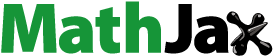
Abstract
Millions of people in the world perform welding as their primary occupation resulting in exposure to metal-containing nanoparticles in the fumes generated. Even though health effects including airway diseases are well-known, there is currently a lack of studies investigating how different welding set-ups and conditions affect the toxicity of generated nanoparticles of the welding fume. The aim of this study was to investigate the toxicity of nine types of welding fume particles generated via active gas shielded metal arc welding (GMAW) of chromium-containing stainless steel under different conditions and, furthermore, to correlate the toxicity to the particle characteristics. Toxicological endpoints investigated were generation of reactive oxygen species (ROS), cytotoxicity, genotoxicity and activation of ToxTracker reporter cell lines. The results clearly underline that the choice of filler material has a large influence on the toxic potential. Fume particles generated by welding with the tested flux-cored wire (FCW) were found to be more cytotoxic compared to particles generated by welding with solid wire or metal-cored wire (MCW). FCW fume particles were also the most potent in causing ROS and DNA damage and they furthermore activated reporters related to DNA double- strand breaks and p53 signaling. Interestingly, the FCW fume particles were the most soluble in PBS, releasing more chromium in the hexavalent form and manganese compared to the other fumes. These results emphasize the importance of solubility of different metal constituents of the fume particles, rather than the total metal content, for their acute toxic potential.
Introduction
It is estimated that 11 million workers worldwide have welding as their primary occupation, and a total of 110 million people are somehow exposed to welding fumes at their work settings (IARC Citation2017). Some of these welders are exposed to stainless steel welding fumes. Gas shielded metal arc welding (GMAW) is one of the most common universal industrial practices (Praveen, Yarlagadda, and Kang Citation2005), in which an electric arc is formed between a consumable wire fed through the welding gun and the base metal. This arc creates sufficient heat to melt and join the metallic surfaces together. A shielding gas is added to provide suitable conditions for the arc and the metal transfer as well as to protect the weld from contaminants and to eliminate slag formation. Welders choose welding conditions, such as potential, current, arc mode, melting rate, filler material feed rate, and choice of shielding gas, based on, among others, the base material and its thickness. The vaporized material generated in the welding process oxidizes and results in a fume mainly containing agglomerates of nanometer-sized primary particles of metals and metal oxides (Mei et al. Citation2018). The composition of the fume is largely dependent on whether a stainless steel or mild steel base alloy is welded. Stainless steel is an iron (Fe)-based alloy that contains at least 11 wt.% chromium (Cr) as well as, depending on grade, other alloy elements such as nickel (Ni), manganese (Mn), and molybdenum (Mo) to achieve particular characteristics and making it considerably more resistant to corrosion compared to mild steel.
Epidemiological studies indicate that welders have an increased risk of developing lung and airway diseases such as bronchitis, airway irritation, and metal fume fever, as reviewed by Antonini (Citation2003). Welding fume was recently reclassified from Group 2B (‘possibly carcinogenic to humans’) carcinogens to Group 1 (‘carcinogenic to humans’) by the IARC (Citation2017). More than 20 case–control studies and nearly 30 occupational or population-based cohort studies have reported increased risks of lung cancer in welders or other workers exposed to welding fumes. There is no distinction made by IARC between welding stainless steel and mild steel or for the use of different types of filler materials or other welding conditions (IARC Citation2017). On the other hand, in vitro studies have demonstrated welding fumes from stainless steel to be significantly more toxic and reactive compared to fumes from mild steel (Antonini et al. Citation1999; Antonini Citation2003; Antonini et al. Citation2005; Leonard et al. Citation2010). This was suggested to be largely due to the presence of more toxic forms of metals such as Cr(VI) and Ni.
Fumes from stainless steel welding have been shown to induce toxicity in vivo, where an increase in markers of oxidative stress and promotion of lung tumorigenesis has been observed (Zeidler-Erdely et al. Citation2013; Falcone et al. Citation2017). Studies have also shown such fumes to induce cytotoxicity, reactive oxygen species (ROS), DNA damage, and inflammatory responses in vitro in mammalian cell lines (McNeilly et al. Citation2004; Antonini et al. Citation2005; Leonard et al. Citation2010; Badding et al. Citation2014; Shoeb et al. Citation2017). The solubility of metal constituents from the fume particles has been proposed to play a major role in the toxicity associated with welding fume exposure, where studies have indicated the toxic responses to welding fumes from stainless steel to be related to the soluble component of the fume, being predominately Cr (McNeilly et al. Citation2004; Antonini et al. Citation2005; Shoeb et al. Citation2017).
Cr has been shown to exist as both Cr(III) and Cr(VI) in welding fume particles from stainless steel (Mei et al. Citation2018). Cr(VI) is classified as a human carcinogen by IARC (Citation2012) and has been shown to be more permeable through plasma membranes compared to other chemical forms of chromium. Previous studies have shown that exposures to compounds containing Cr(VI) result in several toxic effects such as ROS formation, pulmonary injury, and carcinogenesis, as reviewed in Leonard, Harris, and Shi (Citation2004). In addition to Cr(VI), Mn in welding fumes is of concern due to its ability to induce neurotoxic effects (Taube Citation2013).
The welding technique, conditions, and electrode material can affect the composition, reactivity, and size of the fume particles, which may further have an impact on the toxicity. There is no welding fume that is representative for all stainless steel grades, but its composition, such as the Cr(VI) concentration, and generation rate have been shown to vary widely when altering common welding processes and parameters (Zimmer and Biswas Citation2001; Yoon, Paik, and Kim Citation2003; Keane et al. Citation2009; Antonini et al. Citation2011). Mei et al. (Citation2018) further demonstrated an evident effect of the electrode type and conditions related to the base alloy (type of filler material and welding conditions) for the release of metals from the fume particles, in particular Cr(VI) in PBS. It is, however, unclear whether the release of Cr(VI) in PBS correlates with the observed toxicity in lung cells. To our knowledge, no studies have investigated how a range of welding conditions affect the solubility of generated fume particles as well as their toxic potential.
The aim of this study was, therefore, to investigate the toxicity of nine types of welding fume nanoparticles (metal active gas (MAG) welded stainless steel) following exposure of human lung cells and, furthermore, to correlate the observed toxicity with thorough particle characteristics and reactivity. The hypothesis was that the release of metals in PBS, in particular Cr(VI), is a more relevant measure of the toxic response than the total metal content or the content of Cr(VI) of the fume particles. The human bronchial epithelial cell line HBEC3-kt was used as a model since they display a normal non-cancerous phenotype (Ramirez et al. Citation2004). To complement the traditional toxicity assays used to analyze generation of ROS, cytotoxicity and genotoxicity, two different kinds of fumes were further tested in the ToxTracker reporter assay (Hendriks et al. Citation2012; Hendriks et al. Citation2016). These reporter cells are based on mouse embryonic stem (mES) and can monitor the activation of various cellular responses of relevance for carcinogenicity, including DNA damage (Bscl2 and Rtkn reporters), oxidative stress (Srxn1 and Blvrb reporters), general p53-dependent cellular stress (Btg2 reporter), and protein unfolding response (Ddit3 reporter). We recently showed the applicability of these reporters for various nanoparticles including those containing nickel and cobalt (Akerlund et al. Citation2018; Cappellini et al. Citation2018).
Materials and method
Welding fume collection
In total, 15 welding operations were performed by experienced welding operators for nine different conditions including welding of an austenitic (AISI 304 L) or a duplex (UNS S32101) stainless steel grade as base materials with corresponding filler materials of different kind (a certain flux-cored wire (FCW), metal-cored wire (MCW), or solid wire), see Supplementary Table 1 for composition of the filler material alloys. The composition of the flux material that usually consists of several metal oxides and fluoride salts, was not available. Some welding operations were also performed using lower welding current levels (resulting in lower melting rates) to evaluate the influence of melting rate. The arc voltage was adjusted for each combination. Welding speed, stick-out, and torch angles were in accordance with normal welding practice. All welds were performed as bead-on plates (on 5–6 mm thick plates). The fume particles were collected from the different welding operations with varying base metal, filler material, arc mode (spray arc of high current or short arc of low current, resulting in high or low melting rates, respectively), and shielding gas, . The fume particles were collected on Macherey Nagel cellulose filters (Ø 240 mm) using standard particulate fume emission procedures (fume box according to ISO EN 15011-1). Collected fume (nano)particles were in three cases (S1, M1-M2) brushed from the filters directly after welding and delivered for analysis in sealed glass vessels. The other fume particles (F1-F6) were supplied on the filters in sealed plastic bags. Another six collected fumes on filters, generated with similar welding conditions as F1-F6 were investigated on their extent of metal release in order to investigate the reproducibility of the welding fume generation and collection. Data on fume emission rates were made available for the F1-F6 fume particles.
Table 1. Material combinations and test conditions for the generation of welding fume (nano)particles.
Particle morphology
Transmission electron microscopy (TEM) imaging was performed on fume particles from three different conditions (S1, F1, and F3). The fume particles were dispersed in water and were then applied on 200 mesh Cu TEM grids supported with PELCO® TEM grid support film (01814-F from Ted Pella). Imaging was performed using a Hitachi HT 7700 (Hitachi, Tokyo, Japan) electron microscope at 80 kV. Digital images were recorded by means of a Veleta camera (Olympus, Münster, Germany).
Metal release testing
Prior to exposure, filters with collected fume particles (F1-F6 and their six replicates) were cut into a size of 4 cm2 (2 cm × 2 cm). Brushed off fume particles (S1, M1-M2) were weighed using a microbalance to 5 ± 0.5 mg. All fume particles, as well as blank samples (without any welding fume, but with a fume-free filter in the case of the filter samples), were completely immersed in PBS (pH 7.3 ± 0.1) in closed acid-cleaned polyethylene vessels for 24 h. All vessels were placed in a Stuart platform-rocker incubator at 37 ± 0.5 °C at dark conditions and agitated bi-linearly at a 12° inclination and 22 cycles/min. PBS was composed of 8.77 g/L NaCl, 1.28 g/L Na2HPO4, 1.36 g/L KH2PO4, 370 µL/L 50% NaOH, and ultrapure water (18.2 MΩ cm, Millipore, Sweden). A 20 nm inorganic syringe membrane filter was used to separate the solution from undissolved fume particles after exposure. After solid-liquid separation, the solution samples were frozen (in order to maintain chromium speciation) prior to analysis by means of spectrophotometry, or acidified to pH < 2 by 65% ultrapure nitric acid prior to analysis using atomic absorption spectroscopy (AAS).
In order to estimate the total amount of metals of the welding fume particles, a second set of samples was sonicated in 20% aqua regia in an ultrasonic bath for 2 h at room temperature followed by immersion for at least 24 h at room temperature in the same solution. Aqua regia was prepared by equally mixing 25 vol% ultrapure HCl and 65 vol% ultrapure HNO3. A control measurement of a 304 stainless steel powder (<5 µm) showed an acceptable complete dissolution by this method (≥85%). All vessels and instruments in contact with the solutions were acid-cleaned using 10 vol% HNO3 for at least 24 h, and then rinsed four times using ultrapure water.
Released metal concentrations in solution
Total concentrations of released Cr, Ni, Fe, and Mn in PBS were analyzed by means of AAS (AAnalyst 800, Perkin Elmer, Waltham, MA) in the flame mode. The calibration was based on at least four standards, and quality control samples of known concentrations were analyzed regularly throughout the analysis. The limits of detection were 45, 39, 54, and 18 µg/L for Cr, Ni, Fe, and Mn, respectively, as based on three times the highest standard deviation of the blank samples. In the case of the filter samples, the concentrations of Ni and Mn in the blank samples were relatively high and similar to the welding fume samples due to contamination from the filter material. From the metal solution concentration (µg/L) and the exposure volume (L), the amount (µg) of metal released into PBS was calculated and normalized to either the fume particle mass, or to time (per second of welding based on fume emission rates). The released amount of metal (Me) in solution (µg/mg) and the release rate (R µg/s) were calculated as follows:
where
is the measured concentration with the corresponding blank concentration subtracted,
is the exposure volume,
is the mass of the fume particles (the weighed mass of the particles, or the estimated mass based on the filter area and the total fume mass on the filter in the case of filter samples), and
is the fume emission rate measured during the collection of fume particles on filters.
Determination of Cr(VI) in solution
The concentration of Cr(VI) in non-acidified solution samples was measured by mixing 960 µL of the sample with 20 µL of diphenylcarbazide (DPC) solution and 20 µL phosphoric acid (70 vol.%). The reaction between Cr(VI) and DPC results in a pink color, which is spectrophotometrically analyzed at 540 nm by means of ultraviolet-visible spectroscopy (UV–vis. Jenway, 6300 spectrophotometer). The chemical composition of the DPC solution was 1.0 g 1,5-diphenlcarbazide in 100 mL acetone acidified with one drop of glacial ultrapure acetic acid. All calibration samples were based on PBS blank samples and known concentrations of Cr(VI). This procedure is based on the ISO 17075 standard test (ISO Citation2017). The concentrations of calibration standards were 0, 125, 247.5, 495, 990, and 1498 µg/L Cr(VI). The calibration curve was linear (R2 = 0.9986–0.9999). The limit of detection, as based on three times the highest standard deviation of the blank samples, was 41 µg/L.
Elemental analysis of the fume particles and of the outermost particle surface
The fume powders were fixed on carbon tape to perform elemental analysis by means scanning electron microscopy (FEI XL30 ESEM with an X-Max SDD 20 mm2) combined with energy-dispersive x-ray spectroscopy (EDS, instrument) at an accelerating voltage of 10 or 20 kV.
The overall average composition of the outermost (5–10 nm) surface of collected fume particles, fixed in adhesive copper tape, was studied by means of X-ray photoelectron spectroscopy, XPS (UltraDLD spectrometer, Kratos Analytical Manchester, UK) using a monochromatic Al Kα X-ray source (150 W). The pass energy used for detailed spectra was 20 eV, generated for Cr 2p, F 1 s, Fe 2p, Mn 2p, Ti 2p (only for fume particles F1-F6),
Bi 4f, Si 2p, Na 1 s (only for the fume particles S1, M1-M2), K 2p (only for powders S1, M1-M2), N 1 s, O 1 s and C 1 s binding energy at 285.0 eV (used for reference).
Cell culture and reagents
Human bronchial epithelial cells (HBEC3-kt) were cultured in flasks pre-coated with 0.01% collagen (Type I, PureCol®, Advanced BioMatrix) at serum-free conditions in 50% LHC-9 (Laboratory of Human Carcinogenesis-9) medium and 50% Roswell Park Memorial Institute (RPMI) medium. The medium was supplemented with 1% penicillin-streptomycin (PEST, Gibco) and 1% L-glutamine. Cells were seeded 24 h prior to exposure on pre-coated plates.
ToxTracker mES cells were cultured as previously described (Hendriks et al. Citation2012). The cells were maintained in the presence of irradiated mouse embryonic fibroblasts as feeder cells in knockout DMEM with 10% FBS, 2 mM GlutaMAX, 1 mM sodium pyruvate, 100 µM β-mercaptoethanol, and leukemia inhibitory factor (LIF). Cells were seeded (4 × 104 cells/well) on gelatin-coated 96 well plates in complete mES cell medium in absence of feeder cells at 24 h prior to exposure.
Preparation of fume (nano)particle suspensions for cell exposure
The filters with collected fume particles were cut into 4 cm2 (2 cm × 2 cm) pieces, weighed and immersed in 0.5 mL sterilized water in a glass tube. The suspensions were then briefly vortexed, sonicated for 10 min, vortexed and sonicated for another 10 min. The filter piece was carefully removed from the suspension, dried in a fume hood for 2 h, and then weighed again. The concentrations of nanoparticles in the water suspensions from the filter samples were based on the difference in filter weight before and after particle removal. Blank filter extractions showed no measurable weight loss or cytotoxic effects. The welding fume particles received without filters were weighed and suspended in sterilized water (2 mg/mL) in a glass tube followed by sonication in water bath in the same manner as the filters with collected particles. The particle suspensions were further diluted to desired concentrations directly in cell media immediately before exposure of the cells. The fume particles were tested regarding cytotoxicity up to maximum 100 µg/mL. Lower concentrations were used for ROS and DNA damage in order to avoid cytotoxicity and assay interactions. Fresh suspensions were prepared for every experiment.
Cell viability (Alamar blue assay)
HBEC cells were seeded at 1 × 104 cells/well in transparent 96 well plates following exposure to fume particles in HBEC cell medium for 24 and 48 h. Alamar blue assay was performed as previously described (Di Bucchianico et al. Citation2017). Briefly, following exposure the media was removed from the wells and AlamarBlue reagent (10%) in fresh media was added and further incubated for 2 h. The fluorescence was recorded using plate reader Tecan Infinite F200 plate reader (Tecan Trading 125 AG, Männedorf, Switzerland)at 485/590 nm excitation/emission. The average fluorescent intensity for the triplicates in the negative control was set to 100% viability and the mean fluorescence values of the exposed wells were normalized to this value and calculated as % viability, the results were then expressed as % cytotoxicity calculated as 100 minus % viability.
Genotoxicity (comet assay)
HBEC cells were seeded in 24 well plates (5 × 104 cells/well) prior to exposure of the fume particles in HBEC cell medium for 3 h. The alkaline version of the comet assay was performed as previously described (Gliga et al. Citation2014). Following electrophoresis, slides were neutralized, dried, and stained with 1:10 000 dilution of Sybr Green in TAE buffer for 15 min and further scored using a fluorescence microscope (Leica DMLB, Germany) with Comet assay IV software (Perceptive Instruments, Suffolk, UK). At least 50 cells were scored per sample in duplicates.
Acellular ROS generation (DCFH-DA assay)
Acellular generated ROS by the various fume particles were measured using the 2′,7′-dichlorodihydrofluorescein diacetate (DCFH-DA) assay. Briefly, 40 µL DCFH-DA (5 mM in DMSO) was added to 1.6 mL 0.01 M NaOH at dark conditions for cleavage of the diacetate group. After 30 min, 8 mL of PBS was added for neutralization. Horse radish peroxidase (HRP, final concentration 2.2 U/mL) was added to the DCF-solution. An aliquot of 25 µL of the particle suspensions were mixed with 75 µL of DCF-HRP solution (final particle concentrations of 10 and 50 µg/mL) in a black 96 well plate. The fluorescence was recorded (excitation 485 nm, emission 535 nm) at 37 °C after 20 min incubation using a plate reader (Tecan Infinite F200 plate reader, Tecan Trading 125 AG). ROS increase was calculated as fold increase in fluorescence compared to the negative control (PBS added to the DCF-HRP solution).
Reporter cell activation (ToxTracker assay)
Six reporter cell lines (ToxTracker) were used to monitor the activation of various cellular stress responses of relevance for carcinogenicity, including DNA damage, oxidative stress, and protein damage. An initial screening of the cytotoxicity of the fume particles was performed in non-modified mES cells at increasing particle concentrations (0–100 µg/mL) at 24 h. Based on this screening, the fume particles were further tested using green fluorescent protein (GFP) reporter analysis at five increasing concentrations in two-fold dilution steps at 24 h exposure, where the highest dose corresponds to 50–75% cytotoxicity. In cases of no or low observed cytotoxicity, doses up to 100 µg/mL were investigated. Following exposure, cells were washed and trypsinized. Induction of GFP was determined using a Guava easyCyte 8HT flow cytometer (Millipore, Burlington, MA)in intact single cells as described previously (Hendriks et al. Citation2012). Mean GFP fluorescence was used to calculate GFP reporter induction compared to vehicle control exposure. Cytotoxicity was assessed by cell count after 24 h exposure using flow cytometry and expressed as percent of intact cells compared to vehicle control exposure. The GFP response was considered positive if exceeding a two-fold increase, which is based on previous validations (Hendriks et al. Citation2012; Hendriks et al. Citation2016).
Statistical analysis
One-way analysis of variance followed by Bonferroni’s multiple comparison test was used to test for significance between exposures and control (p < 0.05). Results are expressed as mean values ± standard error of the mean (SEM) of at least three independent experiments (n = 3). EC50 values were estimated by dose-response modeling assuming Hills slope (slope = 1). Statistical analysis, linear regression and dose-response modeling were performed using GraphPad Prism 5.02 statistical software (GraphPad Inc., La Jolla, CA). Statistical analysis for differently released metals into PBS was performed by a student's t-test of unpaired data with unequal variance (KaleidaGraph version 4.0, Synergy software, Reading, PA).
Results
The welding fume consists mainly of nanoparticles
In order to investigate the particle size and morphology, we performed TEM imaging. shows TEM images of the fume particles S1, F1, and F3. The images predominantly show nano-sized primary particles typically sized 20–30 nm in aggregates/agglomerates. Our previous published (Mei et al. Citation2018) and unpublished SEM and TEM images show similar particle sizes for welding particles generated at various conditions.
Figure 1. TEM images of welding fume (nano)particles produced using a high melting rate and different base/filler materials and manual welding conditions; S1 (solid filler metal, 304L base alloy, 308L filler alloy); F1 (flux-cored wire, 304L base alloy, 308L filler alloy) and F3 (flux-cored wire, UNS S32101 base and filler alloy).
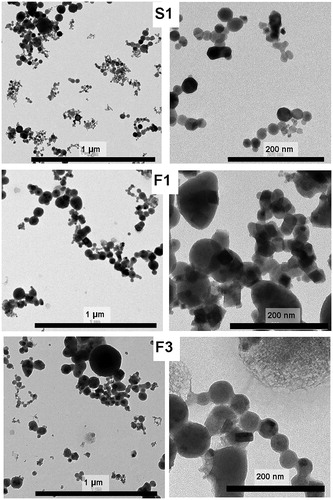
Differences in composition of welding fume particles depend predominantly on the wire filler material
The four metallic elements of interest in this study; Fe, Cr, Mn, and Ni, being the main alloying elements of stainless steel, did only represent a fraction of the fume content, predominantly consisting of elements of the filler material, . Their fraction (Fe + Cr + Mn + Ni) ranged from 46 wt.% in the solid wire fume (S1) to only 12 wt.% of the fume mass for F5 (FCW). In general, S1 and M1-M2 (MCW) had higher contents of these metals (39–46 wt.%) compared to the FCW fume particles tested in this study, F1-F6 (12–21 wt%). S1 differed significantly from F1-F6 in terms of alloy metal content (lower for F1-F6) whereas the content of fluorine (F), sodium (Na), potassium (K), and titanium (Ti) were all higher for F1-F6 fume particles, Supplementary Table 2. XPS was conducted on all fume particles to analyze the chemical composition of their outermost (5–10 nm) surface oxide, . Since many of the welding particles are below the size of 10 nm, XPS results reflect also to a large extent the bulk composition. All observed metals were present in their oxidized form. The F1-F6 particles (generated via FCW electrode welding), are clearly distinguished in the sense that they contain less Fe, Cr, and Mn but more O, F and Bi compared to the other particles. Both Cr(III) and Cr(VI) were observed for all particles in the outermost surface, except for the F6 fume particles, though Cr was detected after extraction in aqua regia, which implies their presence within the bulk of the particles (). The relative amount of total Cr at the particle surfaces, determined by XPS, was higher for the fumes generated with solid or MCW electrodes (9.5–12.8 wt.%) compared with FCW electrodes (0–7.0 wt.%). The fume powders generated with FCW electrodes seem to contain less Fe (0–1.6 wt.%) but more O (66–71 wt.%) when using low melting rate (short arc) compared to fumes (F1-F4) generated with high melting rate (spray arc) (Fe 3.62–8.4 wt.%, O 46–65 wt.%). The relative metal content of Cr, Ni, Mn, and Fe in the outermost 5–10 nm surface of the fume particles was higher if generated via solid and MCW s (S1 and M1-M2 particles; 30–45 wt.%) compared to FCW welding fumes (F1-F6 particles; 0.4–10 wt.%). These findings are in agreement with the results on the alloy metal content determined after complete particle digestion in aqua regia ().
Figure 2. Chemical composition of the outermost surface (5–10nm) of the different welding fume particles determined using X-ray photoelectron spectroscopy (XPS). Ti only tested for F1-F6. Na, K only investigated for S1, M1-M2 with values <1 for all samples and were not included in the graph.
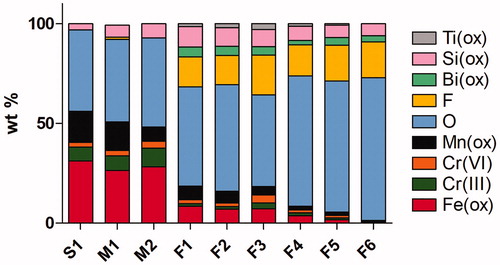
Table 2. Average metal (Cr, Ni, Mn, and Fe) content in the different welding fumes, chemically determined using AAS after complete digestion in aqua regia. Results are expressed as mean of 3 replicates ± SD.
Welding fume particles generated with certain flux-cored wire electrodes release more Cr(VI) and Mn compared with the other fume particles
To determine the amount of released metals from the fume particles, we performed release studies for 24 h at 37 °C in PBS, . Despite the lower alloy metal content of the FCW fume particles (F1-F6) tested in this study, these particles released significantly higher amounts of Cr(VI) (9.4–30 µg/mg) and Mn (4.6–17.5 µg/mg) as compared to fume particles from solid and MCW welding (S1, M1-M2; Cr(VI) 1.8–4.3 µg/mg, Mn 0.13–0.96 µg/mg), when normalized to the total welding fume mass. Welding with FCW electrodes generated (nano)particles that released more Cr(VI) but less Mn per total welding fume mass when generated with spray arc (high melting rates) compared to short arc (low melting rates). shows the released amount of each metal compared with its corresponding total amount in the particles (solubility of particular elements). The solubility of the fume particles into PBS was clearly dominated by Cr(VI) for all welding fumes, followed by Mn for fumes generated with FCW electrodes, . As evident from , the FCW fume particles released a considerably higher percentage of Cr(VI) (55.3–82.5 wt.%) of the total available Cr content compared to fumes from using solid or MCW electrodes (3.2–6.7 wt.%). The same trend was observed when analyzing additional solid wire welding fumes (data not shown). No release of Ni was possible to determine for the F1-F6 fume particles due to high background contamination of Ni in the collection filters. The reproducibility among different welding conditions was relatively high and observed differences among the filler materials consistent for different welding occasions, see Supplementary Table 3. The difference between altered shielding gases among FCWs (F1 compared with F2; F3 compared with F4; F5 compared with F6) was not statistically significant (p =0.3–0.8), Supplementary Tables 3 and 4. The difference between high (F1-F4) and low (F5-F6) melting rates when normalized on total metal content, and Supplementary Table 2, was only statistically significant for a higher Mn solubility at low melting rates as compared to high melting rates (p < 0.006), but not for Cr(VI) solubility (p= 0.7).
Figure 3. Released amounts of Fe, Cr [as Cr(VI), Cr(III)], Mn, and Ni (µg/mg) into PBS (24 h, 37 °C, pH 7.3) from welding fume particles.
![Figure 3. Released amounts of Fe, Cr [as Cr(VI), Cr(III)], Mn, and Ni (µg/mg) into PBS (24 h, 37 °C, pH 7.3) from welding fume particles.](/cms/asset/fcb936f8-15e5-4bd2-85cf-fa202b13fee9/inan_a_1650972_f0003_c.jpg)
Table 3. Average fraction (wt.%) of Cr(VI), Cr(III), Ni, Mn, and Fe released in PBS after 24 h at 37 °C compared to the total metal content of the fume particles.
Welding fume particles generated with flux-cored wires are cytotoxic to lung cells and cause production of ROS
To test the cytotoxic effects of the fume particles, the Alamar blue assay was performed following exposure at particle doses of 5–100 µg/mL for 24 and 48 h, . All particles tested induced cytotoxicity in a dose-dependent manner, although a great variability in cytotoxic potential was observed. The S1 and M1-M2 particles (from solid- and MCW electrodes, respectively) were considerably less cytotoxic compared to F1-F6 (FCWs) at both time points. The FCW fumes generated at high melting rates (F1-F4) were the most toxic with EC50 values at 24 h around 35–50 µg/mL. The F5-F6 particles, generated at low melting rates (normally not used in practice), were less potent with EC50 values of 92 and 100 µg/mL, respectively. No EC50 was determined for the S1 (solid wire electrode) or M1-M2 (MCW electrodes) particles due to low observed cytotoxicity.
Figure 4. Cytotoxicity in HBEC following 24 or 48 h exposure to welding fume particles assessed by Alamar blue assay. 1% Triton X was used as positive control and resulted in a significant cytotoxicity (p < 0.001). At least three independent experiments were performed for each set of particles and dose. The results are presented as mean ± SEM of three or four independent experiments (n = 3–4). Asterisks indicate significant (p < 0.05) increase compared to control (set to 0% cytotoxicity, not shown in graph).
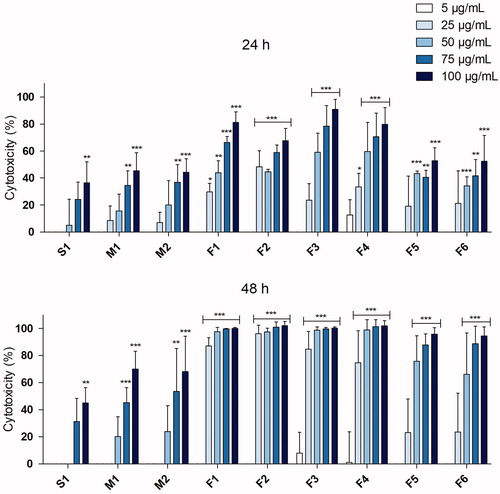
The ability of the fume particles to generate ROS production (in the absence of cells) was tested. In line with the cytotoxicity, the fume particles generated with the tested FCWs (F1-F6) were more reactive compared to the other particles and welding settings (S1, M1-M2), . Thus, a significant ROS production was observed for the F1-F6 particles whereas the S1, M1, and M2 particles did not generate any ROS. However, in contrast to the cell viability results, the F5 and F6 particles generated at low melting rates were slightly more potent than the other settings inducing a 4-fold ROS increase (p <0.001) at 10 µg/mL.
Figure 5. Acellular ROS assessed by DCFH-DA. ROS produced by welding fume particles after 20 min incubation at 37 °C in cell-free conditions in PBS. At least three independent experiments were performed for each set of particles and dose. The results are presented as mean ± SEM of three independent experiments (n= 3). Asterisks indicate significant (p < 0.05) increase compared to DCF.
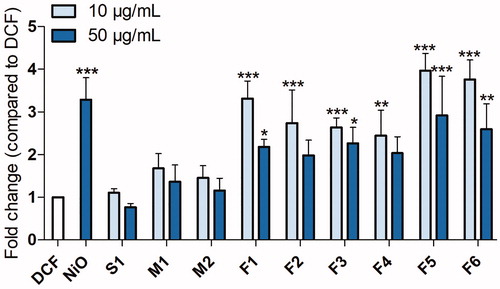
Cytotoxicity of welding fume particles correlates with Cr(VI) release in PBS
To further explore the relation between the release of Cr(VI) and cytotoxicity, linear regression was performed using the levels of cytotoxicity found at the particle dose of 50 µg/mL. As observed in , a significant correlation (R2 =0.86) was observed. No correlation was observed between cytotoxicity and release of Mn (R2 =0.2) (see Supplementary Figure 1). Mn release was, however, well correlated to the acellular ROS production (R2 =0.92) (see ). This correlation was not observed for Cr(VI) release (R2 =0.12) (Supplementary Figure 1).
Figure 6. Correlation between metal release and toxicity. The upper figure shows linear regression between the Cr(VI) release in PBS per fume mass and cytotoxicity observed after exposure to 50 µg/mL of welding particles for 24 h. The lower figure shows the correlation between Mn release in PBS per fume mass and induction of acellular ROS assessed by DCFH-DA.
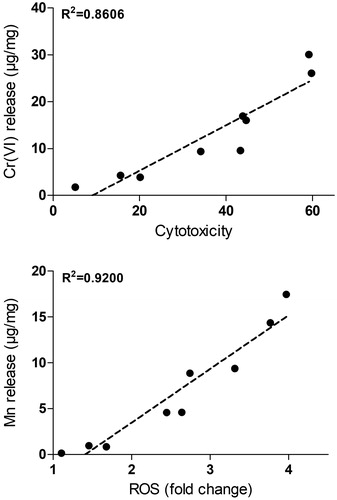
Welding fume particles generated with the tested flux-cored wires induce DNA damage in lung cells
Following the cytotoxicity and ROS assessments, three fume particles (S1, F1, and F3) were selected for assessment of their ability to induce DNA damage in HBEC cells by using the comet assay following 3 h exposure, . The particles generated with solid wire (S1) showed no DNA damage at any of the investigated concentrations. In contrast, a statistically significant induction of DNA damage was observed in response to the F1 and F3 particles (certain FCWs) starting at 25 and 10 µg/mL, respectively, with an evident dose-response. The F3 powder (UNS S32101 as base and filler alloy) was found to be the most potent, reaching up to almost a 7-fold change at the highest dose level (50 µg/mL) compared to the levels of the controls. The F1 particles (304L as base alloy and 308 L as filler material) resulted in a maximum induction of a 4-fold increase compared to the control.
Figure 7. DNA strand breaks in cultivated lung cells following 3 h exposure to welding fume particles (S1, F1, and F3). DNA strand breaks were assessed using the comet assay and shown as % of DNA in tail. NiO nanoparticles (25 µg/mL) were used as positive control and resulted in significant increased levels (31 ± 3.9% DNA in tail). The bars show mean ± SEM of three independent experiments for each set of particles and dose (n= 3).

Fume (nano)particles generated via tested flux-cored wire welding induce signaling pathways related to cancer
To gain mechanistic insight into the toxicity of welding fume particles, the ToxTracker reporter assay was used for two of the collected fume particles (S1 and F3), see . Before the reporter cell experiments, a cytotoxicity dose range finding study was performed for the welding particles at concentrations up to 100 µg/mL for 24 h in order to find the relevant doses to be investigated. The results showed, in accordance with the cytotoxicity results in HBEC, large variance between the two fumes, demonstrating the tested FCW welding fume (F3) to be considerably more toxic compared to welding using solid wire (S1) (as also demonstrated in ). The less cytotoxic particles (S1) were further tested in the ToxTracker assay at concentrations up to 100 µg/mL, while the more toxic particles (F3) were only tested up to 12.5 µg/mL.
Figure 8. Toxtracker assay response to welding fume particles generated via solid wire welding of 304L with a 308L filler alloy (S1, left) or a certain flux-cored wire welding of UNS S32101 (F3, right). By using flow cytometry, viability was assessed as the fraction of intact cells. Toxtracker reporter activation in live cells was measured as GFP-fluorescence. The reporters Srxn1-GFP and Blvrb-GFP report on oxidative stress (Nrf2-associated and Nrf2-independent, respectively), Bscl2-GFP and Rtkn-GFP report on DNA damage (ATR/Chk1 signaling and NF-kB signaling, respectively), Ddit3-GFP indicate unfolded protein response and Btg2-GFP p53-associated cellular stress. The data shows the mean of three independent experiments ± SEM (n= 3).
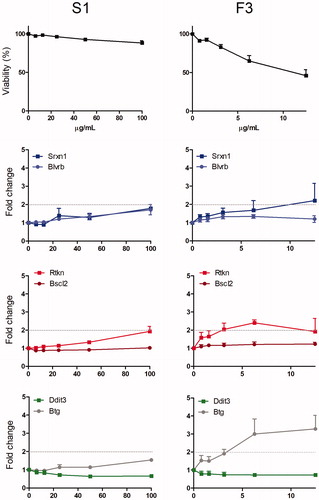
The results from the reporter cell activation are clearly in line with the data from the lung cells suggesting genotoxicity caused by the welding fume particles generated using FCWs tested in this study (F3) but not when using solid wires (S1). The reporter cells are considered activated when the fluorescence is at least doubled when compared to non-exposed cells. For the F3 particles, a clear induction was observed for one of the oxidative stress reporters (Srxn1 reporter) where, interestingly, the Rtkn reporter related to DNA double-strand breaks was activated already at an exposure dose of 3.1 µg/mL. No effect was observed for the DNA damage reporter related to the formation of bulky DNA adducts and subsequent inhibition of DNA replication (Bscl2 reporter). The F3 particles also caused an induction of pathways related to p53-signaling (Btg2 reporter), indicating general stress, whereas the reporter for protein unfolding (Ddit3) was not activated.
Fume generation rates and soluble Cr(VI) show the necessity of good ventilation and personal protection
The toxic properties of the welding fumes are clearly important for understanding health risks, but the generation rate of the fumes (leading to exposure) is also highly relevant. For welding using the FCWs tested in this study, the fume generation rates were estimated as mass per second (). From these values and observed data for the amount of soluble Cr(VI) from the fume powders in PBS after 24 h (), the generation of soluble Cr(VI) per second was calculated. The results show the highest generation rate, expressed as soluble Cr(VI) per second, for the F3 and F4 particles (139–169 µg/s), i.e. fumes generated at high melting rate of duplex stainless steel, UNS S32101, using certain FCW electrodes, compared with the F1-F2 particles generated using similar settings during welding of stainless steel 304 L (67–69 µg/s) and low melting rate welding fumes from UNS S32101 (F5-F6, 18–19 µg/s), see . The total air concentration of different metals in the welding fume during a given time of welding was compared to the existing EU occupational exposure limits (OELs, summarized in Supplementary Table 5). Considering that Cr(VI) has the lowest OEL among the metals considered in this study, and the most soluble metal in PBS, observed data was used to calculate the air volume that is required to ensure that the short-term OEL for Cr(VI) of 15 µg/m³ is not exceeded, . The calculations should mainly be used as an indication of relative comparison among the different welding fume samples and show the necessity of very good ventilation and personal protection.
Table 4. Fume emission rates, calculated exposure rate of PBS-released Cr(VI) from welding fume particles and the dilution air volume required to not exceed the short-term occupational exposure limit (OEL; 15 µg/m³). The calculation assumes that the welding fume is equally distributed in the air space of the welder. The exposure rate of soluble Cr(VI) was calculated based on measured fume emission rates according to (CEN 2009), per second and minute of welding, and the amount of Cr(VI) release in PBS (37 °C, 24 h). The required air volume for dilution (m³) is calculated assuming 5 min welding during a 15 min period.
Discussion
In this study, we explored the toxicity of a variety of welding fume particles and further aimed to elucidate the correlation to metal content, speciation, and fume particle dissolution. Despite the fact that all fumes were generated by MAG welding of stainless steel, we found a great variability in their metal content, extent of metal release as well as toxic potency depending on specific welding conditions. This further suggests that the welding conditions such as the filler material, the shielding gas, and melting rate likely can be optimized to generate welding fumes with less hazardous properties.
The most evident result in the study was the influence of different types of filler materials for the capability of the fume particles to release metals and cause toxicity. By using a range of assays we clearly demonstrated that fume particles generated with FCWs tested in this study (F1-F6) are considerably more toxic regarding all endpoints investigated. They contained less Cr, Mn, Fe, and Ni, as compared to fume particles generated using solid or MCWs, but substantially more Cr(VI) and Mn were released from these tested FCW particles (F1-F6) in PBS. Thus, there was no or only a weak relation between the particle metal content and the release of the corresponding metal in PBS.
When considering the impact of the base alloy and filler alloy composition (which is selected based on the base alloy) comparing welding fumes generated with FCWs only, our results showed that the UNS S32101 alloy (F3-F4) generated fume particles released more Cr(VI) in PBS, compared to fume particles from 304 L welding under similar conditions (F1-F2). These findings are in accordance with results reported by Mei et al. (Citation2018). In line with this, the F3 particles were also more genotoxic compared to F1 (). Furthermore, fume particles generated at high melting rates (F3-F4) released substantially more Cr(VI) per fume mass compared to particles generated at low melting rates (F5-F6), whereas the opposite situation was observed for released Mn. These observed differences regarding the melting rates and base/filler alloys are likely caused by necessary differences in welding conditions such as potential, current, feed rate of the filler material, shielding gas composition, temperature, and melting rate, which all need to be adjusted, among others, based on the base and filler alloy composition and the thickness of the material. Another important difference is the slightly higher Cr content in the base and filler alloys of UNS S32101 as compared with 304L/308L.
An important aspect is the possible correlation between metal release and toxicity. Our results suggest a correlation between the extent of Cr(VI) release in PBS and the cytotoxic response. The pH and absence of complexing and reducing agents of PBS enable the detection of Cr(VI) without causing any significant transformation to Cr(III). Cr(VI) can in contrast to Cr(III) readily be taken up by cells. Following inhalation (nano)particles containing Cr(VI) can be retained in the lung tissue for many years, particularly at major bifurcations. Released Cr(VI) is typically reduced by ascorbate present in the lung fluids, but since the release takes place at the lung surface, some Cr(VI) can also be transported into the epithelial cells escaping extracellular reduction (Urbano, Ferreira, and Alpoim Citation2012; Proctor et al. Citation2014). In the cells, Cr(VI) is in several steps reduced to the more stable Cr(III) that can interact directly with DNA or indirectly via the formation of ROS (Salnikow and Zhitkovich Citation2008; IARC Citation2012). Likely, the release of Cr(VI) in PBS, as shown in our study, represents Cr(VI) release at various conditions of relevance for cell toxicity, i.e. both at the cell surface and the intracellular release of Cr species. In addition to Cr(VI), the release of Mn can be important for the toxicity observed. Our study indicates a correlation between the release of Mn and its potency of generating ROS (R2 =0.92). Ni release was not measurable for most fume particles, partly explained by Ni contamination from the filters on which the welding fumes were sampled (see experimental). However, relatively low levels of Ni are expected to be released from the fume particles based on results from our previous study (Mei et al. Citation2018).
In agreement with our findings, Antonini et al. (Citation1999) demonstrated that the solubility of welding fume particles influences their effects on viability of macrophages as well as production of ROS. The most toxic welding fumes from manual metal arc welding of stainless steel was also found to be the most soluble, with a soluble fraction containing mostly Cr. Further, the soluble fraction was found to induce cytotoxicity alone as compared to the insoluble fraction. The same effect was not observed for ROS production, and, in accordance with our results, it was suggested that soluble Cr seemed to play a lesser role in ROS production. In addition, the soluble fractions of welding fume particles have been shown to play a central role in mediating pro-inflammatory responses in A549 cells (McNeilly et al. Citation2004).
To complement the more conventional toxicity assays, the ToxTracker reporter assay was utilized for the welding particles S1 and F3 (solid wire 304L/308L welding fume and FCW UNS S32101) to provide additional mechanistic insight into the genotoxicity of welding fume particles. The S1 particles were nontoxic to the reporter cells and did not induce any of the six reporters. These findings are well in accordance with the results from the other toxic endpoints investigated in this study, showing no clear effects in toxic response. In contrast, the F3 particles induced the oxidative stress reporter Srxn1, the DNA damage reporter Rtkn, and the p53-associated reporter Btg2. The Srxn1 gene is regulated by the Nrf2-antioxidant pathway, a key regulator of antioxidant enzymes in response to oxidative stress (Hendriks et al. Citation2012; Hendriks et al. Citation2016). Shoeb et al. (Citation2017) found a significant increase in the expression of Nrf2 protein in RAW264.7 macrophages in response to manual metal arc welding fumes (containing Cr) from stainless steel but not for fumes from gas metal arc welding of mild steel (no Cr content), effects also observed in vivo. The Rtkn reporter is associated with induction of DNA strand breaks via NFkB signaling. The induction of this reporter is thus in accordance with the results from the comet assay, showing the potency of the F3 particles but not the S1 particles to induce DNA strand breaks. The Btg2 gene is controlled by p53 and is transcriptionally induced upon exposure to cellular stressors such as DNA damage and oxidative stress-inducing agents (Hendriks et al. Citation2016). When comparing the ToxTracker results for the welding fume particles (F3) with our previous findings on nanoparticles containing nickel (Ni metal, NiO) (Akerlund et al. Citation2018) and cobalt (Co metal, CoO, and Co3O4) (Cappellini et al. Citation2018) some conclusions can be drawn. The welding particles were less potent in causing oxidative stress, similarly potent as the Co and CoO nanoparticles to induce the DNA damage reporter Rtkn1, and more potent to induce the p53 dependent stress reporter. Overall, the data from the ToxTracker suggests a large difference in the genotoxic potential of the welding fume particles generated via solid wire electrodes (S1) compared with the FCW (F3) tested in this study. This difference, in combination with comet assay data on DNA damage in lung cells, suggests that welding fume particles generated with the tested FCW may have a higher carcinogenic potential.
The carcinogenic potential of particles depends, however, on many factors; one of them being the lung retention. Particles with slower dissolution kinetics are more likely to have a longer retention in the lung and may gradually release toxic metal species. On the other hand, a slower dissolution may enable protection by e.g. antioxidant defense in the lung lining and intracellularly. Still, it is important to note that this was a short term study and it would be valuable to further reveal cellular changes using a long term in vitro assay, as we previously did with silver nanoparticles (Gliga et al. Citation2018). It would also be of interest to further study metal release in more relevant in vitro systems e.g. by using mucus or even in the presence of cells such as macrophages (Carlander et al. Citation2019). Clearly, in vivo studies would also be valuable; a recent long-term study (up to 84 d) on mice suggests, for example, that iron oxides play a larger role for inflammation and tumor promotion than more reactive metal compounds (Cr(VI) and Ni(II) oxides) (Falcone et al. Citation2018).
In conclusion, manual welding of stainless steel using different methods and electrode materials results in the generation of fume particles of largely varying toxic potential. Our study clearly elucidates the importance of the type of filler material where the use of the tested FCWs resulted in the most toxic particles. This study further emphasizes the importance of metal release, and not the total metal content, for the acute toxic potential of welding fume particles. The approach of assessing metal release in PBS, with particular focus on Cr(VI) and Mn, seems to be a plausible way forward to determine the toxic potential of welding fume particles and opens up for rapid screening of novel combinations of welding processes, shielding gases, and filler materials.
TNAN-2019-OR-0171-File010.docx
Download MS Word (273.2 KB)Acknowledgment
Ola Runnerstam, Swerim AB, is highly acknowledged for assistance in project organization and coordination and Lars Haag at Karolinska Institutet for TEM imaging.
Disclosure statement
GH, RD, and NM are employed by Toxys, a Dutch company that offers ToxTracker as a commercial service.
Additional information
Funding
References
- Akerlund, E., F. Cappellini, S. Di Bucchianico, S. Islam, S. Skoglund, R. Derr, I. Odnevall Wallinder, G. Hendriks, and H. L. Karlsson. 2018. “Genotoxic and Mutagenic Properties of Ni and NiO Nanoparticles Investigated by Comet Assay, Gamma-H2AX Staining, Hprt Mutation Assay and ToxTracker Reporter Cell Lines.” Environmental and Molecular Mutagenesis 59(3): 211–222. doi:10.1002/em.22163.
- Antonini, J. M. 2003. “Health Effects of Welding.” Critical Reviews in Toxicology 33(1): 61–103. doi:10.1080/713611032.
- Antonini, J. M., M. Keane, B. T. Chen, S. Stone, J. R. Roberts, D. Schwegler-Berry, R. N. Andrews, D. G. Frazer, and K. Sriram. 2011. “Alterations in Welding Process Voltage Affect the Generation of Ultrafine Particles, Fume Composition, and Pulmonary Toxicity.” Nanotoxicology 5(4): 700–710. doi:10.3109/17435390.2010.550695.
- Antonini, J. M., S. S. Leonard, J. R. Roberts, C. Solano-Lopez, S. H. Young, X. Shi, and M. D. Taylor. 2005. “Effect of Stainless Steel Manual Metal Arc Welding Fume on Free Radical Production, DNA Damage, and Apoptosis Induction.” Molecular and Cellular Biochemistry 279(1–2): 17–23. doi:10.3109/17435390.2010.550695.
- Antonini, J., N. Lawryk, G. G. Murthy, and J. D. Brain. 1999. “Effect of Welding Fume Solubility on Lung Macrophage Viability and Function In Vitro.” Journal of Toxicology and Environmental Health Part A 58(6): 343–363. doi:10.1080/009841099157205.
- Badding, M. A., N. R. Fix, J. M. Antonini, and S. S. Leonard. 2014. “A Comparison of Cytotoxicity and Oxidative Stress from Welding Fumes Generated with a New Nickel-, Copper-Based Consumable versus Mild and Stainless Steel-Based Welding in RAW 264.7 Mouse Macrophages.” PLoS One 9(6): e101310. doi:10.1371/journal.pone.0101310.
- Cappellini, F., Y. Hedberg, S. McCarrick, J. Hedberg, R. Derr, G. Hendriks, I. Odnevall Wallinder, and H. L. Karlsson. 2018. “Mechanistic Insight into Reactivity and (Geno)Toxicity of Well-Characterized Nanoparticles of Cobalt Metal and Oxides.” Nanotoxicology 12(6): 602–620. doi:10.1080/17435390.2018.1470694.
- Carlander, U., K. Midander, Y. S. Hedberg, G. Johanson, M. Bottai, and H. L. Karlsson. 2019. “Macrophage-Assisted Dissolution of Gold Nanoparticles.” ACS Applied Bio Materials 2(3): 1006–1016. doi:10.1021/acsabm.8b00537.
- Di Bucchianico, S., F. Cappellini, F. Le Bihanic, Y. Zhang, K. Dreij, and H. L. Karlsson. 2017. “Genotoxicity of TiO2 Nanoparticles Assessed by Mini-Gel Comet Assay and Micronucleus Scoring with Flow Cytometry.” Mutagenesis 32(1): 127–137. doi:10.1093/mutage/gew030.
- Falcone, L. M., A. R. Erdely, M. Salmen, L. Keane, V. Battelli, L. Kodali, A. B. Bowers, et al. 2018. “Pulmonary Toxicity and Lung Tumorigenic Potential of Surrogate Metal Oxides in Gas Metal Arc Welding-Stainless Steel Fume: Iron as a Primary Mediator versus Chromium and Nickel.” PLoS One 13(12): e0209413. doi:10.1371/journal.pone.0209413.
- Falcone, L., A. T. Erdely, L. A. Meighan, R. Battelli, W. Salmen, S. McKinney, A. Stone, et al. 2017. “Inhalation of Gas Metal Arc–Stainless Steel Welding Fume Promotes Lung Tumorigenesis in a/J Mice.” Archives of Toxicology 91(8): 2953–2962. doi:10.1007/s00204-016-1909-2.
- Gliga, A. R., S. Di Bucchianico, J. Lindvall, B. Fadeel, and H. L. Karlsson. 2018. “RNA-Sequencing Reveals Long-Term Effects of Silver Nanoparticles on Human Lung Cells.” Scientific Reports 8(1): 6668. doi:10.1038/s41598-018-25085-5.
- Gliga, A. R., S. Skoglund, I. Odnevall Wallinder, B. Fadeel, and H. L. Karlsson. 2014. “Size-Dependent Cytotoxicity of Silver Nanoparticles in Human Lung Cells: The Role of Cellular Uptake, Agglomeration and Ag Release.” Particle and Fibre Toxicology 11(1): 11. doi:10.1186/1743-8977-11-11.
- Hendriks, G., M. Atallah, B. Morolli, F. Calléja, N. Ras-Verloop, I. Huijskens, M. Raamsman, B. van de Water, and H. Vrieling. 2012. “The ToxTracker Assay: Novel GFP Reporter Systems That Provide Mechanistic Insight into the Genotoxic Properties of Chemicals.” Toxicological Sciences 125(1): 285–298. doi:10.1093/toxsci/kfr281.
- Hendriks, G., R. S. Derr, B. Misovic, B. Morolli, F. M. Calleja, and H. Vrieling. 2016. “The Extended ToxTracker Assay Discriminates between Induction of DNA Damage, Oxidative Stress, and Protein Misfolding.” Toxicological Sciences 150(1): 190–203. doi:10.1093/toxsci/kfv323.
- IARC. 2012. Chromium (VI) Compounds (100C). Lyon, France: IARC.
- IARC. 2017. Welding, Molybdenum Trioxide and Indium Tin Oxide (118). Lyon, France: IARC.
- ISO. 2017. ISO 17075-1, Leather – Chemical Determination of Chromium(VI) Content in Leather – Part 1: Colorimetric Method. Geneva, Switzerland: ISO.
- Keane, M., S. Stone, B. Chen, J. Slaven, D. Schwegler-Berry, and J. Antonini. 2009. “Hexavalent Chromium Content in Stainless Steel Welding Fumes Is Dependent on the Welding Process and Shield Gas Type.” Journal of Environmental Monitoring 11(2): 418–424. doi:10.1039/B814063D.
- Leonard, S. S., B. T. Chen, S. G. Stone, D. Schwegler-Berry, A. J. Kenyon, D. Frazer, and J. M. Antonini. 2010. “Comparison of Stainless and Mild Steel Welding Fumes in Generation of Reactive Oxygen Species.” Particle and Fibre Toxicology 7(1): 32. doi:10.1186/1743-8977-7-32.
- Leonard, S. S., G. K. Harris, and X. Shi. 2004. “Metal-Induced Oxidative Stress and Signal Transduction.” Free Radical Biology and Medicine 37(12): 1921–1942. doi:10.1016/j.freeradbiomed.2004.09.010.
- McNeilly, J. D., M. R. Heal, I. J. Beverland, A. Howe, M. D. Gibson, L. R. Hibbs, W. MacNee, and K. Donaldson. 2004. “Soluble Transition Metals Cause the Pro-Inflammatory Effects of Welding Fumes In Vitro.” Toxicology and Applied Pharmacology 196(1): 95–107. doi:10.1016/j.taap.2003.11.021.
- Mei, N., L. Belleville, Y. Cha, U. Olofsson, I. Odnevall Wallinder, K. A. Persson, and Y. S. Hedberg. 2018. “Size-Separated Particle Fractions of Stainless Steel Welding Fume particles - A Multi-Analytical Characterization Focusing on Surface Oxide Speciation and Release of Hexavalent Chromium.” Journal of Hazardous Materials 342: 527–535. doi:10.1016/j.jhazmat.2017.08.070.
- Praveen, P., P. K. D. V. Yarlagadda, and M. J. Kang. 2005. “Advancements in Pulse Gas Metal Arc Welding.” Journal of Materials Processing Technology 164–165: 1113–1119. doi:10.1016/j.jmatprotec.2005.02.100.
- Proctor, D. M., M. Suh, S. L. Campleman, and C. M. Thompson. 2014. “Assessment of the Mode of Action for Hexavalent Chromium-Induced Lung Cancer following Inhalation Exposures.” Toxicology 325: 160–179. doi:10.1016/j.tox.2014.08.009.
- Ramirez, R. D., S. L. Sheridan, M. Girard, Y. Sato, J. Kim, M. Pollack, Y. Peyton, et al. 2004. “Immortalization of Human Bronchial Epithelial Cells in the Absence of Viral Oncoproteins.” Cancer Research 64(24): 9027–9034. doi:10.1158/0008-5472.CAN-04-3703.
- Salnikow, K., and A. Zhitkovich. 2008. “Genetic and Epigenetic Mechanisms in Metal Carcinogenesis and Cocarcinogenesis: Nickel, Arsenic, and Chromium.” Chemical Research in Toxicology 21(1): 28–44. doi:10.1021/tx700198a.
- Shoeb, M., V. B. Kodali, L. M. Farris, T. Bishop, R. Meighan, T. Salmen, J. R. Eye, et al. 2017. “Evaluation of the Molecular Mechanisms Associated with Cytotoxicity and Inflammation after Pulmonary Exposure to Different Metal-Rich Welding Particles.” Nanotoxicology 11(6): 725–736. doi:10.1080/17435390.2017.1349200.
- Taube, F. 2013. “Manganese in Occupational Arc Welding Fumes–Aspects on Physiochemical Properties, with Focus on Solubility.” The Annals of Occupational Hygiene 57(1): 6–25. doi:10.1093/annhyg/mes053.
- Urbano, A. M., L. M. Ferreira, and M. C. Alpoim. 2012. “Molecular and Cellular Mechanisms of Hexavalent Chromium-Induced Lung Cancer: An Updated Perspective.” Current Drug Metabolism 13(3): 284–305. doi:10.2174/138920012799320464.
- Yoon, C. S., N. W. Paik, and J. H. Kim. 2003. “Fume Generation and Content of Total Chromium and Hexavalent Chromium in Flux-Cored Arc Welding.” The Annals of Occupational Hygiene 47(8): 671–680. doi:10.1093/annhyg/meg063.
- Zeidler-Erdely, P. C., T. G. Meighan, A. Erdely, L. A. Battelli, M. L. Kashon, M. Keane, and J. M. Antonini. 2013. “Lung Tumor Promotion by Chromium-Containing Welding Particulate Matter in a Mouse Model.” Particle and Fibre Toxicology 10(1): 45. doi:10.1186/1743-8977-10-45.
- Zimmer, A. T., and P. Biswas. 2001. “Characterization of the Aerosols Resulting from Arc Welding Processes.” Journal of Aerosol Science 32(8): 993–1008. doi:10.1016/S0021-8502(01)00035-0.