studies comparing the anticancer efficacy of liposomal versus conventional non-liposomal doxorubicin. J Control Release. 2016 Jun 28;232:255–264.
- Ni C, Fang J, Qian H, et al. Liposomal doxorubicin-related palmar-plantar erythrodysesthesia (hand-foot syndrome): a case report. J Int Med Res. 2020 Dec;48(12):300060520974854.
- Visco C, Pregnolato F, Ferrarini I, et al. Efficacy of R-COMP in comparison to R-CHOP in patients with DLBCL: a systematic review and single-arm metanalysis. Crit Rev Oncol Hematol. 2021 Jul;163:103377.
- Ghasemi K, Vaseghi G, Mansourian M. Pharmacological interventions for preventing anthracycline-induced clinical and subclinical cardiotoxicity: a network meta-analysis of metastatic breast cancer. J Oncol Pharm Pract. 2021 Mar;27(2):414–427.
- Tardi P, Johnstone S, Harasym N, et al. In vivo maintenance of synergistic cytarabine: daunorubicin ratios greatly enhances therapeutic efficacy. Leuk Res. 2009 Jan;33(1):129–139.
- Kim HP, Gerhard B, Harasym TO, et al. Liposomal encapsulation of a synergistic molar ratio of cytarabine and daunorubicin enhances selective toxicity for acute myeloid leukemia progenitors as compared to analogous normal hematopoietic cells. Exp Hematol. 2011 Jul;39(7):741–750.
- Lim WS, Tardi PG, Dos Santos N, et al. Leukemia-selective uptake and cytotoxicity of CPX-351, a synergistic fixed-ratio cytarabine: daunorubicin formulation, in bone marrow xenografts. Leuk Res. 2010 Sep;34(9):1214–1223.
- Lancet JE, Uy GL, Cortes JE, et al. CPX-351 (cytarabine and daunorubicin) liposome for injection versus conventional cytarabine plus daunorubicin in older patients with newly diagnosed secondary acute myeloid leukemia. J Clin Oncol. 2018 Sep 10;36(26):2684–2692.
- Cortes JE, Lin TL, Uy GL, et al. Quality-adjusted time without symptoms of disease or toxicity (Q-TWiST) analysis of CPX-351 versus 7 + 3 in older adults with newly diagnosed high-risk/secondary AML. J Hematol Oncol. 2021 Jul 13;14(1):110.
- Bewersdorf JP, Goshua G, Patel KK, et al. Cost-effectiveness of liposomal cytarabine-daunorubicin (CPX-351) compared to conventional cytarabine-daunorubicin chemotherapy in acute myeloid leukemia. Blood. 2021;138(Supplement 1):113–113. DOI:10.1182/blood-2021-144992
- Mastrorilli G, Fiorentino F, Tucci C, et al. Cost analysis of platelet transfusion in Italy for patients with chronic liver disease and associated thrombocytopenia undergoing elective procedures. Clinicoecon Outcomes Res. 2022;14:205–220.
- Michael JV, Wurtzel JGT, Mao GF, et al. Platelet microparticles infiltrating solid tumors transfer miRnas that suppress tumor growth. Blood. 2017 Aug 3;130(5):567–580.
- French SL, Butov KR, Allaeys I, et al. Platelet-derived extracellular vesicles infiltrate and modify the bone marrow during inflammation. Blood Adv. 2020 Jul 14;4(13):3011–3023.
- Ma Q, Bai J, Xu J, et al. Reshaping the inflammatory environment in rheumatoid arthritis joints by targeting delivery of berberine with platelet-derived extracellular vesicles. Adv NanoBiomed Res. 2021;1(11):2100115. DOI:10.1002/anbr.202100115
- Laffont B, Corduan A, Ple H, et al. Activated platelets can deliver mRNA regulatory Ago2*microRNA complexes to endothelial cells via microparticles. Blood. 2013 Jul 11;122(2):253–261.
- Cacic D, Reikvam H, Nordgård O, et al. Platelet microparticles protect acute myelogenous leukemia cells against daunorubicin-induced apoptosis. Cancers (Basel). 2021;13(8):1870. DOI:10.3390/cancers13081870
- Liang H, Yan X, Pan Y, et al. MicroRNA-223 delivered by platelet-derived microvesicles promotes lung cancer cell invasion via targeting tumor suppressor EPB41L3. Mol Cancer. 2015 Mar 11;14(1):58.
- Qu M, Zou X, Fang F, et al. Platelet-derived microparticles enhance megakaryocyte differentiation and platelet generation via miR-1915-3p. Nat Commun. 2020 Oct 2;11(1):4964.
- Vismara M, Zarà M, Negri S, et al. Platelet-derived extracellular vesicles regulate cell cycle progression and cell migration in breast cancer cells. Biochim Biophys Acta, Mol Cell Res. 2021 Jan;1868(1):118886.
- Muley H, Fadó R, Rodríguez-Rodríguez R, et al. Drug uptake-based chemoresistance in breast cancer treatment. Biochem Pharmacol. 2020 Jul;177:113959.
- Xu P, Zuo H, Chen B, et al. Doxorubicin-loaded platelets as a smart drug delivery system: an improved therapy for lymphoma. Sci Rep. 2017 Feb 15;7(1):42632.
- Li QR, Xu HZ, Xiao RC, et al. Platelets are highly efficient and efficacious carriers for tumor-targeted nano-drug delivery. Drug Deliv. 2022 Dec;29(1):937–949.
- Wu YW, Huang CC, Changou CA, et al. Clinical-grade cryopreserved doxorubicin-loaded platelets: role of cancer cells and platelet extracellular vesicles activation loop. J Biomed Sci. 2020 Mar 23;27(1):45. DOI:10.1186/s12929-020-00633-2
- Sarkar S, Alam MA, Shaw J, et al. Drug delivery using platelet cancer cell interaction. Pharm Res. 2013 Nov;30(11):2785–2794.
- Ying M, Zhuang J, Wei X, et al. Remote-loaded platelet vesicles for disease-targeted delivery of therapeutics. Adv Funct Mater. 2018 May 30;28(22):1801032.
- Li T, Chen T, Chen H, et al. Engineered platelet-based micro/nanomotors for cancer therapy. Small. 2021 Dec;17(52):e2104912.
- Yu Y, Cheng Q, Ji X, et al. Engineered drug-loaded cellular membrane nanovesicles for efficient treatment of postsurgical cancer recurrence and metastasis. Sci Adv. 2022 Dec 9;8(49):eadd3599.
- Hu Q, Sun W, Qian C, et al. Relay drug delivery for amplifying targeting signal and enhancing anticancer efficacy. Adv Mater. 2017;29(13):1605803. DOI:10.1002/adma.201605803
- Matyszewska D. The influence of charge and lipophilicity of daunorubicin and idarubicin on their penetration of model biological membranes - Langmuir monolayer and electrochemical studies. Biochim Biophys Acta Biomembr. 2020 Feb 1;1862(2):183104.
- Andreev E, Brosseau N, Carmona E, et al. The human organic cation transporter OCT1 mediates high affinity uptake of the anticancer drug daunorubicin. Sci Rep. 2016 Feb 10;6(1):20508.
- Gaertner F, Ahmad Z, Rosenberger G, et al. Migrating platelets are mechano-scavengers that collect and bundle bacteria. Cell. 2017 Nov 30;171(6):1368–1382.e23.
- Tanaka H, Horioka K, Hasebe T, et al. Treatment of hepatocellular carcinoma with autologous platelets encapsulating sorafenib or lenvatinib: a novel therapy exploiting tumor-platelet interactions. Int J Cancer. 2022 May 15;150(10):1640–1653.
- Li X, Lovell JF, Yoon J, et al. Clinical development and potential of photothermal and photodynamic therapies for cancer. Nat Rev Clin Oncol. 2020 Nov;17(11):657–674.
- Rao L, Bu L-L, Ma L, et al. Platelet-facilitated photothermal therapy of head and neck squamous cell carcinoma. Angewandte Chemie. 2018;57(4):986–991. DOI:10.1002/anie.201709457
- Zhang Y, Sun Y, Dong X, et al. A platelet intelligent vehicle with navigation for cancer photothermal-chemotherapy. ACS Nano. 2022 Apr 26;16(4):6359–6371.
- Dai L, Gu N, Chen BA, et al. Human platelets repurposed as vehicles for in vivo imaging of myeloma xenotransplants. Oncotarget. 2016 Apr 19;7(16):21076–21090.
- Xu P, Zuo H, Zhou R, et al. Doxorubicin-loaded platelets conjugated with anti-CD22 mAbs: a novel targeted delivery system for lymphoma treatment with cardiopulmonary avoidance. Oncotarget. 2017 Aug 29;8(35):58322–58337. DOI:10.18632/oncotarget.16871
- Fan X, Wang K, Lu Q, et al. Surface-anchored tumor microenvironment-responsive protein nanogel-platelet system for cytosolic delivery of therapeutic protein in the post-surgical cancer treatment. Acta Biomater. 2022 Dec;154:412–423.
- Yap ML, McFadyen JD, Wang X, et al. Activated platelets in the tumor microenvironment for targeting of antibody-drug conjugates to tumors and metastases. Theranostics. 2019;9(4):1154–1169. DOI:10.7150/thno.29146
- Li J, Sharkey CC, Wun B, et al. Genetic engineering of platelets to neutralize circulating tumor cells. J Control Release. 2016 Apr 28;228:38–47.
- Ortiz-Otero N, Marshall JR, Lash BW, et al. Platelet mediated TRAIL delivery for efficiently targeting circulating tumor cells. Nanoscale Adv. 2020 Sep 16;2(9):3942–3953.
- Zhao W, Li T, Long Y, et al. Self-promoted albumin-based nanoparticles for combination therapy against metastatic breast cancer via a hyperthermia-induced “platelet bridge”. ACS Appl Mater Interfaces. 2021 Jun 9;13(22):25701–25714.
- Li S, Li L, Lin X, et al. Targeted inhibition of tumor inflammation and tumor-platelet crosstalk by nanoparticle-mediated drug delivery mitigates cancer metastasis. ACS Nano. 2022 Jan 25;16(1):50–67.
- Guo R, Deng M, He X, et al. Fucoidan-functionalized activated platelet-hitchhiking micelles simultaneously track tumor cells and remodel the immunosuppressive microenvironment for efficient metastatic cancer treatment. Acta Pharm Sin B. 2022 Jan;12(1):467–482.
- Wang C, Sun W, Ye Y, et al. In situ activation of platelets with checkpoint inhibitors for post-surgical cancer immunotherapy. Nat Biomed Eng. 2017 Jan 23;1(2):0011.
- Lu Q, Ye H, Wang K, et al. Bioengineered platelets combining chemotherapy and immunotherapy for postsurgical melanoma treatment: internal core-loaded doxorubicin and external surface-anchored anti-PD-L1 antibody backpacks. Nano Lett. 2022 Apr 13;22(7):3141–3150.
- Hu Q, Sun W, Wang J, et al. Conjugation of haematopoietic stem cells and platelets decorated with anti-PD-1 antibodies augments anti-leukaemia efficacy. Nat Biomed Eng. 2018 Nov;2(11):831–840. DOI:10.1038/s41551-018-0310-2
- Hu Q, Li H, Archibong E, et al. Inhibition of post-surgery tumour recurrence via a hydrogel releasing CAR-T cells and anti-PDL1-conjugated platelets. Nat Biomed Eng. 2021 Sep;5(9):1038–1047.
- Zhang X, Wang J, Chen Z, et al. Engineering PD-1-presenting platelets for cancer immunotherapy. Nano Lett. 2018 Sep 12;18(9):5716–5725.
- Li Z, Ding Y, Liu J, et al. Depletion of tumor associated macrophages enhances local and systemic platelet-mediated anti-PD-1 delivery for post-surgery tumor recurrence treatment. Nat Commun. 2022 Apr 6;13(1):1845.
- Li H, Wang Z, Chen Z, et al. Disrupting tumour vasculature and recruitment of aPDL1-loaded platelets control tumour metastasis. Nat Commun. 2021 May 13;12(1):2773. DOI:10.1038/s41467-021-22674-3
- Gao Y, Chen X, Wang B, et al. Engineering Platelets with PDL1 antibodies and iron oxide nanoparticles for postsurgical cancer immunotherapy. ACS Appl Bio Mater. 2023 Jan 16;6(1):257–266.
- Han X, Chen J, Chu J, et al. Platelets as platforms for inhibition of tumor recurrence post-physical therapy by delivery of anti-PD-L1 checkpoint antibody. J Control Release. 2019 Jun 28;304:233–241.
- Hu CM, Fang RH, Wang KC, et al. Nanoparticle biointerfacing by platelet membrane cloaking. Nature. 2015 Oct 1;526(7571):118–121.
- Wang H, Wu J, Williams GR, et al. Platelet-membrane-biomimetic nanoparticles for targeted antitumor drug delivery. J Nanobiotechnology. 2019 May 13;17(1):60.
- Zhou M, Lai W, Li G, et al. Platelet membrane-coated and VAR2CSA malaria protein-functionalized nanoparticles for targeted treatment of primary and metastatic cancer. ACS Appl Mater Interfaces. 2021 Jun 9;13(22):25635–25648.
- Mei D, Gong L, Zou Y, et al. Platelet membrane-cloaked paclitaxel-nanocrystals augment postoperative chemotherapeutical efficacy. J Control Release. 2020 Aug 10;324:341–353.
- Pan V, Siva PN, Modery-Pawlowski CL, et al. Targeted killing of metastatic cells using a platelet-inspired drug delivery system [10.1039/C5RA05339K]. RSC Adv. 2015;5(57):46218–46228. DOI:10.1039/C5RA05339K
- Li J, Ai Y, Wang L, et al. Targeted drug delivery to circulating tumor cells via platelet membrane-functionalized particles. Biomaterials. 2016 Jan;76:52–65.
- Hu Q, Sun W, Qian C, et al. Anticancer platelet-mimicking nanovehicles. Adv Mater. 2015 Nov 25;27(44):7043–7050.
- Kim MW, Lee G, Niidome T, et al. Platelet-like gold nanostars for cancer therapy: the ability to treat cancer and evade immune reactions. Front Bioeng Biotechnol. 2020;8:133.
- Yang H, Ding Y, Tong Z, et al. Ph-responsive hybrid platelet membrane-coated nanobomb with deep tumor penetration ability and enhanced cancer thermal/chemodynamic therapy. Theranostics. 2022;12(9):4250–4268. DOI:10.7150/thno.68996
- Pei W, Huang B, Chen S, et al. Platelet-mimicking drug delivery nanoparticles for enhanced chemo-photothermal therapy of breast cancer. Int J Nanomedicine. 2020;15:10151–10167.
- Ye H, Wang K, Wang M, et al. Bioinspired nanoplatelets for chemo-photothermal therapy of breast cancer metastasis inhibition. Biomaterials. 2019 Jun;206:1–12.
- Chen M, Wang P, Jiang D, et al. Platelet membranes coated gold nanocages for tumor targeted drug delivery and amplificated low-dose radiotherapy. Front Oncol. 2021;11:793006.
- Bahmani B, Gong H, Luk BT, et al. Intratumoral immunotherapy using platelet-cloaked nanoparticles enhances antitumor immunity in solid tumors. Nat Commun. 2021 Mar 31;12(1):1999. DOI:10.1038/s41467-021-22311-z
- Hu Q, Qian C, Sun W, et al. Engineered nanoplatelets for enhanced treatment of multiple myeloma and thrombus. Adv Mater. 2016 Nov;28(43):9573–9580.
- Zhou L, Feng W, Mao Y, et al. Nanoengineered sonosensitive platelets for synergistically augmented sonodynamic tumor therapy by glutamine deprivation and cascading thrombosis. Bioact Mater. 2023 Jun;24:26–36.
- Verheul HM, Lolkema MP, Qian DZ, et al. Platelets take up the monoclonal antibody bevacizumab. Clin Cancer Res. 2007 Sep 15;13(18 Pt 1):5341–5347.
- Bilalis P, Tziveleka L-A, Varlas S, et al. Ph-Sensitive nanogates based on poly(l-histidine) for controlled drug release from mesoporous silica nanoparticles [10.1039/C5PY01841B]. Polym Chem. 2016;7(7):1475–1485. DOI:10.1039/C5PY01841B
- Ho JD, Yeh R, Sandstrom A, et al. Crystal structure of human aquaporin 4 at 1.8 a and its mechanism of conductance. Proc Natl Acad Sci U S A. 2009 May 5;106(18):7437–7442.
- Girardi E, César-Razquin A, Lindinger S, et al. A widespread role for SLC transmembrane transporters in resistance to cytotoxic drugs. Nat Chem Biol. 2020 Apr;16(4):469–478.
- Ueda K, Hosokawa M, Iwakawa S. Cellular uptake of decitabine by equilibrative nucleoside transporters in HCT116 Cells. Biol Pharm Bull. 2015;38(8):1113–1119.
- Arimany-Nardi C, Errasti-Murugarren E, Minuesa G, et al. Nucleoside transporters and human organic cation transporter 1 determine the cellular handling of DNA-methyltransferase inhibitors. Br J Pharmacol. 2014 Aug;171(16):3868–3880.
- Damaraju VL, Mowles D, Yao S, et al. Role of human nucleoside transporters in the uptake and cytotoxicity of azacitidine and decitabine. Nucleosides Nucleotides Nucleic Acids. 2012;31(3):236–255. DOI:10.1080/15257770.2011.652330
- Candelaria M, Corrales-Alfaro C, Gutiérrez-Hernández O, et al. Expression levels of human equilibrative nucleoside transporter 1 and deoxycytidine kinase enzyme as prognostic factors in patients with acute myeloid leukemia treated with cytarabine. Chemotherapy. 2016;61(6):313–318. DOI:10.1159/000445370
- Megías-Vericat JE, Martínez-Cuadrón D, Solana-Altabella A, et al. Systematic review of pharmacogenetics of ABC and SLC transporter genes in acute myeloid leukemia. Pharmaceutics. 2022 Apr 17;14(4). DOI:10.3390/pharmaceutics14040878
- Belkouz A, Labeur TA, Dierks J, et al. Prognostic immunohistochemical biomarkers of chemotherapy efficacy in biliary tract cancer: a systematic review and meta-analysis. Crit Rev Oncol Hematol. 2019 Sep;141:82–94.
- Bird NT, Elmasry M, Jones R, et al. Immunohistochemical hENT1 expression as a prognostic biomarker in patients with resected pancreatic ductal adenocarcinoma undergoing adjuvant gemcitabine-based chemotherapy. Br J Surg. 2017 Mar;104(4):328–336.
- Buelow DR, Anderson JT, Pounds SB, et al. DNA methylation-based epigenetic repression of SLC22A4 promotes resistance to cytarabine in acute myeloid leukemia. Clin Transl Sci. 2021 Jan;14(1):137–142.
- Drenberg CD, Gibson AA, Pounds SB, et al. OCTN1 is a high-affinity carrier of nucleoside analogues. Cancer Res. 2017 Apr 15;77(8):2102–2111.
- Burkhart JM, Vaudel M, Gambaryan S, et al. The first comprehensive and quantitative analysis of human platelet protein composition allows the comparative analysis of structural and functional pathways. Blood. 2012 Oct 11;120(15):e73–82.
- Uslu D, Abas BI, Demirbolat GM, et al. Effect of platelet exosomes loaded with doxorubicin as a targeted therapy on triple-negative breast cancer cells. Mol Divers. 2022 Dec 28. DOI:10.1007/s11030-022-10591-6
- Kodama T, Takehara T, Hikita H, et al. BH3-only activator proteins bid and bim are dispensable for Bak/Bax-dependent thrombocyte apoptosis induced by Bcl-Xl deficiency: molecular requisites for the mitochondrial pathway to apoptosis in platelets. J Biol Chem. 2011 Apr 22;286(16):13905–13913.
- Mason KD, Carpinelli MR, Fletcher JI, et al. Programmed anuclear cell death delimits platelet life span. Cell. 2007 Mar 23;128(6):1173–1186.
- Guo L, Shen S, Rowley JW, et al. Platelet MHC class I mediates CD8+ T-cell suppression during sepsis. Blood. 2021 Aug 5;138(5):401–416.
- Sinauridze EI, Kireev DA, Popenko NY, et al. Platelet microparticle membranes have 50- to 100-fold higher specific procoagulant activity than activated platelets. Thromb Haemost. 2007 Mar;97(3):425–434.
- Ferreira PM, Bozbas E, Tannetta SD, et al. Mode of induction of platelet-derived extracellular vesicles is a critical determinant of their phenotype and function. Sci Rep. 2020 Oct 22;10(1):18061.
- Maurer-Spurej E, Larsen R, Labrie A, et al. Microparticle content of platelet concentrates is predicted by donor microparticles and is altered by production methods and stress. Transfus Apher Sci. 2016 Aug;55(1):35–43.
- Nasiri S. Infusible platelet membrane as a platelet substitute for transfusion: an overview. Blood Transfus. 2013 Jul;11(3):337–342.
- Marcoux G, Magron A, Sut C, et al. Platelet-derived extracellular vesicles convey mitochondrial DAMPs in platelet concentrates and their levels are associated with adverse reactions. Transfusion. 2019 Jul;59(7):2403–2414.
- Rank A, Nieuwland R, Crispin A, et al. Clearance of platelet microparticles in vivo. Platelets. 2011;22(2):111–116. DOI:10.3109/09537104.2010.520373
- Papa AL, Jiang A, Korin N, et al. Platelet decoys inhibit thrombosis and prevent metastatic tumor formation in preclinical models. Sci Transl Med. 2019 Feb 13;11(479). DOI:10.1126/scitranslmed.aau5898
- Zhang L, Zhu Y, Wei X, et al. Nanoplateletsomes restrain metastatic tumor formation through decoy and active targeting in a preclinical mouse model. Acta Pharm Sin B. 2022 Aug;12(8):3427–3447.
- Johnson L, Lei P, Waters L, et al. Identification of platelet subpopulations in cryopreserved platelet components using multi-colour imaging flow cytometry. Sci Rep. 2023 Jan 21;13(1):1221.