ABSTRACT
Introduction
Whole blood samples, including arterial, venous, and capillary blood, are regularly used for disease diagnosis and monitoring. The global Covid-19 pandemic has highlighted the need for a more resilient screening capacity. Minimally invasive sampling techniques, such as capillary blood sampling, are routinely used for point of care testing in the home healthcare setting and clinical settings such as the Intensive Care Unit with less pain and wounding than conventional venepuncture.
Areas covered
In this manuscript, we aim to provide a overview of state-of-the-art of techniques for obtaining samples of capillary blood. We first review both established and novel methods for releasing blood from capillaries in the skin. Next, we provide a comparison of different capillary blood sampling methods based on their mechanism, testing site, puncture size, cost, wound geometry, healing, and perceptions of pain. Finally, we overview established and new methods for enhancing capillary blood collection.
Expert opinion
We expect that microneedles will prove to be a preferred option for paediatric blood collection. The ability of microneedles to collect a capillary blood sample without pain will improve paediatric healthcare outcomes. Jet injection may prove to be a useful method for facilitating both blood collection and drug delivery.
1. Introduction
The global Covid-19 pandemic has placed a significant burden on healthcare infrastructure, limiting capacity and access to routine diagnostic screening. An international review found a median reduction in diagnostic visits during the pandemic of 31 % [Citation1]. The analysis of blood samples is an essential tool in diagnosing and preventing disease. Most laboratory tests are performed on blood drawn from a vein or artery, requiring a healthcare professional to collect the specimen [Citation2]. However, the technological advancement of molecular analysis continues to reduce the sample volume needed for diagnostic testing [Citation3]. Moreover, a shift from a centralised healthcare model toward one that increasingly features decentralised elements will help fortify healthcare systems against significant disruption. The success of decentralisation has been demonstrated in point of care glucose measurements in the ongoing diabetes epidemic, made possible with capillary blood sampling by lancing.
Capillary blood sampling usually involves the use of a lancet to pierce the fingertip to a depth of up to 2.4 mm and produces a small sample for home-based or bedside point of care assays to measure blood clotting, anaemia, lipids, basic chemistry panels, etc [Citation4]. Capillary blood sampling is less invasive, less painful, and more accessible than venous or arterial sampling, and produces samples that are easy to handle and store. Capillary blood sampling can be performed on other sites on the body, such as the earlobe, forearm, heel, palm, and arm. The development of new technologies to reduce the pain associated with lancing will promote frequent testing for more effective screening and treatment of disease, reducing needle-phobia, and improving patient compliance [Citation5].
This article provides a broad review of minimally invasive capillary blood sampling methods and methods to enhance capillary blood collection. It is intended to support the development of new sampling and collection techniques, and ultimately facilitate decentralised healthcare options. The various techniques are discussed based on the mechanism, testing site, puncture size, skin reaction, pain, and examples of commercial products.
2. Methods for skin and capillary penetration
Several different methods have been developed for skin penetration and capillary blood release, including lancets, microneedles, laser, micro-particles, and liquid jets, as summarised in .
Table 1. Various methods for capillary blood sampling.
2.1. Lancets
Lancets are needles or blades used to puncture the skin and release capillary blood. The ‘fingerstick’ commonly used by diabetics is a widely-used example of a lancet-based device. The needle-type lancet tip has three bevelled faces () which keeps the force required to puncture tissue low [Citation6]. In contrast, the blade-type lancet is simply a small, double-edged surgical blade. Blade-type lancets produce a long shallow incision that induces less pain but produces a larger wound. The incision type is routinely used for obtaining blood samples from heels in the paediatric population of children less than 6 months of age, avoiding possible nerve damage in fingers by the puncture type [Citation4].
Figure 1. a – Tri-bevelled lancet tip. b – Commercially available lancet devices. c – Needle type lancet puncturing through tissue as in a conventional spring-loaded lancing pen.
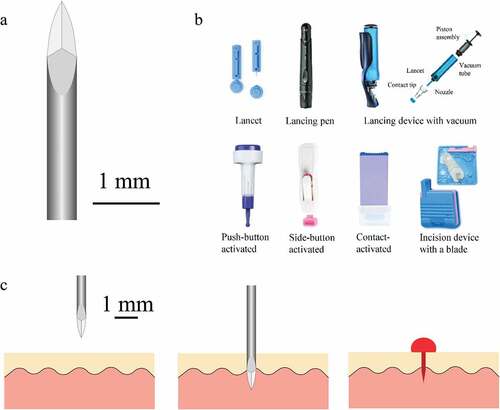
Automatic lancing devices () are designed to reduce pain while releasing sufficient blood volume. These devices automatically retract the lancet into the device after activation, reducing the risk of accidental needle stick injuries. Freehand lancets are associated with more tissue trauma [Citation7]. Less haemolysis is observed in blood samples acquired using an automated incision device than a manual lance [Citation8]. A pen-style automatic lancing device provides fine depth adjustment with replaceable lancets, whereas a single-use disposable lancing device has limited depth adjustment and reduces the risk of cross-contamination.
Most commercially available lancing devices use a spring-loaded stainless-steel needle lancet to penetrate less than 2.4 mm into the skin within milliseconds, releasing blood from the subpapillary venous plexus through the wound channel () [Citation4]. The patient then squeezes or milks the finger near the puncture site until a sufficient volume of blood is available. In general, capillary or venous blood pressure is sufficient to keep the wound channel open and release a blood sample of 3 μL to 5 μL from the puncture site for point of care tests, such as glucose measurement [Citation9].
2.1.1. Factors affecting blood volume and pain
The volume of blood released, and intensity of pain experienced, is positively correlated with lancet size and penetration depth [Citation10,Citation11]. Capillary blood sampling targets the subpapillary venous plexus to release a sufficient blood volume without penetrating deeper to the reticular dermis, where abundant nerve fibres are present. The released blood volume is also related to the type of the lancing device. Automatic incision lancing devices with blades release a larger volume of blood compared to automatic lancing devices with needles [Citation12,Citation13].
The lancing process is associated with pain as the lancet hits pain nerve receptors, in addition to small blood vessels in the skin. There is significant variability in pain sensitivity and skin thickness between individuals [Citation14] that, along with the choice of bodily location for lancing, give rise to a range of patient experiences. The pain experienced during blood sampling is a significant reason for poor patient compliance to regular finger stick testing regimes [Citation15,Citation16]. Many mechanical factors are linked to lancing pain: penetration depth, lancet size and geometry, lancing mechanism, noise, and activation method. The ideal lancing device will provide a sufficient blood volume to perform the blood test while minimising pain sensation.
2.1.2. Lancet size and geometry
Lancet diameter and geometry are related to pain sensation [Citation11,Citation17]. However, thicker lancets only induce more pain when they penetrate unnecessarily deep into the skin. For example, pricking with thicker lancets leads to no difference in pain at a depth of 0.9 mm but more pain at 1.2 mm, where a higher lateral density of nerves is present [Citation11]. Lancets have a pointed tip and three surfaces with two cutting edges to reduce the insertion force into tissue and associated pain [Citation18,Citation19]. A coating is often added to improve the surface finish and reduce the friction between lancet and tissue during penetration [Citation20].
2.1.3. Lancet motion
Characteristics of lancing motion, such as trajectory, vibration, and speed, are important factors directly affecting lancing pain [Citation16]. There are three actuation methods used to lance the skin: linear actuation, cam actuation, and electronic actuation [Citation16]. Linear actuation is used in conventional lancing devices. One spring releases the lancet into the skin, and the other springs retract the lancet back to its original position. Such automated lancing methods can improve sample quality by reducing haemolysis [Citation21]
The best-performing lancing devices on the market are cam-driven and rail-guided, providing a smoother actuation [Citation17]. Such lancing devices reduce vibration, lateral motion, and repetitive penetration of the lancet in the tissue. They are shown to reduce pain and increase blood volume compared to conventional lancing devices [Citation22]. The smoother gliding mechanism also reduces noise accompanying the firing of the device, reducing any upset evoked from the patient, which decreases pain [Citation22].
Electronic-actuated lancing devices use a small electronic motor (e.g. voice coil, solenoid) coupled with a position sensor to allow for precise movement of the lancet [Citation17]. Electronic devices actuate lancets at a higher speed (2 m/s – 10 m/s) than mechanical or spring-loaded devices (1 m/s – 6 m/s) [Citation23]. High speed lancing results in lower skin-piercing force and smaller puncture wounds, facilitating quick wound closure and reducing lancing pain [Citation20,Citation24]. However, the complexity of such electronic devices substantially increases the size and cost (USD 250 for Pelikan Sun, discontinued).
2.1.4. Lancet release trigger
Lancing pain is also influenced by activation methods: push button, side button, and contact activation [Citation25]. Mechanical push-button activated lancing devices result in higher pain levels than contact-activated devices [Citation25,Citation26]. Side-button and contact activated lancing devices induce similar levels of pain. Push-button activated lancing devices provide larger blood samples than contact activated devices, while side-button and contact activated lancing devices supply blood samples of similar volume [Citation25].
2.1.5. Lancet wound
A needle-type lancet generates a straight cut following skin penetration [Citation27]. The crack faces are wedged open by the advancing punch and closed after removing the punch, resulting in a planar crack [Citation27]. A ‘C-shaped’ wound in the skin has also been reported due to the faceted cutting edges of the lancet [Citation28]. Bruising and erythema have been observed at the lancet wounds on the fingertip and other body sites, with 80 % of the lancing wounds healed after three days [Citation29].
Automatic lancing devices with blades cut across the skin surface, leading to longer but shallower slices than conventional manual lancets. For example, a Tenderfoot Preemie automatic incision device with a blade creates a wound with a depth of 0.85 mm and a length of 1.75 mm, whereas a conventional manual lancet has a blade length of 2.4 mm. The longer but shallower wounds allow access to a sufficient number of capillaries while avoiding disruption of deeper nerve endings. The automatic incision device (such as Tenderfoot Preemie) cause less bruising and damage to the heels of premature infants than a conventional manual lancet [Citation21,Citation30].
2.2. Microneedles
Microneedles or micron-sized needles were first developed for transdermal drug delivery in 1976 and have become the subject of significant research since the 1990s [Citation31]. They penetrate the stratum corneum and the underlying epidermis, leading to microscopic pores for drug diffusion into microcirculation, while avoiding nerve contact. Sensors have been integrated within microneedles to analyse both blood and interstitial fluid [Citation32]. This has led to the recent development of systems that can provide continuous transdermal monitoring and therapeutic delivery [Citation33,Citation34]. Lancet pricks are stigmatised by pain, needle-phobia, and the spread of blood-borne pathogens by needle reuse and disposal. Microneedles are less intimidating compared to hypodermic needles and thus lead to reduced psychological stress and increased patient compliance.
2.2.1. Solid microneedles
Solid microneedles are the simplest type of microneedle designed to perforate the skin. Once inserted, they are then retracted to uncover the perforated tissue (). The perforation increases the permeability of skin to fluid and drugs, along with potentially breaking capillaries beneath. In contrast, dissolvable microneedles are designed to remain embedded in the tissue while they are slowly absorbed, which is useful for drug delivery. Transdermal drug delivery using microneedles reduces pain and risk of infection, and increases patient compliance [Citation35].
Figure 2. a – Schematic of skin penetration with an array of solid microneedles for blood release. b – Schematic of hollow microneedle array penetrating tissue. c – SEM of an array of 500 µm tall conical microneedles. Figure reproduced with permission from [Citation33] d – Solid microneedle array with two electrodes for sensing. Figure reproduced with permission from [Citation33].
![Figure 2. a – Schematic of skin penetration with an array of solid microneedles for blood release. b – Schematic of hollow microneedle array penetrating tissue. c – SEM of an array of 500 µm tall conical microneedles. Figure reproduced with permission from [Citation33] d – Solid microneedle array with two electrodes for sensing. Figure reproduced with permission from [Citation33].](/cms/asset/2793ed4a-d41b-45eb-8a69-f6b6905bff5e/ierd_a_2170783_f0002_oc.jpg)
The perforation of the epidermis or dermis produces a local pressure gradient that forces interstitial fluid or blood into the holes and up to the skin surface (). This blood flow can be enhanced and directed by vacuum into a collection device using an integrated micro-pump [Citation36] or the capillary effect [Citation37]. Blood can be both extracted and stored in microgrooves near the substrate of the microneedle [Citation38]. The cost for such a microneedle array is approximately $1 [Citation39].
The first commercial microneedle-based blood sampling device (TAP Blood Collection System, Seventh Sense Biosystems) passed US Food and Drug Administration clearance in 2019 for layperson use. This one-step, fully-automated, and low-cost capillary blood collection device has been developed to simplify the workflow of finger-stick sampling [Citation5]. The device penetrates skin using a ring of 30 solid microneedles which has a high probability of rupturing blood vessels and can collect 100 µL of blood. The next generation of the device, the TAP II (YourBio Health Inc.) is at a clinical trial stage and looks to improve the device performance and measurement processing pipeline.
2.2.2. Hollow microneedles
Hollow microneedles are characterised by the existence of channels through the microneedle array which provide a convenient path for fluid flow () for the extraction of blood and interstitial fluid. A sample of whole blood has been extracted painlessly, by blood pressure alone, from the capillaries in the back of the hand following the application and removal of a microneedle array [Citation40]. Hence, hollow microneedles have frequently been investigated for biosensor integrated devices to enable point of care measurement [Citation41,Citation42]. Hollow microneedles typically rely on capillary action to draw blood into and through the fluid channels [Citation43]. They are often made with polymer coatings to improve biocompatibility of microneedles [Citation44].
2.2.3. Design
The size of microneedle devices depend on the type of microneedle, the material used, and the intended application. Appropriate choice of geometry and material is essential to achieving high ratios of failure-force to insertion-force for efficient skin penetration [Citation45]. Microneedles are typically 50 µm to 250 µm wide and 150 µm to 1500 µm long, as the epidermis thickness is less than 1500 µm [Citation46]. Blood vessels are present at depths near 1000 µm [Citation47];penetration beyond this level is associated with increased stimulation of pain [Citation5].
The tips of the microneedles are usually 1 µm to 25 µm thick and can be constructed with various shapes, including cylindrical, triangular, pointed, and pentagonal [Citation46]. Tissue may block the conduit of a hollow microneedle during penetration [Citation48]. To combat this, the conduit can be fabricated on the side of a microneedle to prevent tissue blockage [Citation37,Citation49].
A range of materials, including polymers, stainless steel, titanium, nickel, and silicon, have been investigated as substrates for microneedle fabrication [Citation50]. Polymers play an important role in fabricating microneedles as they can be biodegradable, have high mechanical strength, and low cost. Polymer-based microneedles enhance resistance to shear-induced breakage due to the viscoelasticity of polymers. Some metal microneedles are coated with polymers such as parylene to improve biocompatibility but still risk breakage in the skin [Citation42]. Metals are typically chosen for their strength and ability to resist fracturing when inserted into the skin. Silicon is often an appealing option as the technology to mass produce silicon microstructures is well established.
In-plane silicon-processed microneedles with fluid channels have been under development since the early 1990s [Citation51]. Such microneedle arrays or patches were developed to distribute normal and shear forces, facilitating smoother punctures and reducing the risk of needle fracture [Citation43]. An arm stick using a 2 mm long and 100 µm thick silicon microneedle was less painful than a fingerstick with a lancet (Smart & Subramanian, 2000).
2.2.4. Actuation mechanism for penetration
Most microneedle arrays are applied manually to the skin and rely on the application of force to the back of the patch to ensure even penetration across the patch. Manual insertion is unreliable in achieving complete, uniform insertion due to the viscoelastic behaviour of the skin at low insertion velocities [Citation52]. Several actuation methods have been explored to provide automated and repeatable microneedle penetration. Shape memory alloy actuators recover their pre-deformed shape by the electrical heating of a shape memory alloy spring [Citation36]. Voice coil electromagnetic actuators provide higher piercing force and insertion depth than shape memory alloy actuators with the same driving voltage [Citation53]. The vibration of microneedles by a piezoelectric actuator effectively reduces the insertion force and tissue displacement and can be incorporated with microneedles for efficient insertion [Citation54]. MEMS-based microneedle arrays with shorter microneedles have been under development for minimally invasive blood sampling by several research groups since the early 2000s [Citation43,Citation55,Citation56]
There has been significant research effort recently to improve the ease and comfort of penetration, including the design of microneedles for blood extraction inspired by arthropods, especially mosquitoes. The proboscis of a mosquito consists of a central labrum that pierces into the skin and a surrounding labium that provides lateral support. The labrum is comprised of multiple elements that move individually at different phases, producing vibratory reciprocating motions which advance incrementally in the tissue [Citation57,Citation58]. Micro-actuators have been developed in order to mimic the penetration of a mosquito’s proboscis [Citation59]. Fabrication of structures emulating the labrum and surrounding structures has been demonstrated [Citation60]. Work remains to be done to reinforce the structures to avoid buckling when attempting to pierce human skin.
2.3. Laser lancing
A short high-power laser pulse can be used to release blood from the fingertip by ablating the skin tissue, creating a small hole (). Erbium: yttrium-aluminium-garnet (Er:YAG) laser emission (at 2940 nm) is strongly absorbed by water in the tissue and has been used to disrupt blood vessels in the dermis. Laser lancing devices eliminate the need for conventional lancets and provide protection from the transmission of blood-borne diseases [Citation61].
Figure 3. a – Schematic of laser lancing wherein tissue is ablated to create a puncture. b – Schematic of liquid jet-injection wherein a high velocity water jet penetrates into tissue. c – Schematic of liquid jet-injection followed by a retraction of the piston in order to suck blood drawn into the ampoule.
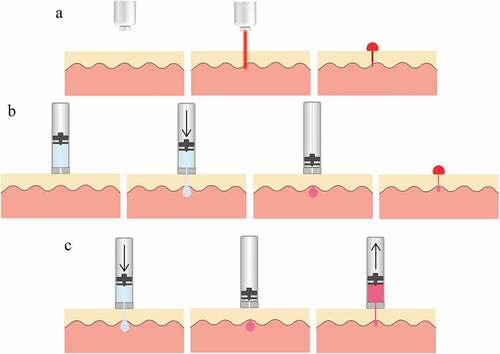
A portable pulsed Er:YAG laser (Venisect Inc, Little Rock, AR) was developed to obtain a blood sample for subsequent point of care testing, and successfully collected sufficient blood in 97 % of samples [Citation62]. The Laser Lancet (TransMedica International, Little Rock, AR) creates a 0.25 mm by 1.1 mm slit through the stratum corneum on the fingertip. The healing rate of the skin punctures using this device was no less than that with conventional lancets [Citation63]. Pain perceived from this method is dependent on the power of the laser beam.
Two clinical studies in 2021 demonstrated that the LMT-1000 (LaMeditech Co., Ltd, Seoul, Korea) laser lancing device induces less pain than a conventional lancet prick of the fingertip, while delivering the same point of care test results (pH, CO2, O2, lactate, and haematocrit levels), capillary haemoglobin and blood typing results [Citation64,Citation65]. The odour of burning skin during ablation, and longer healing time due to the cauterisation of blood vessels, may impede the adoption of the laser lancet [Citation16].
2.4. Liquid jet
Needle-free jet-injection is a well-developed technique for liquid drug delivery into skin tissue: a narrow, high-speed liquid jet will readily pierce the skin and penetrate many millimetres into the underlying tissue () [Citation66]. An electronically-actuated jet injector provides dynamic control over injection pressure and can be used to inject liquid to a controlled depth [Citation67]. The control over injection depth makes the jet injector a versatile injection system capable of serving multiple functions.
A 2021 study trialled the use of both circular and slot-shaped fluid jets for blood release from volunteers’ fingertips [Citation68]. This study demonstrated that sufficient blood could be retrieved from the fingertip to conduct a glucose measurement, with no difference in skin reactions at the intervention site relative to a lancet prick [Citation68]. Jet injections performed with slot-shaped nozzles mimicked the wound induced by the lancet perforation [Citation69,Citation70]. However, in this trial, the volume of blood released after jet penetration was less than after lancing, and was more painful in some cases.
Further development of this technology may enable both blood specimen collection and drug delivery from a single device. Any samples collected will contain some portion of injectate fluid mixed within, so this dilution will need to be measured or otherwise accounted for in any measurements ().
3. Methods for enhancing blood release from capillaries
The average volume of blood released by a typical finger lancing is 3.1 µL [Citation71]. This leaves little room for mistakes in collecting the 0.5 µL to 1 µL required for a glucose test strip. As the density of capillaries is lower in non-fingertip sample sites (so-called ‘alternate sites’) the volume of blood released by lancing at these sites is even lower. These factors encourage the adoption of techniques to increase the blood sample volume from lancing, thereby decreasing the risk of a failed measurement.
Blood release enhancing techniques aim to increase the volume of blood delivered through broken capillaries to the site of skin penetration. Such techniques may also enable less painful methods that would otherwise release insufficient blood volume to be adopted. Blood flow through capillaries is controlled by upstream myogenic regulation of arterioles that constrict and dilate in response to neuronal stimuli [Citation72]. Physical manipulation of the tissue is the most prevalent method, applying pressure in the surrounding region so as to ‘milk’ it for blood.
3.1. Tactile
It is common to apply pressure proximal to the fingertip to promote blood flow. This is believed to both redistribute blood towards the fingertip and occlude veins that would drain blood back from the fingertip. Rubbing the lancing site, particularly in the case of alternate site sampling, is commonly recommended [Citation73]. Such stimulation will often result in a visible redness to the region, indicating blood pooling in capillaries. The AtLast Blood Glucose monitoring system (Amira Medical, Scotts Valley, CA) incorporates a stimulator ring that presses against the skin surface surrounding the puncture site to promote blood flow [Citation74]. The initial depression of the system triggers the lancing and squeezes the skin area around the wound. The stimulator ring helps achieve consistent penetration depth, keeping the skin stretched and wound open for blood release and forcing blood to the surface by milking the lanced area. By introducing vibration to the skin surface a needle lance can penetrate deeper, with less force () [Citation75].
Figure 4. a – (left) Lateral stretching of skin resulting in reduced tissue deformation when lancing. (right) Vibration of skin introduced by a stimulator ring. b – Schematic of the application of suction to the lancing site in order to increase the volume of blood drawn.
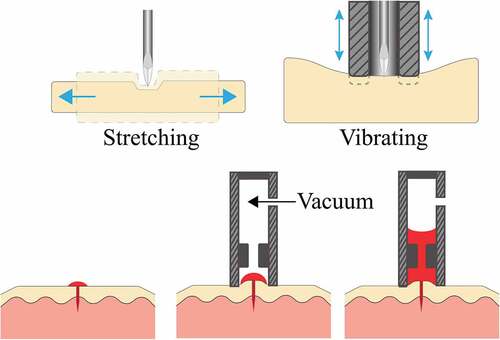
The shape of the device brought into contact with the skin influences the skin’s tension and strain, and thereby the volume of blood collected. A nosepiece is often used to interface between skin and lancing device to provide a constant standoff distance. A parametric analysis of the nosepiece diameter demonstrates that blood volume collected from a simulated lancing site increases as the diameter widens until an optimal 14 mm is reached for the nosepiece diameter [Citation74]. As the nosepiece diameter increased from 4 mm to 10 mm, the skin elevation and the volume of blood extracted both increased [Citation76]. The height of skin stretching into the nosepiece (from 1.6 mm to 6 mm) is also positively correlated with the volume of blood collected.
3.2. Vacuum
Applying vacuum over the lanced site leads to increased blood release (). The extent of vacuum and the length of time over which it is applied are positively correlated with the volume of blood released. The Microlet Vaculance Lancing device (Bayer, West Haven, CT) uses a plunger mechanism to actuate the lancet to pierce the skin and create a vacuum around the puncture site to draw blood to the skin’s surface [Citation74]. Stretching the skin up into the nosepiece by applying a vacuum before lancing results in a larger volume of blood extracted from the lancet wound [Citation76]. The application of vacuum before and after lancing the forearm, combined with skin stretching, can collect approximately 16 µL of blood in 30s [Citation76]. This technique induces vasodilation and an up to fivefold increase in blood perfusion. With the application of vacuum, redness and bruising were experienced in some cases by the participants but were resolved in a few days [Citation76]
The drawbacks of such techniques include blood splatter when the device is removed under a vacuum, difficulty forming a vacuum on hairy regions, and the presence of welts and bruising from the suction. The SoftTact Diabetes Management System (Abbott Laboratories, MediSense Products, Bedford, MA) overcomes some of these drawbacks, combining the lancing device and meter into one automated device for alternative site testing [Citation77]. The system automatically pricks the skin, extracts blood from the puncture site, and draws blood onto the strip by applying a vacuum.
Due to the flow constraints of microneedle geometry, suction is required to collect a blood sample in a timely manner. Such approaches can collect capillary blood volumes in the order of 100 µL within 3 minutes [Citation5]. Vacuum has the additional benefit of being able to automatically direct the released blood into storage, suitable for subsequent laboratory analysis.
3.3. Vasodilation
Vasodilating agents are commonly applied to the sampling region to collect arterialised capillary blood from sites such as an earlobe for blood gas measurement. Agents such as menthol significantly increase perfusion 15 minutes after application [Citation78]. This method is practically restricted to a limited set of cases where a patient is willing to apply a cream in anticipation of collecting a capillary sample.
Local vibration of the dermis introduces shear stresses within blood vessels stimulating the release of the vasomodulator nitric oxide (NO) [Citation79–82]. NO-mediated vasodilation produces a rapid response in skin perfusion, increasing perfusion by up to fivefold in a matter of seconds. The frequency of vibration used commonly ranges between 26 Hz and 50 Hz. This increase in perfusion similarly dissipates in the order of seconds following the cessation of vibration. The benefit of localised vibration on capillary blood sampling has yet to be explored in a direct study.
Heating the skin to around 40°C or 45°C before lancing releases more blood after lancing, at the expense of sampling duration and complexity of the apparatus (Young et al., 2013). The skin circulation increases to facilitate heat loss in hot conditions, promoting a larger volume of blood released from the lancing site (Young et al., 2013). Despite this, a study of the use of warming to assist in neonatal heel pick sampling failed to find any benefit [Citation83]. Cooling the skin can cause hypothermia-induced reversible platelet dysfunction, increasing bleeding time [Citation84]. This effect was observed from blood drawn from forearm tissue when skin temperature was brought to and held at 22°C. These conditions allow for a greater total volume collected if the patient is willing to spend up to 3 minutes collecting a blood sample.
3.4. Sampling location
The fingertip is often the default site for capillary blood sfampling for point of care diagnosis but can be quite sensitive to pain. Analyte concentrations in capillary blood are between those in arterial and venous blood. Rapid shifts in arterial blood glucose concentration in relation to meals or exercise may only be reflected in capillary measurements after a significant time delay. Blood sampled from a fingertip can reflect this change, whereas in alternate sites such as the forearm, thigh, and shoulder there is a significant time delay [Citation85,Citation86].
Alternative sites vary in tissue structure and characteristics, such as nerve sensitivity, capillary density, and proximity to arterial supply. Unlike fingertip capillary sampling, earlobe sampling is a suitable replacement to arterial sampling for measuring blood gases [Citation87]. The palm of the hand is a less sensitive but comparably accurate capillary sampling site [Citation88,Citation89]. The arm and leg are common alternative sites to fingertips to minimise pain. Supportive techniques, such as vacuum-assisted blood release, are often required to ensure sufficient blood release at alternate sites due to the lower capillary density relative to fingertips.
4. Methods for collecting blood
4.1. Passive extraction techniques
Passive extraction techniques rely on capillary action to draw blood into a storage vessel for transport and analysis, usually resulting in less blood volume than active extraction techniques (). Blood flow under capillary action can be enhanced by adding a surface hydrophilic coating and a wire to guide blood [Citation90]. Microneedle arrays have been fabricated with integrated sensing capabilities to perform analyses in situ [Citation47,Citation91].
4.2. Active extraction techniques
Active extraction techniques involve providing an eternal driving force to facilitate collection of the blood sample and direct blood flow into a storage vessel. The most common method is to apply a vacuum from the storage reservoir (). In this way, the same vacuum source that is used to enhance blood release can drive the collection into an appropriate reservoir. Microfluidic pumps have also been fabricated from piezoelectric micro-actuators [Citation36] and by directly applying a voltage across a channel [Citation92]. Simplifying actuator design and the number of external support instruments, such as batteries, heaters, and valves, is necessary to lower manufacturing costs and increase user convenience.
Blood can be extracted into a reservoir after a puncture with a single/array of microneedles by both passive and active fluidic manipulation systems. After lancing, wet blood samples are collected for subsequent biochemical tests by applying positive pressure, capillary forces, and vacuum. For example, Innovac Quick-Draw device (Innovative Medical Technologies, Lenexa, Kansas), Tasso-SST (Tasso Inc, Seattle, WA) and SofTact™ (Abbott Laboratories/MediSense Products, Bedford, MA) use a lancet to puncture and withdraw blood under vacuum into a collection tube.
4.3. Dried blood spots (DBS)
The dried blood spots (DBS) technique has been used for more than 40 years, providing convenient and low-cost home sampling. Following a lancet prick, capillary blood is directly applied to special filter paper. The dried sample, which is robust for shipping and storage, is then sent for laboratory tests by mail. The sample is then sub-punched and extracted with an aqueous or organic solvent for analysis [Citation93]. The DBS method typically produces interchangeable results with venous blood sampling [Citation94]. Neonatal diabetes can be diagnosed by measuring glucose in a dried blood spot collected on day 5 of life [Citation95].
Volumetric absorptive microsampling (VAMS) is a more advanced DBS sampling method for the remote collection of capillary blood. VAMS overcomes the spot area bias and homogeneity issues associated with the conventional DBS method [Citation93]. The hydrophilic polymeric tips of VAMS samplers use capillary action to wick up a fixed volume blood sample (less than 50 µl) into a porous substrate, regardless of the haematocrit, in less than 4 seconds [Citation93]. The blood volume absorbed is determined by the properties and amount of substrate. The VAMS sample is dried for 2 hours at room temperature and then sent via mail to a central laboratory for analysis. The VAMS sampler can be integrated into automated bioanalytical procedures in the laboratory. This simple, user-friendly dried blood collection approach reduces analysis costs, the number of clinical visits, and may be used to monitor study drug adherence.
Other than its use in therapeutic drug monitoring, VAMS has been used in COVID-19 clinical trials due to its ease of sampling, storage, and delivery [Citation96]. VAMS method has been reported to meet the accuracy and precision criteria of analysis in several bioanalytic studies. Sample imprecision increases as the specimen is dried prior to analysis due to degradation of haemoglobin [Citation97].
5. Conclusions
Lancing is a reliable, inexpensive and simple procedure for collecting a capillary blood sample. But as the prevalence of undiagnosed diseases and chronic conditions such as diabetes are on the rise, the development of less invasive sampling options is essential. As new options become available, each with its own strengths, it is important to select a technology appropriate to its use case.
Microneedles can be distributed in large quantities relatively inexpensively, and offer the ability to collect samples painlessly, although a longer sampling time is required. However, blood sampling using microneedles is still predominantly at the research stage. The cost per sample of microneedles is presently an order of magnitude above that of a lancet (). This may achieve parity as manufacturing techniques develop and reach scale.
The combination of active manipulation and smaller features of microneedles should enable robust painless capillary blood collection. The cost-effectiveness of employing active manipulation in applying the single-use microneedle arrays has yet to be demonstrated. If the active components can be reused, their incorporation may not substantially increase the cost of distributing the system at scale.
Microparticle, laser, and jet injection technologies offer needle-free sampling on a similar time scale to lancing. The cost and complexity of the needle-free systems may mean they are better suited to a centralised healthcare setting. In a high throughput setting, needle-free techniques can reduce medical waste and improve the sustainability of medical care. Blood sampling using microparticles is still nascent in development and requires more information about the cost, safety, and level of pain compared to other methods. Laser and jet injection have similarly high unit costs. Jet injectors have a broader use case than laser methods as the same jet injectors may be able to be used for drug delivery.
The reversibility of electronically-controlled jet injection devices may allow the application of a vacuum to the skin to extract blood into an integrated biosensor. Methods to quantify the dilution of the sample collected may be required depending on the intended analysis of the sample due to dilution by the fluid injected. This technique has the potential to enable blood sampling, biochemical testing (e.g. glucose), and drug delivery (e.g. insulin) to be combined into a single device. The injection speed can also be controlled to target a specific injection depth, or to account for variation in patient skin thickness. This makes the system much more robust in being able to deliver a consistent injection across a wide range of conditions without producing sharps waste. Despite these capabilities of controllable, reversible jet injectors, research into these devices for blood sampling is still at an early stage and they are still some ways from delivering a cost-effective solution for capillary blood sampling.
Use of a topical vasodilator is standard practice when collecting a sample for blood gas measurement. However, this approach is unlikely to be adopted for widescale routine sampling. Thermal methods similarly extend the sampling process to be much less convenient for the patient. The required complexity to automatically apply either method would likely be unwarranted compared to the option to provide patient instructions such as, to apply a warm wet cloth. Such an approach would likely have high compliance for low frequency or one-off sampling.
Alternative sampling sites have been explored considerably by the diabetic community. Typically, the inconvenience of accessing such sites for frequent testing, along with probability of blood stains on clothes, outweighs the benefits of reduced pain. However, the infrequent or one-off patient collecting a sample is likely to prioritise the reduction of pain. The targeting of alternative body sites for sampling does come at the cost of blood volume collected as compared to fingertip sampling. There is also a difference in blood composition that can be significant, depending on the intended analysis.
For most patients who must frequently sample their blood, it is important that any method be quick, cheap, and easy. For various reasons, diabetics will often sample infrequently and reuse lancets contrary to best practice. Systems that apply a vacuum after blood release act within the existing timeframe of capillary sampling. Single use systems that integrate a lancet or microneedle array with a sealed vacuum reservoir provide simplicity of use at the cost of unit complexity and price. This is unlikely to suit a diabetic patient, but would be quite suited to the patient who requires less frequent tests. An alternative reusable system that instead generated the suction at the point of sampling would require more patient skill and device cost, but may achieve lower cost per sample for the frequent user. This makes a suction-based method to enhance blood volume collected a suitable approach that merits further development.
6. Expert opinion
A lancet prick is the current standard method for capillary blood sampling. However, promising alternatives such as microneedles, jet injection, and lasers offer the potential to avoid the pain, needle-phobia, and reduce the spread of blood-borne pathogens associated with lancing. Microneedle arrays offer a way to painlessly collect blood samples in an outpatient setting, without the need of a trained phlebotomist. As it stands, lancing will likely remain the standard method for diabetes management due to the short time required to collect sufficient blood for a glucose test.
A main barrier to further adoption of these blood sampling techniques is to overcome the patient aversion associated with discomfort. A promising path to reducing pain is to shift sampling to sites other than a fingertip, although often associated with lower blood volumes. Ensuring consistent blood volumes is essential for reliable laboratory test results, thus robust methods to enhance blood release are necessary. At present, questions remain as to what, if any, relationship exists between the composition of blood drawn from the sampling location. Additionally, it is crucial to understand the variability of blood composition between samples. Significant advancements have been made in methods to improve the release and collection of capillary blood samples.
The application of suction to assist in the extraction and collection of blood has been shown a robust and effective method to enhance the blood volume collected. Such supporting techniques may allow for the shift of capillary blood sampling away from the sensitive fingertip and utilising less invasive techniques. The consideration of patient compliance is essential when evaluating methods to enhance blood release. If adoption on a meaningfully wide scale is desired, then the combined sampling method along with blood collection should be no more cumbersome than current methods. Incorporation into an automated and repeatable sampling device has been demonstrated in a range of commercial devices. In this way, routine venepuncture may soon be replaced with painless, at-home, capillary blood sampling that can then be delivered to a laboratory for analysis.
Direct blood sampling will continue to be an important diagnostic and therapeutic tool for the foreseeable future. The development of minimally invasive blood sampling methods will improve patient outcomes as developments allow more analyses to be performed with diminishing volumes of blood. More frequent, lower volume blood analysis can be widely incorporated into patient care allowing for earlier diagnosis and evaluation of intervention efficacy. The main obstacle to this future is developing institutional confidence in the results of analyses made on blood collected in this way.
Large scale screening programs will be impractical as long as blood collection is associated with pain and requires a trained phlebotomist. Developing methods to painlessly collect a blood sample, and robust measurement techniques to reliably draw inferences from reduced sample sizes will allow for more informed patient healthcare. In the near future, we will see microneedle patches used in paediatric wards, along with needle free options being offered in healthcare centres. This is a step towards a more equitable future which improves public access to healthcare, while freeing up the labour often required for blood sampling. The continued move towards personalised medicine will develop an environment in which blood samples can be collected at home and be reliably delivered to a laboratory.
Article highlights
The capabilities of capillary blood sampling rivals those of venous sampling in many regards.
New skin penetration techniques such as microneedles, liquid jets, and lasers are being developed that offer several advantages over the standard practise of lancing.
Vaccuum and other methods for enhancing capillary blood release have the potential to improve the consistency, ease, and comfort of sampling.
Of the many methods to collect a capillary blood sample, microneedles offer the most reliably pain free patient experience.
Continued development of minimally invasive methods will help improve health outcomes.
Declaration of interest
Andrew J. Taberner has a minority interest in medical device company Portal Instruments, Inc. The authors have no other relevant affiliations or financial involvement with any organization or entity with a financial interest in or financial conflict with the subject matter or materials discussed in the manuscript. This includes employment, consultancies, honoraria, stock ownership or options, expert testimony, grants or patents received or pending, or royalties.
Reviewers disclosure
Peer reviewers on this manuscript have no relevant financial relationships or otherwise to disclose.
Additional information
Funding
References
- Moynihan R, Sanders S, Michaleff ZA, et al. Impact of COVID-19 pandemic on utilisation of healthcare services: a systematic review. BMJ Open. 2021;11(3):e045343.
- Dincer C, Bruch R, Kling A, et al. Multiplexed point-of-care testing – xPOCT. Trends Biotechnol. 2017;35(8):728.
- Lei BUW, Prow TW. A review of microsampling techniques and their social impact. Biomed Microdevices. 2019;21(4). Doi: 10.1007/s10544-019-0412-y.
- WHO guidelines on drawing blood: best practices in phlebotomy WHO library cataloguing-in-publication data. Geneva, Switzerland: Printed by the WHO Document Production Services; 2010.
- Blicharz TM, Gong P, Bunner BM, et al., Microneedle-based device for the one-step painless collection of capillary blood samples. Nat Biomed Eng. 2018;2(3):151–157. .
- Meyer CH, Kaymak H, Liu Z, et al. Geometry, penetration force, and cutting profile of different 23-gauge trocars systems for pars plana vitrectomy. Retina. 2014;34(11):2290–2299.
- Merter OS, Bolişik ZB. The effects of manual and automatic lancets on neonatal capillary heel blood sampling pain: a prospective randomized controlled trial. J Pediat Nurs [Internet]. 2021 [cited 2022 Jul 21];58:e8–e12. Doi: 10.1016/j.pedn.2020.11.015.
- Kazmierczak SC, Robertson AF, Briley KP. Comparison of hemolysis in blood samples collected using an automatic incision device and a manual lance. Arch Pediatr Adolesc Med. 2002;156(11):1072–1074.
- Feldman B, McGarraugh G, Heller A, et al. FreeStyle ™: a small-volume electrochemical glucose sensor for home blood glucose testing. Diabetes Technol Ther. 2000;2(2):221–229.
- Fruhstorfer H, Müller T, Scheer E. Capillary blood sampling: how much pain is necessary? Part 2: relation between penetration depth and puncture pain. Practical Diabet Int. 1995;12(4):184–185
- Fruhstorfer H, Schmelzeisen-Redeker G, Weiss T. Capillary blood sampling: relation between lancet diameter, lancing pain and blood volume. Eur J Pain. 1999;3(3):283–286.
- Serafin A, Malinowski M, Prażmowska-Wilanowska A. Blood volume and pain perception during finger prick capillary blood sampling: are all safety lancets equal? Postgrad Med. 2020;132(3):288–295.
- Folk LA. Guide to capillary heelstick blood sampling in infants. Adv Neonatal Care. 2007;7(4):171–178
- Fruhstorfer H, Abel U, Garthe CD, et al. Thickness of the stratum corneum of the volar fingertips. Clin Anat. 2000;13(6):429–433.
- Kim S. A pain-free lancet with a small needle for glucose measurement. Clin Med Insights Endocrinol Diabetes. 2010;3:1.
- Heinemann L, Boecker D. Lancing: quo vadis? J Diabetes Sci Technol. 2011;5(4):966–981.
- Kocher S, Tshiananga JKT, Koubek R. Comparison of lancing devices for self-monitoring of blood glucose regarding lancing pain. J diabet Science Technol. 2009;3(5):1136–1143
- Kirk JK, Stegner J. Self-monitoring of blood glucose: practical aspects. J Diabetes Sci Technol. 2010;4(2):435–439.
- Qi Y, Jin J, Chen T, et al. Modeling of geometry and insertion force of a new lancet medical needle. Sci Prog. 2020;103(1):1–19.
- Chang H, Yeh Y-J, Lee R, et al. A feature study of innovative high-speed lancing device and safety lancet. Australas Phys Eng Sci Med Internet]. 2016;39(4):895–902
- Vertanen H, Fellman V, Brommels M, et al. An automatic incision device for obtaining blood samples from the heels of preterm infants causes less damage than a conventional manual lancet. Arch Dis Childhood-Fetal Neonatal Ed. 2001;84(1):F53–F55.
- Grady M, Lamps G, Shemain A, et al. Clinical evaluation of a new, lower pain, one touch lancing device for people with diabetes: virtually pain-free testing and improved comfort compared to current lancing systems. J Diabetes Sci Technol. 2021;15(1):53–59.
- Fritz M, Argauer H, List H, et al. Blood lancet with hygienic tip protection. Google Patents; 2003.
- Fruhstorfer H. Capillary blood sampling: the pain of single‐use lancing devices. Eur J Pain. 2000;4(3):301–305.
- Jarus-Dziedzic K, Zurawska G, Banys K, et al. The impact of needle diameter and penetration depth of safety lancets on blood volume and pain perception in 300 volunteers: a randomized controlled trial. J Med Lab Diagn. 2019;10(1):1–12.
- Warunek D, Stankovic AK. Evaluation of lancets for pain perception and capillary blood volume for glucose monitoring. Am Soc Clin Lab Sci [Internet]. 2008 [cited 2022 Oct 2];21:215–218. Available from http://clsjournal.ascls.org/content/21/4/215
- Shergold OA, Fleck NA. Mechanisms of deep penetration of soft solids, with application to the injection and wounding of skin. Proceedings of the Royal Society of London Series A: mathematical. Phys Eng Sci. 2004;460:3037–3058. Internet]. [cited 2022 Jul 21]. Available from:https://royalsocietypublishing.org/doi/10.1098/rspa.2004.1315
- Verdonk ED, Lum P. Lancet device with skin movement control and ballistic preload. U.S.: Google Patents; 2001.
- Cunningham DD, Henning TP, Young DF, et al. Wound healing after lancing the skin. Wounds A Compendium Clin Res Pract. 2000;12:131–137.
- Kellam B, Waller J, McLaurin C, et al. Tenderfoot preemie vs a manual lancet: a clinical evaluation. Neonatal Network. 2001;20(7):31–36.
- Kim Y-C, Park J-H, Prausnitz MR. Microneedles for drug and vaccine delivery. Adv Drug Deliv Rev. 2012;64(14):1547–1568.
- Madden J, O’Mahony C, Thompson M. Biosensing in dermal interstitial fluid using microneedle based electrochemical devices. Sensing and Bio-Sensing Research. 2020 Doi:10.1016/j.sbsr.2020.100348.
- Liu Y, Yu Q, Luo X, et al. Continuous monitoring of diabetes with an integrated microneedle biosensing device through 3D printing. [cited 2022 Dec 12]; www.nature.com/micronano.
- Luo X, Yu Q, Liu Y, et al. Closed-loop diabetes minipatch based on a biosensor and an electroosmotic pump on hollow biodegradable microneedles. 2022 [cited 2022 Dec 12]; Doi:10.1021/acssensors.1c02337.
- Hegde NR, Kaveri SV, Bayry J. Recent advances in the administration of vaccines for infectious diseases: microneedles as painless delivery devices for mass vaccination. Drug Discov Today. 2011;16(23–24):1061–1068.
- Tsuchiya K, Nakanishi N, Uetsuji Y, et al. Development of blood extraction system for health monitoring system. Biomed Microdevices. 2005;7(4):347–353.
- Li T, Barnett A, Rogers KL, et al. A blood sampling microsystem for pharmacokinetic applications: design, fabrication, and initial results. Lab Chip. 2009;9(24):3495–3503.
- Khumpuang S, Kawaguchi G, Sugiyama S. Quadruplets-microneedle array for blood extraction. Proceedings of the Nanotechnology Conference and Trade Show, Boston, MA. Citeseer; 2004. p. 7–11.
- Khumpuang S, Horade M, Fujioka K, et al. Geometrical strengthening and tip-sharpening of a microneedle array fabricated by X-ray lithography. Microsyst Technol. 2007;13(3–4):209–214.
- Moon SJ, Lee SS. A novel fabrication method of a microneedle array using inclined deep x-ray exposure. J Micromech Microeng. 2005;15(5):903.
- Li CG, Joung H-A, Noh H, et al. One-touch-activated blood multidiagnostic system using a minimally invasive hollow microneedle integrated with a paper-based sensor. Lab Chip. 2015;15(16):3286–3292.
- Li CG, Dangol M, Lee CY, et al. A self-powered one-touch blood extraction system: a novel polymer-capped hollow microneedle integrated with a pre-vacuum actuator. Lab Chip. 2015;15(2):382–390.
- Mukerjee EV, Collins SD, Isseroff RR, et al. Microneedle array for transdermal biological fluid extraction and in situ analysis. Sens Actuators A Phys. 2004;114(2–3):267–275.
- Vinayakumar KB, Hegde GM, Nayak MM, et al. Fabrication and characterization of gold coated hollow silicon microneedle array for drug delivery. Microelectron Eng. 2014;128:12–18.
- O’Mahony C. Structural characterization and in-vivo reliability evaluation of silicon microneedles. Biomed Microdevices. 2014;16(3):333–343.
- Waghule T, Singhvi G, Dubey SK, et al. Microneedles: a smart approach and increasing potential for transdermal drug delivery system. Biomed Pharmacother. 2019;109:1249–1258. DOI:10.1016/j.biopha.2018.10.078.
- Puttaswamy SV, Lubarsky GV, Kelsey C, et al. Nanophotonic-carbohydrate lab-on-a-microneedle for rapid detection of human cystatin C in finger-prick blood. ACS Nano. 2020;14(9):11939–11949.
- Griss P, Stemme G. Novel, side opened out-of-plane microneedles for microfluidic transdermal interfacing. Technical Digest MEMS 2002 IEEE International Conference Fifteenth IEEE International Conference on Micro Electro Mechanical Systems (Cat No 02CH37266), Las Vegas, Nevada. IEEE; 2002. p. 467–470.
- Smart WH, Subramanian K. The use of silicon microfabrication technology in painless blood glucose monitoring. Diabetes Technol Ther. 2000;2(4):549–559.
- Nayak AK, Ahmad SA, Beg S, et al. Applications of nanocomposite materials in drug delivery [Internet]. Drug Deliv Elsevier; 2018 [cited 2022 May 11]. p. 255–282. Available from https://linkinghub.elsevier.com/retrieve/pii/B9780128137413000121
- Lin L, Pisano AP. Silicon-processed microneedles. J Microelectromech Syst. 1999;8(1):78–84.
- Davis SP, Landis BJ, Adams ZH, et al. Insertion of microneedles into skin: measurement and prediction of insertion force and needle fracture force. J Biomech. 2004;37(8):1155–1163.
- Leone M, van Oorschot BH, Nejadnik MR, et al. Universal applicator for digitally-controlled pressing force and impact velocity insertion of microneedles into skin. Pharmaceutics. 2018;10(4):211.
- Li ADR, Putra KB, Chen L, et al. Mosquito proboscis-inspired needle insertion to reduce tissue deformation and organ displacement. Sci Rep. 2020;10:1–14.
- Gardeniers HJGE, Luttge R, Berenschot EJW, et al. Silicon micromachined hollow microneedles for transdermal liquid transport. J Microelectromech Syst. 2003;12(6):855–862.
- Griss P, Stemme G. Side-opened out-of-plane microneedles for microfluidic transdermal liquid transfer. J Microelectromech Syst. 2003;12(3):296–301.
- Ramasubramanian MK, Barham OM, Swaminathan V. Mechanics of a mosquito bite with applications to microneedle design. Bioinspiration & Biomimetics. 2008;3(4):46001
- Kong XQ, Wu CW. Mosquito proboscis: an elegant biomicroelectromechanical system. Phys Rev E. 2010;82(1):11910.
- Izumi H, Suzuki M, Aoyagi S, et al. Realistic imitation of mosquito’s proboscis: electrochemically etched sharp and jagged needles and their cooperative inserting motion. Sens Actuators A Phys. 2011;165(1):115–123.
- Suzuki M, Sawa T, Takahashi T, et al. Fabrication of microneedle mimicking mosquito proboscis using nanoscale 3D laser lithography system. Int J Autom Tech. 2015;9(6):655–661.
- Il YS, Roe J. Capillary blood sampling for self-monitoring of blood glucose. Diabetes Technol Ther. 1999;1(1):29–37.
- Fonseca V, Hinson J, Pappas A, et al. An erbium: YAG laser to obtain capillary blood samples without a needle for point-of-care laboratory testing. Arch Pathol Lab Med. 1997;121(7):685.
- Cunningham DD. Transdermal microfluidic continuous monitoring systems. Vivo Glucose Sensing. 2009;174:191–215.
- Yoo WS, Min J, Chung P-S, et al. Biochemical and pain comparisons between the laser lancing device and needle lancets for capillary blood sampling: a randomized control trial. Lasers Surg Med. 2021;53(3):316–323.
- Kim J, Yoon S, Lim CS, et al. Comparison between a laser lancing device and lancet for capillary blood sampling, capillary blood hemoglobin measurement, and blood typing. Transfusion (Paris). 2021;61(10):2918–2924.
- Mitragotri S. Current status and future prospects of needle-free liquid jet injectors. Nat Rev Drug Discov. 2006;5(7):543–548.
- Taberner A, Hogan NC, Hunter IW. Needle-free jet injection using real-time controlled linear Lorentz-force actuators. Med Eng Phys. 2012;34(9):1228–1235.
- Xu J, McKeage JW, Ruddy BP, et al. Jet-induced blood release from human fingertips: a single-blind, randomized, crossover trial. J Diabetes Sci Technol [Internet]. 2021 [cited 2022 May 2];19322968211053896. Available from: http://www.ncbi.nlm.nih.gov/pubmed/34711060. .
- Xu J, Ruddy BP, Nielsen PMF, et al. Development of jet-injection nozzles for blood release. 2018 IEEE-EMBS Conference on Biomedical Engineering and Sciences (IECBES), Kuching, Malaysia. IEEE; 2018. p. 583–587.
- Xu J, McKeage JW, Ruddy BP, et al. Blood collection from the porcine ear using a jet injector. 2020 IEEE International Engineering in Medicine & Biology Society (EMBC), Montreal, Canada. IEEE; 2020. p. 5119–5123.
- Grassi G, Scuntero P, Trepiccioni R, et al. Optimizing insulin injection technique and its effect on blood glucose control. J Clin Transl Endocrinol. 2014;1(4):145–150.
- Segal SS. Regulation of blood flow in the microcirculation. Microcirculation. 2005;12(1):33–45.
- Jungheim K, Koschinsky T. Glucose monitoring at the arm risky delays of hypoglycemia and hyperglycemia detection. Diabetes Care. 2002;25(6):956–960.
- Kuriger RJ, Romanowicz BF. Analysis of blood extraction from a lancet puncture. Tech Proc 2004 NSTI Nanotechnol Conf Trade Show. 2004: 275–279
- Kim J, Park S, Nam G, et al. Bioinspired microneedle insertion for deep and precise skin penetration with low force: why the application of mechanophysical stimuli should be considered. J Mech Behav Biomed Mater Internet]. 2018 [cited 2022 Jul 5];78:480–490. Doi: 10.1016/j.jmbbm.2017.12.006.
- Cunningham DD, Henning TP, Shain EB, et al. Blood extraction from lancet wounds using vacuum combined with skin stretching. J Appl Physiol. 2002;92(3):1089–1096.
- Fineberg SE, Bergenstal RM, Bernstein RM, et al. Use of an automated device for alternative site blood glucose monitoring. Diabetes Care. 2001;24(7):1217–1220.
- Craighead DH, McCartney NB, Tumlinson JH, et al. Mechanisms and time course of menthol-induced cutaneous vasodilation. Microvasc Res. 2017;110:43–47.
- Ichioka S, Yokogawa H, Nakagami G, et al. In vivo analysis of skin microcirculation and the role of nitric oxide during vibration. Ostomy Wound Manage. 2011;57(9):40.
- Tzen Y-T, Weinheimer-Haus EM, Corbiere TF, et al. Increased skin blood flow during low intensity vibration in human participants: analysis of control mechanisms using short-time Fourier transform. PLoS One. 2018;13(7):e0200247.
- Maloney-Hinds C, Petrofsky JS, Zimmerman G, et al. The role of nitric oxide in skin blood flow increases due to vibration in healthy adults and adults with type 2 diabetes. Diabetes Technol Ther. 2009;11(1):39–43.
- Johnson PK, Feland JB, Johnson AW, et al. Effect of whole body vibration on skin blood flow and nitric oxide production. J Diabetes Sci Technol. 2014;8(4):889–894.
- Janes M, Pinelli J, Landry S, et al. Comparison of capillary blood sampling using an automated incision device with and without warming the heel. J Perinatol 2002 [cited 2022 May 2];22(2):154–158
- Valeri CR, MacGregor H, Cassidy G, et al. Effects of temperature on bleeding time and clotting time in normal male and female volunteers. Crit Care Med. 1995;23(4):698–704.
- Ellison JM, Stegmann JM, Colner SL, et al., Rapid changes in postprandial blood glucose produce concentration differences at finger, forearm, and thigh sampling sites. Diabetes Care. 2002;25(6):961–964. .
- McGarraugh G, Price D, Schwartz S, et al. Physiological influences on off-finger glucose testing. Diabetes Technol Ther. 2001;3(3):367–376.
- Zavorsky GS, Cao J, Mayo NE, et al., Arterial versus capillary blood gases: a meta-analysis. Respir Physiol Neurobiol. 2007;155(3):268–279. .
- Pavithran AA, Ramamoorthy L, Suryanarayana BS, et al. Comparison of fingertip vs palm site sampling on pain perception, and variation in capillary blood glucose level among patients with diabetes mellitus. J Caring Sci. 2020;9(4):182.
- Kempe KC, Budd D, Stern M, et al. Palm glucose readings compared with fingertip readings under steady and dynamic glycemic conditions, using the onetouch® ultra® blood glucose monitoring system. Diabetes Technol Ther. 2005;7(6):916–926.
- Xue P, Zhang L, Xu Z, et al. Blood sampling using microneedles as a minimally invasive platform for biomedical diagnostics. Appl Mater Today. 2018;13:144–157.
- Sarabi MR, Ahmadpour A, Yetisen AK, et al. Finger-actuated microneedle array for sampling body fluids. Appl Sci. 2021;11(12):5329.
- Hossan MR, Dutta D, Islam N, et al. Review: electric field driven pumping in microfluidic device. Electrophoresis. 2018;39(5–6):702–731.
- Denniff P, Spooner N. Volumetric absorptive microsampling: a dried sample collection technique for quantitative bioanalysis. Anal Chem. 2014;86(16):8489–8495.
- Veenhof H, Koster RA, Junier LAT, et al. Volumetric absorptive microsampling and dried blood spot microsampling vs conventional venous sampling for tacrolimus trough concentration monitoring. Clin Chem Lab Med. 2020;58:1687–1695.
- McDonald TJ, Besser RE, Perry M, et al. Screening for neonatal diabetes at day 5 of life using dried blood spot glucose measurement. Diabetologia. 2017;60(11):2168–2173.
- Harahap Y, Diptasaadya R, Purwanto DJ. Volumetric absorptive microsampling as a sampling alternative in clinical trials and therapeutic drug monitoring during the COVID-19 pandemic: a review. Drug Des Devel Ther. 2020;14:5757.
- Verougstraete N, Lapauw B, van Aken S, et al. Volumetric absorptive microsampling at home as an alternative tool for the monitoring of HbA1c in diabetes patients. Clin Chem Lab Med. 2017;55(3):462–469.