Abstract
Prenatal particle exposure has been shown to increase allergic responses in offspring. Carbon nanotubes (CNTs) possess immunomodulatory properties, but it is unknown whether maternal exposure to CNTs interferes with offspring immune development. Here, C57Bl/6J female mice were intratracheally instilled with 67 of μg multiwalled CNTs on the day prior to mating. After weaning, tolerance and allergy responses were assessed in the offspring. Offspring of CNT-exposed (CNT offspring) and of sham-exposed dams (CTRL offspring) were intranasally exposed to ovalbumin (OVA) once weekly for 5 weeks to induce airway mucosal tolerance. Subsequent OVA sensitization and aerosol inhalation caused low or no OVA-specific IgE production and no inflammation. However, the CNT offspring presented with significantly lower OVA-specific IgG1 levels than CTRL offspring. In other groups of 5-week-old offspring, low-dose sensitization with OVA and subsequent OVA aerosol inhalation led to significantly lower OVA-specific IgG1 production in CNT compared to CTRL offspring. OVA-specific IgE and airway inflammation were non-significantly reduced in CNT offspring. The immunomodulatory effects of pre-gestational exposure to multiwalled CNTs were unexpected, but very consistent. The observations of suppressed antigen-specific IgG1 production may be of importance for infection or vaccination responses and warrant further investigation.
Introduction
Exposure to particulate matter, including automotive, industrial and household emissions, has long been linked to aggravation of asthma as well as to development of allergic diseases and asthma in children and adults (Parnia and Frew Citation2001; Weichenthal, Dufresne, and Infante-Rivard Citation2007). Maternal exposure to environmental factors has furthermore been suggested to influence the developing fetus. The immature immune system appears more susceptible to perturbating exposures than the adult immune system (Van Loveren and Piersma Citation2004; Blossom et al. Citation2017). Epidemiological studies have shown associations between maternal exposure to particulate matter during pregnancy and increased occurrence of wheeze, airway disease and infection in the children, but it is difficult to separate the contributions from pre- and postnatal exposures (Jedrychowski et al. Citation2009, Citation2010a, Citation2010b, Citation2013). Several studies have investigated the effects of maternal particulate exposures on child immune function and suggest that prenatal particulate exposures might modulate the developing immune system and/or lead to increased susceptibility to develop allergy and asthma (Pandya et al. Citation2002; Dietert and Zelikoff Citation2008; Wang and Pinkerton Citation2008; Finkelman Citation2014; Manners et al. Citation2014). A key experimental study is the work by Fedulov and coworkers (Fedulov et al. Citation2008). They exposed pregnant mice intranasally (i.n.) to micro-sized TiO2, diesel exhaust particles or carbon black particles on gestational day 14. Following a protocol of sensitization, the offspring presented with increased airway inflammation and airway hyper-responsiveness (Fedulov et al. Citation2008). Another study observed decreased spleen CD4+ and CD8+ cell numbers at postnatal day 1–5, but few changes in splenic mRNA expression levels of immune-related genes in offspring of mice i.n. instilled with a suspension of nanosized carbon black particles on gestational days 5 and 9 (Shimizu et al. Citation2014). In contrast, carbon black nanoparticle exposure by i.n. instillation on gestational days 9 and 15 increased offspring thymocyte and splenocyte numbers and thymic Traf6 gene expression, a gene related to tolerance induction, at postnatal day 5 (El-Sayed et al. Citation2015).
Recently, fiber-like carbon nanotubes (CNTs) have attracted interest across several industries, due to their many attractive properties such as high tensile strength, conductivity, and unique electronic properties. CNTs are strong immunomodulators causing cytotoxicity, immune activation, and pulmonary inflammation (Nygaard et al. Citation2009; Vlaanderen et al. Citation2017; Dong and Ma Citation2018; Francis and Devasena Citation2018). Hence, potential adverse health effects of CNTs, particularly in the occupational settings, should be seriously considered along with identification of the mechanisms of toxicity (Canu et al. Citation2016). Since CNT-like structures, probably from anthropogenic sources, have been found in the lungs of Parisian children with asthma (CitationKolosnjaj-Tabi et al. 2015), knowledge on such adverse health effects may also be of relevance for the general population.
The worldwide prevalence of allergic diseases has increased for more than 50 years (Pawankar et al. Citation2013). Allergies are caused by an overreaction of the body’s immune system to substances that would otherwise be harmless. Allergic responses are primarily triggered by allergen-immunoglobulin E (IgE) cross-linking on mast cells (Galli, Tsai, and Piliponsky Citation2008). Symptoms occur when the sensitized individual receives successive exposures to the allergen, which stimulates mast cells on mucosal surfaces to degranulate, resulting in allergic inflammation. The normal mucosal immune response to encountered innocuous antigens is induction of immunological tolerance to the antigens (Lowrey et al. Citation1998; Smole et al. Citation2017).
Based on findings of offspring immune activation by particles, as well as immune activating properties of CNTs, we hypothesized that pre-gestational maternal exposure to multiwalled CNT would lead to exacerbated immune responses in offspring. This could be caused by perturbation of the normal tolerance induction by mucosal exposure, where ‘immunological tolerance is defined as a state of specific non-responsiveness to a particular antigen induced by previous exposure to that same antigen’ (Lowrey et al. Citation1998, p. 93). We investigated the hypothesis in a mouse model simulating women exposed at a time point close to conception by exposing female mice prior to mating. The hypothesis was tested in different immunization models: 1) Mucosal tolerance was induced in offspring prior to allergic sensitization – a method which has been assessed in numerous models of airway exposure (Holt et al. Citation1981; Lowrey et al. Citation1998; Clausen et al. Citation2003; Hansen et al. Citation2012; Smole et al. Citation2017); 2) Allergic response was induced by suboptimal immunization. This immunization sought to induce a weak allergic response by a booster injection. By using a suboptimal immunization, an exacerbation of the allergic response in the offspring due to the maternal treatment can be observed (Fedulov et al. Citation2008; Manners et al. Citation2014). Such an exacerbation can be difficult to detect with conventional immunization protocols, as these are designed to evoke a strong response that can hamper detection of differences between exposure groups.
Materials and methods
Animals and housing
Inbred, naïve C57Bl/6J mice, 7–8 weeks of age, were purchased from Taconic M&B (Ry, DK) and five mice of the same sex were randomly assigned to polypropylene cages. The cages were sanitized twice weekly and furnished with bedding material (Tapvei, Estonia), small aspen gnaw sticks (Tapvei, Estonia), plastic nesting houses (Mouse House 80-ACRE011, Techniplast, Italy) and nesting material (Enviro Dri, Lillico Biotechnology, UK). The light-period was from 6 a.m. to 6 p.m. Food (Altromin no. 1314 for dams/litters and 1324 for weaned offspring, Altromin, Lage, DE) and municipal tap water were available ad libitum. Housing and experimental conditions are described in more detail in our previous studies using the dams (Barfod et al. Citation2015; Johansson et al. Citation2017).
Ethical statement
Treatment of the animals followed procedures approved by The Animal Experiment Inspectorate in Denmark (Permissions no. 2010/561-1779 C1 and no. 2006/561-1900-C2). All experiments were performed by trained personnel and conformed to the Danish Regulations on Animal Experiments (LBK nr. 474 af 15/05/2014 and BEK nr. 88 af 30/01/2013), which includes guidelines on care and use of animals in research. The ARRIVE guidelines were followed for the reporting of this study (Kilkenny et al. Citation2010).
Experimental protocols
Upon arrival, all animals were group-housed, with males and females in separate rooms. The female cages were supplemented with soiled bedding from the male cages for synchronization of the estrous cycle (Whitten Citation1959). After one week, all animals were weighed and males moved to single housing. The day before exposure, cages were semi-randomized into exposure groups based on body weights to obtain comparable group means. The next day, females were anesthetized by 4% isoflurane and 50 µL of vehicle or 67 μg of multiwalled CNTs dispersed in 50 µL of vehicle (approx. 2.7 mg/kg) were injected into the tracheal lumen by intratracheal instillation. The following day, one female was transferred to a cage with one male. Females were then weighed twice weekly. The male was removed when the female’s body weight had increased at 2–3 successive weighings, by more than 25%, or if pups could be palpated. See the study by (Johansson et al. Citation2017) for a detailed description of the study design.
The maternal exposure was performed in two independent experiments separated in time, and designated Experiment 1 and Experiment 2. Details of each experiment are provided below and in the Supplementary figures. From each experiment, the used offspring came from 7 CTRL and 7–8 CNT dams. A maximum of one male and one female sibling were used in any one protocol to avoid litter effects. Offspring were weaned at 3 to 4 weeks of age and housed 3–4 mice of the same sex and maternal treatment per cage. Maternal lung inflammation following multiwalled CNT exposure was assessed by differential cell count of bronchoalveolar lavage fluid (BALF, see below) after weaning (7–8 weeks after exposure; Johansson et al. Citation2017).
Protocol for tolerance induction
Tolerance was induced in the offspring (Experiment 1, ) and was followed by a standard intraperitoneal (i.p.) immunization model as described in a previous study (Hansen et al. Citation2012). One male and one female offspring from 7 CTRL and 8 CNT dams, i.e. in total 30 offspring, were used. Offspring were anesthetized by inhalation of 4% isoflurane and exposed once weekly to 5 μg OVA i.n. for 5 weeks, starting at age 4 weeks. The volume of the i.n. vehicle bolus was increased with age to facilitate maximal lung deposition, so that offspring of 4–5 and 6+ weeks of age were given OVA in 25 and 35 µL saline, respectively (Hansen et al. Citation2007). At 9 weeks of age, offspring were sensitized i.p. with 5 µg OVA and 1 mg Al(OH)3 in a bolus of 500 µL saline, followed one week later by a booster injection with 5 µg OVA in the same volume. At 11 weeks of age, the offspring were exposed to an aerosol from a 0.001% OVA solution for 1 h on two consecutive days (). Two days later, animals were killed in Hypnorm/Dormicum anesthesia by collection of heart blood. BALF and organs were collected.
Figure 1. Overview of pre-conceptional exposure of female mice, mating as well as postnatal OVA exposure of offspring. (A) Mucosal tolerance was induced by weekly i.n. instillations of 5 µg OVA in offspring aged 4-8 weeks. This was followed by allergen immunization at age 9 weeks (5 µg OVA in 1 mg Al(OH)3) and a booster of 5 µg OVA one week later. Finally, offspring were exposed to a 0.001% OVA aerosol by inhalation. (B) Suboptimal sensitization (5 µg OVA in 1 mg Al(OH)3, no booster) and inhalation of allergen (1% OVA) by was performed in offspring, starting at 2 weeks of age.
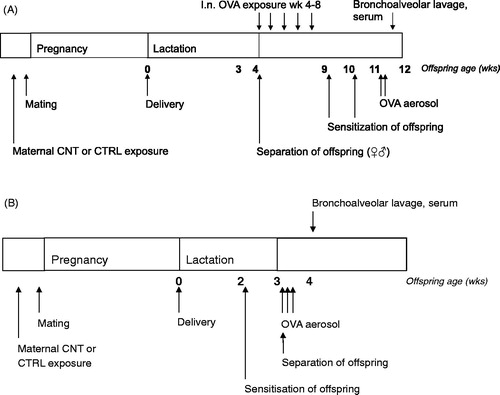
Protocol for allergic sensitization
Allergic sensitization (Experiment 2, ) was tested in a suboptimal sensitization protocol based on Fedulov et al. (Citation2008), as we hypothesized that the CNT offspring would exhibit an exaggerated immune response. The protocol was used to induce airway allergy in one male and one female offspring from each of 7 CTRL and 7 CNT dams, i.e. in a total of 28 offspring. At 2 weeks of age, offspring were immunized i.p. with 5 µg OVA and 1 mg Al(OH)3 in a bolus of 100 µL saline. One week later, the offspring were exposed to an aerosol of a 1% OVA solution for 20 min on three consecutive days () and killed three days later in Hypnorm/Dormicum anesthesia by collection of heart blood. BALF and organs were collected.
Naïve offspring
Serum from 4-week-old naïve offspring from CTRL and CNT dams was collected for measurement of IgM levels. Further, spleens were collected from naïve CTRL and CNT offspring on postnatal day 7. These offspring were siblings to the offspring undergoing tolerance induction and/or sensitization as described above and therefore, from both Experiment 1 and 2.
Preparation of multiwalled CNTs
Female mice were exposed to the multiwalled CNT NM-400 (OECD sponsorship program). The dose level (67 μg/mouse) was chosen as it in previous studies induced a robust inflammatory lung response shortly after exposure without affecting gestational parameters, such as litter size and birth weights (Hougaard et al. Citation2013; Poulsen et al. Citation2015a). NM-400 consists of sub-µm long and highly curved multiwalled CNTs with mean diameter and length of 10 and 295 nm, respectively, containing approximately 16 wt% of incombustible impurities, mainly aluminum (5.3 wt%), iron (0.4 wt%) and cobalt (0.2 wt%; Hougaard et al. Citation2013). The specific surface area was 298 m2/g (Jackson et al. Citation2015). Endotoxin levels in the used batch of NM-400 were below the detection limit of 0.05 EU/mL, as assessed by the kinetic Limulus Amebocyte Lysate test (Kinetic-QCL endotoxin kit, Lonza, Walkersville Inc., USA; Hougaard et al. Citation2013).
Multiwalled CNTs were dispersed in vehicle as described (Johansson et al. Citation2017). Multiwalled CNTs were sonicated for 16 min at 1.34 mg/mL in 0.2 µm filtered, γ-irradiated Nanopure Diamond UV water (Pyrogens: <0.001 EU/mL, total organic carbon: 3.0 ppb) with 2% mouse serum using a 400 W Branson Sonifier S-450D (Branson Ultrasonics Corp., Danbury, CT, USA) mounted with a disruptor horn and operated at 10% amplitude. Samples were cooled on ice during sonication. Mouse serum was prepared in our own laboratory by collection of heart blood from another group of anesthetized mature female mice (C57BL/6BomTac, Taconic Europe, Ejby, Denmark); heart blood was pooled, mixed and aliquoted.
The particle size number distribution in vehicle was determined by dynamic light scattering (DLS; Zetasizer Nano ZS, Malvern Instruments Ltd., UK) using the Dispersion Technology Software as previously described (Johansson et al. Citation2017).
Allergen and anesthetics
Ovalbumin (OVA, grade V) was obtained from Sigma-Aldrich, Germany and the Al(OH)3 adjuvant was from Alhydrogel, Brenntag Biosector, Denmark. Four % isoflurane was used as inhalation anesthesia. Hypnorm (fentanyl citrate 0.315 mg/mL and fluanisone 10 mg/mL from Janssen Pharma, Belgium) and Dormicum (midazolam 5 mg/mL from Roche, Switzerland) were used during the terminal procedures of heart blood, BALF and organ collection, each diluted 1:1 in water and mixed prior to subcutaneous injection of a volume of 125 μL per mouse.
Generation of OVA aerosols
For airway challenge, offspring were placed, but not neck restrained, in tubes extending head-out into an exposure chamber (Clausen et al. Citation2003). The OVA aerosol was generated using a PARI LC STAR nebulizer (PARI GmbH, Germany). Characterization of the aerosol has been described in previous studies (Clausen et al. Citation2003; Hansen et al. Citation2007). In these studies, air concentrations of the aerosolized 1% OVA solution was approx. 50 mg/m3 as measured by filter collection. Based on this, lung deposition was estimated to 20 µg/20 min exposure. Hence, the 1% and 0.001% OVA solutions, used for aerosolization in the present study, are estimated to imply exposure to 50 and 0.05 mg/m3, respectively. The estimated cumulated lung deposition is 30 and 0.02 µg after exposure to a 1% solution for three days and to a 0.001% solution for two days, respectively.
Collection and processing of BALF
BAL was collected by flushing of the lungs with ice-cold sterile saline using a tracheal cannula as previously described (Hougaard et al. Citation2013). Lungs were flushed 3 times with the same volume of ice-cold saline in dams and offspring in the tolerance study. In the allergic sensitization study, lungs were flushed 3 times, each time with a new volume of saline that were subsequently pooled for each mouse. Volumes for flushing were 0.9 mL for dams, 1 mL for male offspring and 0.8 mL for female offspring. BALF was then centrifuged (400 × g for 10 min at 4 °C). The supernatant was frozen and the pellet resuspended in a 100 μl HAM’s F12 (Cat No: 21765029, Gibco, Thermo Fischer Scientific, Waltham, MA, USA). The total number of cells was counted in a Nucleocounter NC-900-004 (ChemoMetec, Allerød, DK). For differential counts, cytospin preparations were made (Cytospin 2, StatSpin, Inc., Norwood, MA, USA; 1000 × g, 4 min). Slides were stained with May–Grünwald/Giemsa, and the same technician inspected blinded slides. Cells were identified by standard morphology and differentiated into neutrophils, eosinophils, lymphocytes, and macrophages. For each slide, 200 cells were counted and the percentage of each cell type was calculated.
Antibody measurements by ELISA
Serum was diluted 1:10 for measuring OVA-specific IgE in an in-house assay as previously described (Hansen et al. Citation2016). For OVA-specific IgG1, serum was diluted 1:5 and antibodies measured by a competitive enzyme-linked immunosorbent assay (ELISA) according to the manufacturer’s recommendations (catalogue number MBS733854, MyBioSource, San Diego, CA, USA). Serum for IgM analyses was diluted 10 000-fold and analyzed according to the manufacturer’s recommendation (catalogue number 88-50470, Invitrogen™, Waltham, MA, USA). Samples were analyzed in duplicate and absorbance was read at 405 nm on an Epoch microplate reader (BioTek, Winooski, VT, USA).
Protein analysis in BALF
Total protein concentration in BALF was determined with the CBQCA Protein Quantitation kit (C-6667, Molecular Probes™, Eugene, US) according to the manufacturer in 50 µL of undiluted BALF sample in 96-well plates. A standard curve was generated with bovine serum albumin. Plates were read in a fluorometer (Victor2, Wallac 1420 multilabel counter, EG&G Wallac, Turku, Finland) at a wavelength of 550 nm with excitation at 465 nm.
TGF-β mRNA expression
Tgf-β mRNA levels were quantified as described (Saber et al. Citation2009, Citation2012). In short, RNA was purified from spleen and lung tissue on Maxwell® 16 (Promega, Madison, WI, USA) using Maxwell® 16 LEV simply RNA Tissue Kit (AS1280, Promega, Madison, WI, USA) and converted into cDNA using Taq‐Man® reverse transcription reagents (Applied Biosystems, Naerum, DK). Tgf-β gene expression was determined using real‐time RT‐PCR and normalized to 18S RNA using the 2−ΔCt method as previously described (Saber et al. Citation2012).
Statistical analyses
A maximum of one male and one female sibling were used in each assay, and individual pups were therefore regarded as independent observations. Data were analyzed by the general linear model (GLM) procedure. Maternal treatment (CTRL/CNT) and offspring sex (male/female) were used as fixed factors. For one analysis of IgM levels, maternal treatment (CTRL/CNT) and experiment (1/2) were fixed factors. When data did not conform to the assumptions of the ANOVA, they were log-transformed. P-values of main and interaction effects are reported in the figures. No significant interaction effects were found and therefore, post hoc tests comparing subgroups were not performed. Mann–Whitney’s U-test was used to test for differences in IgE levels between CTRL and CNT offspring in the tolerance study (). If not otherwise indicated, medians and individual values are shown. Statistical analyses were performed in Minitab vers. 15. The level of significance was p < 0.05.
Figure 2. Effect of pre-conceptional maternal CNT exposure on mucosal tolerance in offspring. After 5 weeks of i.n. OVA exposure, offspring were s.c. immunized with OVA and exposed to OVA by inhalation, c.f. . OVA-specific IgE (A) and IgG1 (B) were measured in serum and the total number of cells was counted in recovered BALF (C). Individual and median values of female (black) and male (white) offspring are shown (n = 7-8). p-values of the GLM analyses are given above B and C. Broken line indicates the lower detection limit of the ELISA.
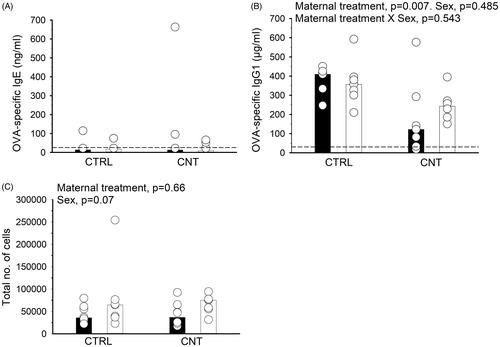
Results
Immune responses following mucosal tolerance induction were affected by pre-gestational maternal CNT exposure
It was hypothesized that maternal CNT exposure would interfere with development of mucosal tolerance. Therefore, offspring were repeatedly exposed to OVA by i.n. instillation to induce mucosal tolerance, and later sensitized to, and airway challenged with OVA to observe the effectiveness of the tolerance induction. OVA-specific IgE levels were generally very low, and no differences between the groups were observed (, Mann–Whitney U-test, p = 0.6). In contrast, OVA-specific IgG1 production was measurable and significantly lowered in CNT offspring independently of sex (). The total number of cells recovered from BALF was unaffected by the maternal treatment () and no allergic airway inflammation was observed in any offspring group (data not shown). A comparable immunization protocol performed in 2-week-old offspring without prior tolerance induction led to increased eosinophilic inflammation (Supplementary Figure 2(D)), supporting the notion that allergic inflammation was not observed in either of the groups, when mucosal tolerance had been induced.
Effect of pre-gestational CNT exposure on airway allergy development in offspring
Mucosal tolerance development in terms of suppression of allergen-specific IgE and prevention of allergic inflammation appeared to be unaffected by the maternal CNT exposure. Therefore, development of airway allergy was investigated next; 2-week-old offspring were sensitized with OVA and airway challenged with a 1% OVA solution one week later.
The IgE production was not statistically significantly affected by the maternal CNT exposure, albeit only one of the 7 CNT males presented with a OVA-specific IgE value above the detection limit (). As in the previous study, OVA-specific IgG1 was significantly lowered by maternal exposure to CNT (). Interestingly, the total number of cells in spleens of the immunized offspring was numerically (but non-significantly) lower in CNT offspring compared to CTRL offspring (). The suppressed antibody production was also reflected by a non-significant, but numerically lower number of inflammatory cells recovered from BALF of the CNT offspring (, mean CTRL = 180 700 and CNT = 113 979). The inflammation was mainly driven by an influx of eosinophils, which also appeared higher in the CTRL compared to CNT offspring (). That pulmonary inflammation appeared lower in CNT offspring was supported by the significantly lower level of protein in BALF compared to CTRL offspring ().
Figure 3. Effect of pre-conceptional maternal CNT exposure on allergen immunization in offspring. At age 2 weeks, offspring were s.c. immunized with OVA and subsequently exposed to OVA by inhalation, c.f. . OVA-specific IgE (A) and IgG1 (B) were measured in serum and the total number of spleen cells counted (C). Individual and median values of female (black) and male (white) offspring are shown (n = 7, except for CTRL offspring, where n = 5 for the IgG1 measurements). p-values of the GLM analyses are given above the figures. Data in (B) were log-transformed for the statistical analysis. Broken line indicates the lower detection limit of the ELISA.
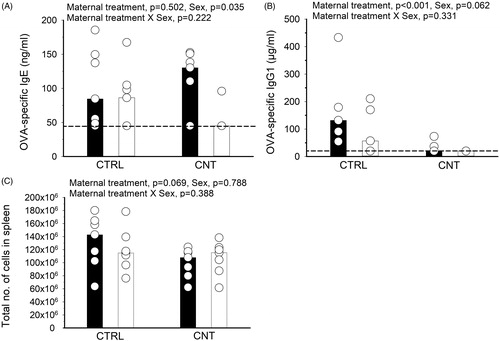
Figure 4. Effect of pre-conceptional maternal CNT exposure on allergen immunization in offspring. At age 2 weeks, offspring were s.c. immunized with OVA and exposed to OVA by inhalation, c.f. . Allergic airway inflammation was assessed in BALF from the total number of cells (A), the percentage (B) and total number (C) of eosinophils and from protein levels (D). Individual and median values of female (black) and male (white) offspring are shown (n = 7). p-values of the GLM analyses are given above the figures. Data in (A) and (C) were log-transformed for the statistical analyses.
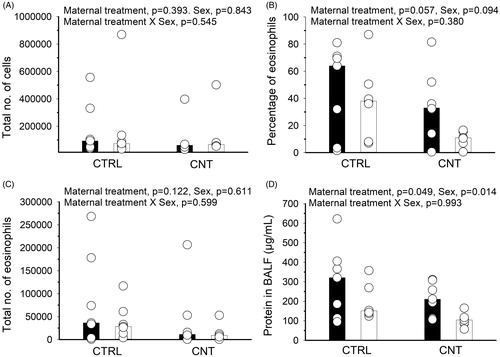
The suppressed allergen-specific IgG1 production was reproduced in two additional experiments, where different immunization protocols were used to induce airway allergy in young offspring (Supplementary Figures 1(B) and 2(B)). Interestingly, in one of the sensitization protocols, where a very low OVA concentration was used for the airway challenges, a significantly increased inflammatory response occurred in lungs of the CNT offspring (Supplementary Figure 1(C)). It was mainly driven by an influx of macrophages and not eosinophils (data not shown and Supplementary Figure 1(D)). This was in contrast to the study reported in and and could be related to the different concentrations of OVA used for exposure in the sensitization protocols.
IgM levels in naïve and immunized offspring
To investigate if the observation of immune suppression was related only to the allergen-exposure or rather occurred due to a constitutive decrease in antibody production, serum IgM levels were measured in naïve (unimmunized) offspring at 4 weeks of age. Serum was collected from offspring in the two separate maternal exposure experiments. The IgM levels were generally lower in CNT compared to CTRL offspring, although not significantly, but differed significantly between experiments (). No differences in IgM levels were observed in 4-week-old immunized offspring (, same offspring as depicted in ).
Figure 5. Effect of pre-conceptional maternal CNT exposure on IgM levels in naïve 4-week-old offspring (A, n = 4-5 per group per experiment) and suboptimally sensitized offspring (B, n = 4-5, from ). In B, individual and median values of female (black) and male (white) offspring are shown. The limit of detection for the assay was 3990 ng/mL. p-values of the GLM analyses are given above the figures. All data were log-transformed for the statistical analyses.
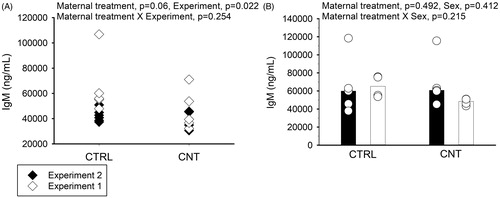
TGF-β expression in offspring
Tgf-β mRNA expression was quantified in spleens from naïve offspring at postnatal day 7 () and in lung tissue from the immunized offspring (, same offspring as in and ). Maternal CNT exposure neither affected Tgf-β mRNA levels at postnatal day 7 nor in the older immunized offspring. The immunized females had significantly higher expression levels compared to males ().
Figure 6. Tgf-β mRNA expression was quantified in (A) spleens from naïve offspring at postnatal day 7 (n = 4), and in (B) lung tissue from the sensitized offspring (n = 7, from ). Individual and median values of female (black) and male (white) offspring are shown. p-values of the GLM analyses are given above the figures.
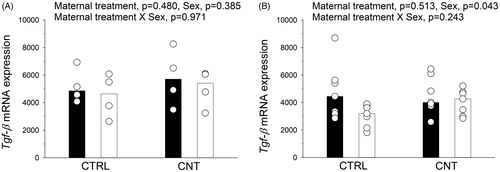
Discussion
The present study investigated the effects of maternal pre-conceptional exposure to multiwalled CNTs on development of mucosal tolerance and allergy in the offspring. Development of mucosal tolerance was unaffected by maternal exposure to CNTs, since IgE levels were similarly low in CTRL and CNT offspring. In contrast, IgG1 levels after tolerance induction were decreased in CNT compared to CTRL offspring, indicating an immunosuppressive effect of pre-conceptional CNT exposure. IgG1 levels were also suppressed in CNT offspring following allergic sensitization in three separate protocols. IgE levels were numerically lower in male CNT offspring in two of three sensitization studies, but this difference did not reach statistical significance.
The suppression of IgG1 antibody production appeared very robust: it was observed following allergen exposure in both sexes, in four different immunization protocols, and in offspring originating from two independent experiments of maternal pre-gestational CNT exposure. These findings were unexpected and did not support our initial hypothesis that pre-gestational exposure to multiwalled CNT would exacerbate offspring immune responses and increase allergic antibody production and inflammation. We speculated whether suppression of antibody production could be due to a general immunosuppression but this was not supported from the non-significant decrease in IgM production in naïve offspring at 4 weeks of age. IgM may be transferred from milk, but antibody uptake ceases after postnatal day 16 in mice (Mackenzie Citation1990) making it likely that the measured IgM originates from the offspring and not from mother’s milk. We have no explanation for the differences in IgM levels between experiments.
In mice and humans, the production of IgE antibodies occurs directly from IgM or through sequential switching requiring an IgG1 intermediate (Xiong et al. Citation2012; He et al. Citation2015, Citation2017; Looney et al. Citation2016). IgG1 is produced when high doses of antigen are used for immunization (Sudowe, Rademaekers, and Kolsch Citation1997; Hansen et al. Citation2007). This was the case for the immunizations in the present study. Therefore, the IgG1-pathway of isotype switching was most likely stimulated. In two of three immunization protocols, IgE levels appeared suppressed in male CNT offspring (in and Supplementary Figure 2(A), but not in Supplementary Figure 1(A)), but the total IgM level was not suppressed in sensitized offspring (). This suggests a specific suppression of the IgG1-isotype switching. TGF-β regulates isotype switching and antibody secretion, but mRNA expression was unaffected by the maternal CNT exposure. Further, the pulmonary allergic inflammation was not suppressed in the three allergic sensitization protocols. Overall, the lack of clear suppression of IgM and IgE production suggests that only certain branches of the offspring’s own antibody production was affected by maternal CNT exposure.
A key study performed in the Kobzik lab (Fedulov et al. Citation2008) indicated that gestational exposure to micro-sized particles (TiO2, diesel exhaust and carbon black) increased asthma susceptibility in offspring sensitized and subsequently exposed to aerosolized OVA as compared to control offspring. This was observed as increased airway inflammation and hyper-responsiveness in offspring of particle-exposed dams, but antibody levels were not reported. Also in the C57Bl/6 strain, in utero exposure to diesel exhaust enhanced allergen-specific IgE and airway inflammation and hyperreactivity in offspring (Manners et al. Citation2014). Other studies of prenatal exposure to diesel exhaust particle/particulate matter, however, report contradictory findings (Corson et al. Citation2010; Rychlik et al. Citation2019). Corson et al. (Citation2010) found suppression of allergen-specific IgE, but normal levels of allergen-specific IgG1 and airway inflammation in Balb/c offspring exposed to diesel exhaust in utero. Rychlik et al. (Citation2019) found evidence of suppressed allergen-induced airway inflammation after ambient ultrafine particle pollution exposure in utero of both Balb/c and C57Bl/6 mice. The latter study did not report antibody production. Overall, the above studies present contradictory findings probably related to the different maternal exposure protocols and different sensitization regimens of the offspring. Immunosuppression is at least partly observed in the two latter studies, thus supporting the present study, where we present strong evidence of immune suppression upon pre-conceptional exposure to CNTs. As Rychlik et al. (Citation2019, p. 3444) states: ‘The source and size of particles as well as timing of both PM [particulate matter] and allergen exposure may be critical due to the complexity of fetal and infant lung and immune system development during this period.’
The prevalence of asthma and allergy have increased for decades, and therefore, many studies have sought explanations in the concurrently increasing ambient air pollution. Few studies have addressed if immune responses to infections and vaccinations are sensitive to prenatal particulate exposure. One study suggests that prenatal exposure to particulate matter increases the susceptibility to respiratory infections (Jedrychowski et al. Citation2013). IgG responses are involved in protection against pathogens and development of immunity following vaccination. The pronounced serological suppression due to maternal CNT exposure could potentially lead to insufficient immune responses to early life infections. Here, only IgG1 was investigated. It is therefore warranted to investigate whether other antibody isotypes or IgG subclasses in the adaptive humoral immune response are also prone to disruption by maternal exposure to particulates.
The dose of multiwalled CNTs used in the current study is equivalent to pulmonary deposition of 200 mg into the human airways. This dose is very high, but is used in the present study as proof-of-principle. Further, the exposure did not affect litter and gestation parameters (litter size, implantations, implantation loss and sex ratio) nor maternal and offspring weight change during lactation, as evaluated for the maternal exposure in Experiment 2 (Johansson et al. Citation2017). The present study is to our knowledge the first study reporting suppression of adaptive immunity in offspring of rodents exposed prenatally or pre-gestationally to non-soluble, carbon-based nanoparticles (See literature search in Supplementary Table 1).
Pulmonary instillation of multiwalled CNTs induces pronounced eosinophilic and neutrophilic inflammation. This was assessed for the dams of Experiment 2 as previously reported (Barfod et al. Citation2015; Johansson et al. Citation2017). Stimulation of overt pulmonary inflammation is also described for other CNTs (Nygaard et al. Citation2009; Poulsen et al. Citation2015b). Eosinophil-dominated defense mechanisms are well-known from helminthic infections and may be elicited through toll-like receptor (TLR) activation (Rajasekaran, Anuradha, and Bethunaickan Citation2017). Therefore, we speculate if the inflammation stimulated by multiwalled CNTs could be caused by activation of TLRs. Recent experimental data supports that CNTs can bind to certain TLRs (Turabekova et al. Citation2014). A recent paper showed that multiwalled CNT-induced DNA damage in human lung epithelial cells was dependent on activation of TLR9 and subsequent generation of NO (Hiraku et al. Citation2016). Singlewalled CNTs were shown to induce chemokine secretion in macrophages through a TLR2/4-MyD88-NFκB signaling pathway, while graphene oxide did not elicit any response on chemokine-encoding genes (Mukherjee et al. Citation2018). The possible activation of TLRs may be implicated, since studies showed that maternal exposure to bacteria or LPS reduced features of allergic asthma in offspring following allergic sensitization (Blumer et al. Citation2005, Citation2007; Conrad et al. Citation2009; Cao et al. Citation2010). However, only one of these studies found a significant reduction of allergen-specific IgE and IgG1 as well as suppressed pulmonary inflammation in the offspring (Blumer et al. Citation2005). Presently, this mechanism remains very speculative.
An alternative mechanism is proposed based on the studies from Mitchell et al. (Citation2007, Citation2009): In adult mice inhaling CNTs, antibody production was suppressed. This was suggested to occur via release of TGF-β from the lungs and subsequent change in splenic T cell function through a COX-2-mediated mechanism (Mitchell et al. Citation2009). TGF-β in milk is taken up in the rodent intestine (Zhang et al. Citation1999), suggesting a possibility for lactational transfer and hence postnatal effects of CNT-induced maternal inflammation.
In conclusion, this is the first study to report immunosuppression of IgG1-antibodies in the allergic response of offspring, whose mothers were exposed to multiwalled CNTs. Future studies are encouraged to investigate immune responses in offspring driven by the IgG isotypes such as delayed-type hypersensitivity reactions, vaccine or infection responses, and whether the effect is maintained beyond adolescence.
Supplemental Material
Download PDF (464.7 KB)Acknowledgements
The technical assistance from Michael Guldbrandsen, Lisbeth M. Petersen, Maria Hammer, Elzbieta Christiansen, Anne-Karin Asp and Lourdes Petersen is gratefully acknowledged.
Disclosure statement
The authors report no conflict of interest.
Data availability statement
The data that support the findings of this study are available from JSH and KSH, upon reasonable request.
Correction Statement
This article has been republished with minor changes. These changes do not impact the academic content of the article.
Additional information
Funding
References
- Barfod, K. K., K. Vrankx, H. C. Mirsepasi-Lauridsen, J. S. Hansen, K. S. Hougaard, S. T. Larsen, A. C. Ouwenhand, et al. 2015. “The Murine Lung Microbiome Changes During Lung Inflammation and Intranasal Vancomycin Treatment.” The Open Microbiology Journal 9 (1): 167–179. doi:10.2174/1874285801509010167.
- Blossom, S. J., S. B. Melnyk, M. Li, W. D. Wessinger, and C. A. Cooney. 2017. “Inflammatory and Oxidative Stress-Related Effects Associated with Neurotoxicity Are Maintained after Exclusively Prenatal Trichloroethylene Exposure.” Neurotoxicology 59: 164–174. doi:10.1016/j.neuro.2016.01.002.
- Blumer, N., U. Herz, M. Wegmann, and H. Renz. 2005. “Prenatal Lipopolysaccharide-Exposure Prevents Allergic Sensitization and Airway Inflammation, but Not Airway Responsiveness in a Murine Model of Experimental Asthma.” Clinical and Experimental Allergy 35 (3): 397–402. doi:10.1111/j.1365-2222.2005.02184.x.
- Blumer, N, et al. 2007. “Perinatal Maternal Application of Lactobacillus rhamnosus GG Suppresses Allergic Airway Inflammation in Mouse Offspring.” Clinical and Experimental Allergy 37: 348–357.
- Canu, I. G, et al. 2016. “Human Exposure to Carbon-Based Fibrous Nanomaterials: A Review.” International Journal of Hygiene and Environmental Health. 219: 166–175.
- Cao, L., J. Wang, Y. Zhu, I. Tseu, and M. Post. 2010. “Maternal Endotoxin Exposure Attenuates Allergic Airway Disease in Infant Rats.” American Journal of Physiology-Lung Cellular and Molecular Physiology 298 (5): L670–L677. doi:10.1152/ajplung.00399.2009.
- Clausen, S. K., M. Bergqvist, L. K. Poulsen, O. M. Poulsen, and G. D. Nielsen. 2003. “Development of Sensitisation or Tolerance Following Repeated OVA Inhalation in BALB/cJ Mice. Dose-Dependency and Modulation by the Al(OH)3 Adjuvant.” Toxicology 184 (1): 51–68. doi:10.1016/S0300-483X(02)00583-8.
- Conrad, M. L., R. Ferstl, R. Teich, S. Brand, N. Blümer, A. Ö. Yildirim, C. C. Patrascan, et al. 2009. “Maternal TLR Signaling Is Required for Prenatal Asthma Protection by the Nonpathogenic Microbe Acinetobacter lwoffii F78.” The Journal of Experimental Medicine 206 (13): 2869–2877., doi:10.1084/jem.20090845.
- Corson, L., H. Zhu, C. Quan, G. Grunig, M. Ballaney, X. Jin, F. P. Perera, et al. 2010. “Prenatal Allergen and Diesel Exhaust Exposure and Their Effects on Allergy in Adult Offspring Mice.” Allergy, Asthma & Clinical Immunology 6 (1): 7. doi:10.1186/1710-1492-6-7.
- Dietert, R. R., and J. T. Zelikoff. 2008. “Early-Life Environment, Developmental Immunotoxicology, and the Risk of Pediatric Allergic Disease Including Asthma.” Birth Defects Research Part B: Developmental and Reproductive Toxicology 83 (6): 547–560. doi:10.1002/bdrb.20170.
- Dong, J., and Q. Ma. 2018. “Type 2 Immune Mechanisms in Carbon Nanotube-Induced Lung Fibrosis.” Frontiers in Immunology 9: 1120. doi:10.3389/fimmu.2018.01120.
- El-Sayed, Y. S., R. Shimizu, A. Onoda, K. Takeda, and M. Umezawa. 2015. “Carbon Black Nanoparticle Exposure During Middle and Late Fetal Development Induces Immune Activation in Male Offspring Mice.” Toxicology 327: 53–61. doi:10.1016/j.tox.2014.11.005.
- Fedulov, A. V., A. Leme, Z. Yang, M. Dahl, R. Lim, T. J. Mariani, L. Kobzik, et al. 2008. “Pulmonary Exposure to Particles During Pregnancy Causes Increased Neonatal Asthma Susceptibility.” American Journal of Respiratory Cell and Molecular Biology 38 (1): 57–67. doi:10.1165/rcmb.2007-0124OC.
- Finkelman, F. D. 2014. “Diesel Exhaust Particle Exposure During Pregnancy Promotes Development of Asthma and Atopy.” Journal of Allergy and Clinical Immunology 134 (1): 73–74. doi:10.1016/j.jaci.2014.04.002.
- Francis, A. P., and T. Devasena. 2018. “Toxicity of Carbon Nanotubes: A Review.” Toxicology and Industrial Health 34 (3): 200–210. doi:10.1177/0748233717747472.
- Galli, S. J., M. Tsai, and A. M. Piliponsky. 2008. “The Development of Allergic Inflammation.” Nature 454 (7203): 445–454. doi:10.1038/nature07204.
- Hansen, J. S, et al. 2007. “Does Lipophilicity per se Induce Adjuvant Effects? Methyl Palmitate as Model Substance Does Not Affect Ovalbumin Sensitization.” Journal of Toxicology & Environmental Health Part A: Current Issues. 70: 128–137.
- Hansen, J. S., U. C. Nygaard, R. Lyle, and M. Lovik. 2012. “Early Life Interventions to Prevent Allergy in the Offspring: The Role of Maternal Immunization and Postnatal Mucosal Allergen Exposure.” International Archives of Allergy and Immunology 158 (3): 261–275. doi:10.1159/000332963.
- Hansen, J. S., A. W. Nørgaard, I. K. Koponen, J. B. Sørli, M. D. Paidi, S. W. K. Hansen, P. A. Clausen, et al. 2016. “Limonene and Its Ozone-Initiated Reaction Products Attenuate Allergic Lung Inflammation in Mice.” Journal of Immunotoxicology 13 (6): 793–803., doi:10.1080/1547691X.2016.1195462.
- He, J. -S., S. Narayanan, S. Subramaniam, W. Q. Ho, J. J. Lafaille, and M. A. Curotto de Lafaille. 2015. “Biology of IgE Production: IgE Cell Differentiation and the Memory of IgE responses.” Current Topics in Microbiology and Immunology 388: 1–19. doi:10.1007/978-3-319-13725-4_1.
- He, J. -S., S. Subramaniam, V. Narang, K. Srinivasan, S. P. Saunders, D. Carbajo, T. Wen-Shan, et al. 2017. “IgG1 Memory B Cells Keep the Memory of IgE Responses.” Nature Communications 8 (1): 641., doi:10.1038/s41467-017-00723-0.
- Hiraku, Y., F. Guo, N. Ma, T. Yamada, S. Wang, S. Kawanishi, M. Murata, et al. 2016. “Multi-Walled Carbon Nanotube Induces Nitrative DNA Damage in Human Lung Epithelial Cells via HMGB1-RAGE Interaction and Toll-like Receptor 9 Activation.” Particle and Fibre Toxicology 13 (1): 16. doi:10.1186/s12989-016-0127-7.
- Holt, P. G., A. H. Rose, J. E. Batty, and K. J. Turner. 1981. “Inhibition of Specific IgE Responses in Mice by Pre-Exposure to Inhaled Antigen.” International Archives of Allergy and Immunology 65 (1): 42–417. doi:10.1159/000232736.
- Hougaard, K. S., P. Jackson, Z. O. Kyjovska, R. K. Birkedal, P. -J. De Temmerman, A. Brunelli, E. Verleysen, et al. 2013. “Effects of Lung Exposure to Carbon Nanotubes on Female Fertility and Pregnancy. A Study in Mice.” Reproductive Toxicology. 41: 86–97. doi:10.1016/j.reprotox.2013.05.006.
- Jackson, P., K. Kling, K. A. Jensen, P. A. Clausen, A. M. Madsen, H. Wallin, U. Vogel, et al. 2015. “Characterization of Genotoxic Response to 15 Multiwalled Carbon Nanotubes with Variable Physicochemical Properties Including Surface Functionalizations in the FE1-Muta(TM) Mouse Lung Epithelial Cell Line.” Environmental and Molecular Mutagenesis 56 (2): 183–203. doi:10.1002/em.21922.
- Jedrychowski, W., F. P. Perera, U. Maugeri, D. Mrozek-Budzyn, E. Mroz, E. Flak, S. Edwards, et al. 2009. “Early Wheezing Phenotypes and Severity of Respiratory Illness in Very Early Childhood: Study on Intrauterine Exposure to Fine Particle Matter.” Environment International. 35 (6): 877–884. doi:10.1016/j.envint.2009.03.004.
- Jedrychowski, W. A., F. P. Perera, U. Maugeri, E. Mroz, M. Klimaszewska-Rembiasz, E. Flak, S. Edwards, et al. 2010a. “Effect of Prenatal Exposure to Fine Particulate Matter on Ventilatory Lung Function of Preschool Children of Non-Smoking Mothers.” Paediatric and Perinatal Epidemiology 24 (5): 492–501. doi:10.1111/j.1365-3016.2010.01136.x.
- Jedrychowski, W. A., F. P. Perera, U. Maugeri, D. Mrozek-Budzyn, E. Mroz, M. Klimaszewska-Rembiasz, E. Flak, et al. 2010b. “Intrauterine Exposure to Polycyclic Aromatic Hydrocarbons, Fine Particulate Matter and Early Wheeze. Prospective Birth Cohort Study in 4-Year Olds.” Pediatric Allergy and Immunology 21 (4p2): e723–32., doi:10.1111/j.1399-3038.2010.01034.x.
- Jedrychowski, W. A., F. P. Perera, J. D. Spengler, E. Mroz, L. Stigter, E. Flak, R. Majewska, et al. 2013. “Intrauterine Exposure to Fine Particulate Matter as a Risk Factor for Increased Susceptibility to Acute Broncho-Pulmonary Infections in Early Childhood.” International Journal of Hygiene and Environmental Health 216 (4): 395–401. doi:10.1016/j.ijheh.2012.12.014.
- Johansson, H. K. L., J. S. Hansen, B. Elfving, S. P. Lund, Z. O. Kyjovska, S. Loft, K. K. Barfod, et al. 2017. “Airway Exposure to Multi-Walled Carbon Nanotubes Disrupts the Female Reproductive Cycle Without Affecting Pregnancy Outcomes in Mice.” Particle and Fibre Toxicology 14 (1): 17., doi:10.1186/s12989-017-0197-1.
- Kilkenny, C., W. J. Browne, I. C. Cuthill, M. Emerson, and D. G. Altman. 2010. “Improving Bioscience Research Reporting: The ARRIVE Guidelines for Reporting Animal Research.” PLoS Biology 8 (6): e1000412. doi:10.1371/journal.pbio.1000412.
- Kolosnjaj-Tabi, J., J. Just, K. B. Hartman, Y. Laoudi, S. Boudjemaa, D. Alloyeau, H. Szwarc, et al. 2015. “Anthropogenic Carbon Nanotubes Found in the Airways of Parisian Children.” EBioMedicine 2 (11): 1697–1704. doi:10.1016/j.ebiom.2015.10.012.
- Looney, T. J., J. -Y. Lee, K. M. Roskin, R. A. Hoh, J. King, J. Glanville, Y. Liu, et al. 2016. “Human B-Cell Isotype Switching Origins of IgE.” Journal of Allergy and Clinical Immunology 137 (2): 579–586.e7. doi:10.1016/j.jaci.2015.07.014.
- Lowrey, J. A., N. D. L. Savage, D. Palliser, M. Corsin-Jimenez, L. M. G. Forsyth, G. Hall, S. Lindey, et al. 1998. “Induction of Tolerance via the Respiratory Mucosa.” International Archives of Allergy and Immunology 116 (2): 93–102., doi:10.1159/000023931.
- Mackenzie, N. M. 1990. “Transport of Maternally Derived Immunoglobulins Across the Intestinal Epithelium.” In Ontogeny of the Immune System of the Gut, edited by T. T. MacDonald, 69–82. 1st ed. Boca Raton, FL: CRC Press.
- Manners, S., R. Alam, D. A. Schwartz, and M. M. Gorska. 2014. “A Mouse Model Links Asthma Susceptibility to Prenatal Exposure to Diesel Exhaust.” Journal of Allergy and Clinical Immunology 134 (1): 63–72. doi:10.1016/j.jaci.2013.10.047.
- Mitchell, L. A., J. Gao, R. V. Wal, A. Gigliotti, S. W. Burchiel, and J. D. McDonald. 2007. “Pulmonary and Systemic Immune Response to Inhaled Multiwalled Carbon Nanotubes.” Toxicological Sciences 100 (1): 203–214. doi:10.1093/toxsci/kfm196.
- Mitchell, L. A., F. T. Lauer, S. W. Burchiel, and J. D. McDonald. 2009. “Mechanisms for How Inhaled Multiwalled Carbon Nanotubes Suppress Systemic Immune Function in Mice.” Nature Nanotechnology 4 (7): 451–456. doi:10.1038/nnano.2009.151.
- Mukherjee, S. P., O. Bondarenko, P. Kohonen, F. T. Andón, T. Brzicová, I. Gessner, S. Mathur, et al. 2018. “Macrophage Sensing of Single-Walled Carbon Nanotubes via Toll-like Receptors.” Scientific Reports 8 (1): 1115. doi:10.1038/s41598-018-19521-9.
- Nygaard, U. C., J. S. Hansen, M. Samuelsen, T. Alberg, C. D. Marioara, and M. Løvik. 2009. “Single-Walled and Multi-Walled Carbon Nanotubes Promote Allergic Immune Responses in Mice.” Toxicological Sciences 109 (1): 113–123. doi:10.1093/toxsci/kfp057.
- Pandya, R. J., G. Solomon, A. Kinner, and J. R. Balmes. 2002. “Diesel Exhaust and Asthma: Hypotheses and Molecular Mechanisms of Action.” Environmental Health Perspectives. 110 (Suppl 1): 103–112. doi:10.1289/ehp.02110s1103.
- Parnia, S., and A. J. Frew. 2001. “Is Diesel the Cause for the Increase in Allergic Disease?” Ann Allerg Asthma Immunol 87 (6): 18–23. doi:10.1016/S1081-1206(10)62335-6.
- Pawankar R., S. T. Holgate, G. Walter Canonica, R. F. Lockey, M. S. Blaiss, eds. 2013. WAO White Book on Allergy: Update 2013. Milwaukee, WI: World Allergy Organization.
- Poulsen, S. S., A. T. Saber, A. Mortensen, J. Szarek, D. Wu, A. Williams, O. Andersen, et al. 2015a. “Changes in Cholesterol Homeostasis and Acute Phase Response Link Pulmonary Exposure to Multi-Walled Carbon Nanotubes to Risk of Cardiovascular Disease.” Toxicology and Applied Pharmacology. 283 (3): 210–222. doi:10.1016/j.taap.2015.01.011.
- Poulsen, S. S., A. T. Saber, A. Williams, O. Andersen, C. Købler, R. Atluri, M. E. Pozzebon, et al. 2015b. “MWCNTs of Different Physicochemical Properties Cause Similar Inflammatory Responses, but Differences in Transcriptional and Histological Markers of Fibrosis in Mouse Lungs.” Toxicology and Applied Pharmacology. 284 (1): 16–32.
- Rajasekaran, S., R. Anuradha, and R. Bethunaickan. 2017. “TLR Specific Immune Responses Against Helminth Infections.” Journal of Parasitology Research. 2017: 6865789. doi:10.1155/2017/6865789.
- Rychlik, K. A., J. R. Secrest, C. Lau, J. Pulczinski, M. L. Zamora, J. Leal, R. Langley, et al. 2019. “In Utero Ultrafine Particulate Matter Exposure Causes Offspring Pulmonary Immunosuppression.” Proceedings of the National Academy of Sciences 116 (9): 3443–3448. doi:10.1073/pnas.1816103116.
- Saber, A. T., S. Halappanavar, J. K. Folkmann, J. Bornholdt, A. Boisen, P. Møller, A. Williams, et al. 2009. “Lack of Acute Phase Response in the Livers of Mice Exposed to Diesel Exhaust Particles or Carbon Black by Inhalation.” Particle and Fibre Toxicology 6 (1): 12., doi:10.1186/1743-8977-6-12.
- Saber, A., N. Jacobsen, A. Mortensen, J. Szarek, P. Jackson, A. Madsen, K. Jensen, et al. 2012. “Nanotitanium Dioxide Toxicity in Mouse Lung Is Reduced in Sanding Dust from Paint.” Particle and Fibre Toxicology 9 (1): 4. doi:10.1186/1743-8977-9-4.
- Shimizu, R., M. Umezawa, S. Okamoto, A. Onoda, M. Uchiyama, K. Tachibana, S. Watanabe, et al. 2014. “Effect of Maternal Exposure to Carbon Black Nanoparticle During Early Gestation on the Splenic Phenotype of Neonatal Mouse.” The Journal of Toxicological Sciences 39 (4): 571–578. doi:10.2131/jts.39.571.
- Smole, U., I. Schabussova, W. F. Pickl, and U. Wiedermann. 2017. “Murine Models for Mucosal Tolerance in Allergy.” Seminars in Immunology. 30: 12–27. doi:10.1016/j.smim.2017.07.007.
- Sudowe, S.,. A. Rademaekers, and E. Kolsch. 1997. “Antigen Dose-Dependent Predominance of Either Direct or Sequential Switch in IgE Antibody Responses.” Immunology 91 (3): 464–472. doi:10.1046/j.1365-2567.1997.00268.x.
- Turabekova, M., B. Rasulev, M. Theodore, J. Jackman, D. Leszczynska, and J. Leszczynski. 2014. “Immunotoxicity of Nanoparticles: A Computational Study Suggests That CNTs and C60 Fullerenes Might Be Recognized as Pathogens by Toll-like Receptors.” Nanoscale 6 (7): 3488–3495. doi:10.1039/C3NR05772K.
- Van Loveren, H., and A. Piersma. 2004. “Immunotoxicological Consequences of Perinatal Chemical Exposures.” Toxicology Letters. 149 (1-3): 141–145. doi:10.1016/j.toxlet.2003.12.028.
- Vlaanderen, J., A. Pronk, N. Rothman, A. Hildesheim, D. Silverman, H. D. Hosgood, S. Spaan, et al. 2017. “A Cross-Sectional Study of Changes in Markers of Immunological Effects and Lung Health Due to Exposure to Multi-Walled Carbon Nanotubes.” Nanotoxicology 11 (3): 395–404., doi:10.1080/17435390.2017.1308031.
- Wang, L., and K. E. Pinkerton. 2008. “Detrimental Effects of Tobacco Smoke Exposure During Development on Postnatal Lung Function and Asthma.” Birth Defects Research Part C: Embryo Today: Reviews 84 (1): 54–60. doi:10.1002/bdrc.20114.
- Weichenthal, S., A. Dufresne, and C. Infante-Rivard. 2007. “Indoor Ultrafine Particles and Childhood Asthma: Exploring a Potential Public Health Concern.” Indoor Air 17 (2): 81–91. doi:10.1111/j.1600-0668.2006.00446.x.
- Whitten, W. K. 1959. “Occurrence of Anoestrus in Mice Caged in Groups.” Journal of Endocrinology 18 (1): 102–107. doi:10.1677/joe.0.0180102.
- Xiong, H., J. Dolpady, M. Wabl, M. A. Curotto de Lafaille, and J. J. Lafaille. 2012. “Sequential Class Switching Is Required for the Generation of High Affinity IgE Antibodies.” The Journal of Experimental Medicine 209 (2): 353–364. doi:10.1084/jem.20111941.
- Zhang, M. F., H. Zola, L. C. Read, and I. A. Penttila. 1999. “Localization of Transforming Growth Factor-Beta Receptor Types I, II, and III in the Postnatal Rat Small Intestine.” Pediatric Research 46 (6): 657–665. doi:10.1203/00006450-199912000-00017.